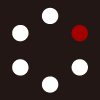
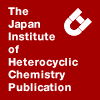
HETEROCYCLES
An International Journal for Reviews and Communications in Heterocyclic ChemistryWeb Edition ISSN: 1881-0942
Published online by The Japan Institute of Heterocyclic Chemistry
e-Journal
Full Text HTML
Received, 3rd January, 2012, Accepted, 1st March, 2012, Published online, 12th March, 2012.
DOI: 10.3987/REV-12-726
■ Synthesis of Nitrogen-Containing Heterocycles Using Conjugate Addition Reactions of Nucleophiles to α,β-Unsaturated Imines
Iwao Hachiya, Isao Mizota, and Makoto Shimizu*
Department of Chemistry for Materials, Graduate School of Engineering, Mie University, 1577 Tsu, Mie 514-8507, Japan
Abstract
Synthetic methods for the synthesis of nitrogen-containing heterocycles are of utmost interest and importance because these structures are the key components of natural and unnatural biologically active compounds and functionalized materials. This review summarizes the synthesis of nitrogen-containing heterocycles, where the conjugate addition of nucleophiles to α,β-unsaturated imines is the crucial step.CONTENTS
1. Introduction
2. Synthesis of nitrogen-containing heterocycles via 1,4- and 1,2-double nucleophilic addition reactions
2-1. Synthesis of 2,3,5-trisubstituted pyrroles
2-2. Synthesis of δ-lactams
3. Synthesis of nitrogen-containing heterocycles via conjugate addition of nucleophiles to alkynyl imines
3-1. Synthesis of multi-substituted 2-pyridones
3-2. Synthesis of multi-substituted bicyclo-2-pyridones
3-3. Synthesis of multi-substituted 2-iminopyridines and 2-aminopyridines
3-4. Stereodivergent synthesis of both cis- and trans-β-lactams
4. Conclusion
5. Acknowledgements
6. References
1. INTRODUCTION
The development of new, efficient synthetic methods for the synthesis of nitrogen-containing heterocycles is extensively studied, because many nitrogen-containing heterocycles, such as nucleic acid bases, vitamins, amino acids, alkaloids, and β-lactams, exhibit biological activities and are useful as functionalized materials for organic electronics or photonics. Imines, one of the most useful types of nitrogen-containing compounds, are typically prepared by the condensation of carbonyl compounds with primary amines via dehydration. This review describes new synthetic methods for the synthesis of nitrogen-containing heterocycles via the conjugate addition reactions of nucleophiles to α,β-unsaturated imines.
2. SYNTHESIS OF NITROGEN-CONTAINING HETEROCYCLES VIA 1,4- AND 1,2-DOUBLE NUCLEOPHILIC ADDITION REACTIONS
2-1. Synthesis of 2,3,5-trisubstituted pyrroles
Pyrrole derivatives have widespread biological importance,1 and therefore, it is increasingly important to construct such skeletons in an efficient manner.2 A potentially effective approach for the construction of the pyrrole ring involves cyclization of γ-amino carbonyl compounds followed by dehydrogenation. For the synthesis of γ-amino carbonyl compounds, crucial intermediates in this strategy, the nucleophilic addition to γ-oxoimines constitutes a straightforward pathway. However, difficulty has been encountered frequently in the synthesis of γ-oxoimines due to their susceptibility to hydrolysis and/or isomerization.3 For the circumvention of such a drawback of isolating these relatively unstable imine intermediates as well as to use an operationally simple experimental procedure, we introduced a double nucleophilic addition reaction to α,β-unsaturated imines 1.4 When α,α-dialkoxy ketene silyl acetal 2, an acyl anion equivalent, is used as the first nucleophile, this methodology offers a facile synthetic route to γ-amino carbonyl synthons 4, which on treatment with sulfuric acid followed by oxidation with DDQ gave 2,3,5-trisubstituted pyrroles 5 in good yields.5
The following examples show the double nucleophilic addition (Scheme 2), cyclization (Scheme 3), and dehydration (Scheme 4).
To synthesize γ-amino carbonyl compounds, this reaction was carried out using imine 6 and ketene silyl acetal 2 in CH2Cl2 in the presence of aluminum chloride and MS 4 Å containing H2O from −78°C to room temperature to give the double addition product 7 in good yield with syn-selectivity. In addition, the use of ketene silyl thioacetal 8 or trimethylsilyl cyanide (9) as the second nucleophile with ketene silyl acetal 2 as the first nucleophile gave the double addition products 7 in good yields. The ketene silyl acetal 2 underwent 1,4-addition, while the ketene silyl thioacetal 8 or trimethylsilyl cyanide (9) underwent 1,2-addition in a highly regioselective manner. All of the 1,4- and 1,2-addition products 7 were readily converted into the corresponding multi-substituted pyrroles 11 via cyclization into the dihydropyrrole 10 with H2SO4 followed by dehydrogenation with DDQ. Both steps proceeded well to give these products in good to high yields.
This transformation into 2,3,5-trisubstituted pyrroles enabled a six-step synthesis of imidazole glycerol phosphate dehydratase inhibitors (IGPDIs, which exhibit herbicidal activity.6 The synthesis of the IGPDI 12 was carried out in the following manner (Scheme 5). The ketene silyl acetal 2 and trimethylsilyl cyanide (9) were treated with N-4-methoxycrotylideneamine 13 in the presence of AlCl3 to give the doubly alkylated product 14 in 95% yield. Switching of the PMP group for a Boc analogue was carried out before cyclization into the pyrrole. The Boc-protected double-addition product 15 was treated with MeSO3H and DDQ to afford the pyrrole 17 in 92% yield (2 steps), where the NH-free dihydropyrrole intermediate 16 was not isolated. Finally, reduction of the cyano group with Raney-Ni W4 in the presence of NaPH2O2 gave the desired monopyrrole aldehyde 12 in 73% yield.
2-2. Synthesis of δ-Lactams
δ-Lactams are frequently observed in natural products, such as strychnine, matrine, and surugatoxin, and their derivatives are useful for the preparation of drug-like molecules. They are important synthetic building blocks for the development of medicinally active compounds, and many of them are of great interest because of their unique biological activities.7 As mentioned above, weintroduced a double nucleophilic addition reaction to α,β-unsaturated imines using several nucleophiles.4,5,8 For the reaction with N-allylideneamine 18, when the trisubstituted ketene silyl thioacetal 19 derived from S-cyclohexyl propanethioate was used in the presence of dried silica gel, the cyclized δ-lactam 20 was obtained in high yield by work-up with trifluoroacetic acid (TFA) (Table 1, entry 1).9 The trisubstituted ketene silyl thioacetals 19 gave the corresponding δ-lactams 20 in moderate to good yields (entries 1–6). The use of a tetrasubstituted analogue also gave the product 20 in moderate yield (entry 7).
A proposed reaction mechanism is shown in Scheme 6. First, the N-allylideneamine 18 is activated with silica gel followed by 1,4-addition of ketene silyl thioacetal 19 to give an intermediary enamine species 21. Isomerization to iminium species 22 is then promoted by silica gel followed by the 1,2-addition of TMSCN 9 to give the 1,4- and 1,2-products 23. Deprotection of the trityl group with TFA gives amino nitrile 24, which cyclizes into δ-lactams 20.
3. SYNTHESIS OF NITROGEN-CONTAINING HETEROCYCLES VIA CONJUGATE ADDITION OF NUCLEOPHILES TO ALKYNYL IMINES
3-1. Synthesis of Multi-substituted 2-Pyridones
Alkynyl imines are one of the most important nitrogen-containing starting materials because of their extensive use in the synthesis of heterocycles including nitrogen atoms, such as pyrazoles,10 pyrimidines,10 pyrrolinones,11 pyrroles,2g indolizines,2g quinolines,12 fused pyrrolines,12 and pyridines.13 In addition, alkynyl imines are used in [2+2]-cycloaddition reactions with ketenes or the reaction with several enolates to give β-lactams.14 The reactivity at the β-position of alkynyl imines is of particular interest. For example, the reaction of the sodium salt of dimethyl alkylmalonate 25 with alkynyl imine 26 proceeds in moderate to high yields to give the 2-pyridone 27 bearing a 5-methoxycarbonyl group.15 The development of synthetic methods for the preparation of functionalized 2-pyridone is important because a large number of biologically active compounds contain the 2-pyridone structure.16 Representative examples of this 2-pyridone synthesis are summarized in Table 2.
Both aromatic and aliphatic imines can be used to produce the 2-pyridones 27 in moderate to good yields. Even when the steric bulk of the nucleophile was increased, as with dimethyl allylmalonate 25 (R1 = allyl), the 2-pyridones 27 were obtained in moderate yields (entries 5–7). Although numerous methods for the synthesis of 2-pyridones have been reported,17 the present 2-pyridone synthesis is an attractive alternative because alkynyl imines and substituted malonic esters are readily available.
A possible reaction mechanism is shown in Scheme 7. The metalloallenamine 28 would be generated via a 1,4-addition reaction of the sodium salt of dimethyl methylmalonate 25 to alkynyl imine 26 and undergo an intramolecular cyclization to give cyclobutenoxide 29. The cyclobutenoxide 29 would collapse into the metalloenamine 30 via a ring-opening reaction to release the ring strain of the cyclobutene, followed by subsequent cyclization to give the 2-pyridone 27.
When β-keto esters 31 were used as the nucleophile instead of 2-substituted malonic esters 25, the reactions with imines 26 gave 5-acetyl-2-pyridones 32 in moderate yields (Table 3).18
A possible reaction mechanism is shown in Scheme 8. The metalloallenamine 33 generated via the 1,4-addition reaction of the sodium salt of β-keto ester 31 to alkynyl imine 26 undergoes an intramolecular cyclization at the more reactive keto carbonyl group to give the cyclobutenoxide 34. The cyclobutenoxide 34 is then transformed into the metalloenamine 35 via ring-opening, and subsequent cyclization gives the 5-acetyl-2-pyridone 32.
The synthesis of 3,4,5,6-tetrasubstituted 2-pyridone was carried out via the nucleophilic addition of active methine compounds to dialkynyl imines with the goal of achieving the synthesis of (-)-A58365A. This compound was obtained in the Eli Lilly laboratories from a fermentation broth of the bacterium Streptomyces chromofucus and found to be an angiotensin-converting enzyme inhibitor at nanomolar concentrations.19,20 The reaction of active methine compounds 36, such as malonic esters or β-keto esters, to dialkynyl imines 37 proceeded to give 3,4,5,6-tetrasubstituted-2-pyridones 38 in moderate to good yields (Table 4).21 The reaction of unsymmetrical dialkynyl imine 37 proceeded regioselectively to give only 2-pyridone 38, where the less hindered sp carbon reacted preferentially (entries 5–10).
3-2. Synthesis of Multi-substituted Bicyclo-2-pyridones22
Bicyclic compounds containing a 2-pyridone structure are key intermediates for the total synthesis of anagyrine,23 lupinine,23a and ipalbidine.24 In addition, there are biologically active compounds possessing a 2-pyridone structure, such as (-)-A58365A.19,20 2-Alkynylpyridine 39 (Z = CH) and 2-alkynylpyrimidine 39 (R = N) were used as a cyclic alkynyl imine equivalent. The reaction of 2-alkynylpyridines 39 (Z = CH) or 2-phenylethynylpyrimidine 39 (Z = N) with several malonic esters 25 proceeded to give 4H-quinolizin-4-ones 40 (Z = CH) or 6H-pyrido[1,2-a]pyrimidin-6-ones 40 (Z = N), respectively, in moderate to good yields (Table 5).
The use of 2-alkynylthiazoles 41 as a cyclic alkynyl imine equivalent gave 5H-thiazolo[3,2-a]pyridin- 5-ones 42 in good to high yields (Table 6).
When cyclic β-keto ester 43 was used in the conjugate addition reactions with alkynyl imine 44 instead of the acyclic derivatives, the ring expansion reactions proceeded via cyclobutenoxide as a Grob-type fragmentation intermediate to give the two-atom enlarged carbocyclic product 45 possessing a masked formyl group.25 During the investigation of the ring expansion reaction, when the ethyl 2-oxocyclododecanecarboxylate (46), a more flexible molecule, was used as the β-keto ester, the reaction with alkynyl imine 44 gave the bicylo-2-pyridone 47 in 53% yield (Scheme 9).
A plausible reaction mechanism is shown in Scheme 10. Metalloallenamine 48 would be generated via a conjugate addition of the sodium salt of β-keto esters 43 or 46 to alkynyl imine 44, and undergo a chemoselective intramolecular cyclization at the keto carbonyl group to give the cyclobutenoxide intermediate 49. The cyclobutenoxide 49 (n = 4) would collapse into the metalloenamine 50 via a ring-opening reaction to release the ring strain in the cyclobutene, and subsequent protonation with water to quench the reaction would give the ring expansion product 45. On the other hand, in the reaction of ethyl 2-oxocyclododecanecarboxylate (46), (E)-metalloenamine 51 generated via the ring-opening reaction would isomerize into (Z)-metalloenamine 52 because of the flexibility of the large carbocycle, and subsequent cyclization would give bicylo-2-pyridone 47.
3-3. Synthesis of Multi-substituted 2-Iminopyridines and 2-Aminopyridines26
iologically active compounds containing a 2-iminopyridine structure are less known than those containing the 2-pyridone counterpart;27 however, 2-iminopyridines are highly attractive compounds compared with members of the large group of biologically active 2-pyridones.16,28 Several methods for the synthesis of 2-iminopyridines via the condensation of cyano derivatives29 and other reactions30 using transition metals have been reported. However, the former methods are not yet satisfactory from the viewpoint of synthesizing 2-iminopyridines possessing the desired substituents. Therefore, the development of alternative synthetic methods is requied for the preparation of multi-substituted 2-iminopyridines from readily available starting materials. On the basis of our 2-pyridone synthesis, the reaction of alkynyl imines 53 was carried out with ethyl cyanoacetate derivatives 54. The reaction of alkynyl imines 53 bearing p-methoxyphenylmethyl (MPM) and p-methoxyphenyl (PMP) groups at the nitrogen proceeded with ethyl cyanoacetate derivatives 54 to give the corresponding 2-iminopyridines 55 (R3 = MPM or PMP) in moderate to good yields (Table 7).
A plausible reaction mechanism is shown in Scheme 11. Metalloenamine 56 would be generated via a conjugate addition of the potassium salt of the cyanoacetate derivative 54 to the alkynyl imine 53 and undergo a chemoselective intramolecular cyclization at the ethoxycarbonyl group to give the cyclobutenoxide intermediate 57. The cyclobutenoxide 57 would collapse into the metalloenamine 58 via a ring-opening reaction to release the ring strain of the cyclobutene, and subsequent cyclization would provide the 2-iminopyridine 55 after protonation of the 2-iminopyridine potassium salt 59 with water to quench the reaction.
Alkynylthiazole 60 was used instead of alkynyl imines 53 to synthesize a bicyclic compound containing a 2-iminopyridine structure. The reaction of 60 with ethyl 2-cyanopropanoate (61) gave the bicyclo-2- iminopyridine 62 in 64% yield. In addition, the synthesis of a 3,4,5,6-tetrasubstituted 2-iminopyridine was carried out. The reaction of dialkynyl imine 63 with 61 gave 2-iminopyridine 64 in 52% yield (Scheme 12).
The transformation of 2-iminopyridine into 2-aminopyridine was also achieved via the deprotection of substituent at the nitrogen.31 2-Aminopyridines are one of the most important heterocycles because of their biological activity.32 Deprotection of the p-methoxyphenyl group of 2-iminopyridine 55 (R3 = PMP) was attempted under several reaction conditions. However, the desired 2-aminopyridine 65 was not obtained. Trifluoromethanesulfonic acid (TfOH) was found to promote the deprotection of 2-iminopyridine 55 (R3 = MPM) in trifluoroacetic acid (TFA) under reflux, to give the desired 2-aminopyridine 65 in moderate to good yields (Scheme 13).
3-4. Stereodivergent Synthesis of Both cis- and trans-β-Lactams33
New synthetic routes to β-lactams are of great importance for structure-activity relationship studies and the development of new derivatives of β-lactam antibiotics. β-Lactams are also used as versatile building blocks.34 It has been recognized that the stereochemistry at the C-3 and C-4 carbons of the β-lactam ring is involved in the manifestations of biological activity.35 The Staudinger reaction, enolate-imine condensations, and the cyclization of β-amino acids or esters have been used for stereocontrolled construction of the C-3 and C-4 carbons of β-lactams.36 Although numerous methods for the stereoselective synthesis of cis- and trans-β-lactams have been reported, a single-step synthesis has been highly desired from the same precursor. During our investigations involving alkynyl imines, it was envisioned that iminocyclobutenones would be useful intermediates for the synthesis of nitrogen-containing heterocycles via thermal rearrangement of the cyclobutenone ring.37
The conjugate addition of alkynyl imines 66 with ketene silyl acetals 67 in the presence of aluminum chloride proceeded to give iminocyclobutenones 68 (Table 8). Alkynyl imines possessing heteroaromatic or aromatic groups as the R2 substituent underwent conjugate addition reactions to give the corresponding iminocyclobutenones 68 in good to high yields (entries 1–4). The reaction of alkynyl imines 66 with the ketene silyl acetal 67 possessing an ethanesulfenyl group gave iminocyclobutenones 68 in good yields (entries 6 and 7).
We carried out the chemoselective reduction of the iminocyclobutenones 68 possessing three reducible functional groups, such as imino, carbonyl, and alkenyl substituents. When sodium cyanoborohydride was used as a reducing reagent, the reduction of iminocyclobutenones 68 in methanol in the presence of acetyl chloride, which reacted with methanol to generate in situ a limited amount of hydrogen chloride, proceeded chemoselectively at room temperature to give the desired aminocyclobutenones 69 in moderate to high yields (Table 8).
Thermal rearrangement of the aminocyclobutenones 69 in toluene or octane at 110 °C proceeded to give the desired β-lactams 70 in moderate to good yields, and with good cis-selectivities (Table 9).
A plausible reaction mechanism for the thermal rearrangement of the aminocyclobutenones 69 into the β-lactams 70 is shown in Scheme 14. Aminoketene 71 would be generated by the ring opening of aminocyclobutenone 69 and undergo cyclization to give the enol intermediate 72. Protonation of the enol intermediate 72 would occur predominantly from the less hindered face of the enol, to give the cis-β-lactam 70.
The protonation step is crucial in determining the cis-selectivity. Therefore, several additives were investigated in the thermal rearrangement of aminocyclobutenones 73. Among the additives tested, 1,4-dimethylpiperazine was found to be the most effective regarding both the cis-selectivity and yield (Table 10, entries 1–5). On the other hand, thermal rearrangement in the presence of a stronger base, DBU, gave the β-lactam 74 with trans-selectivity (Table 10, entries 6–10).
4. CONCLUSION
The 1,4- and 1,2-double nucleophilic addition studied here provides a new entry into reactions using α,β-unsaturated aldimine as a good acceptor of two types of nucleophiles in one pot. In particular, the use of an α,α-dialkoxy ketene silyl acetal, an acyl anion equivalent as the first nucleophile, provides a facile approach to 2,3,5-trisubstituted pyrroles in a regioselective manner. The synthetic utility of this methodology is realized in the construction of various types of pyrroles, in particular the IGPDI. For the reaction with N-allylideneamine, the use of a ketene silyl thioacetal as the first nucleophile in the presence of dried silica gel provides the cyclized δ-lactam in high yield.
In addition, we found an intriguing heterocycle synthesis based on the nucleophilic addition of active methine compounds to alkynyl imines. Varying the active methine compounds leads to a specific approach to otherwise non-trivial heterocycles. When a transannular migration of the carbonyl group is involved, this methodology offers a Grob-type fragmentation reaction leading to ring-expansion products involving bicyclo-2-pyridones in a chemoselective manner. The stereodivergent synthesis of both cis- and trans-β-lactams was also discovered using the following three crucial reactions: the iminocyclobutenone formation, chemoselective reduction of the imino groups, and thermal rearrangement of the aminocyclobutenones. In this route, the key synthetic intermediates: the iminocyclobutenones were readily synthesized in good yields via the conjugate addition of alkynyl imines with ketene silyl acetals. These new reactions provide new and easy access to nitrogen-containing molecules that are important synthetic intermediates for many bioactive compounds.
5. ACKNOWLEDGEMENTS
We would like to thank our coworkers for their intellectual and experimental contributions to the present project. This work was supported by Grants-in-Aid for Scientific Research from the Ministry of Education, Science, Sports, and Culture, of the Japanese Government, and grants from the Japan Science and Technology Agency are also gratefully acknowledged.
References
1. (a) H. Annoura and T. Tatsuoka, Tetrahedron Lett., 1995, 36, 413; CrossRef (b) A. Fürstner and H. Weintritt, J. Am. Chem. Soc., 1998, 120, 2817; CrossRef (c) D. L. Boger, C. W. Boyce, M. A. Labroli, C. A. Sehon, and Q. Jin, J. Am. Chem. Soc., 1999, 121, 54; CrossRef (d) D. Stien, G. T. Anderson, C. E. Chase, Y.-h. Koh, and S. M. Weinreb, J. Am. Chem. Soc., 1999, 121, 9574; CrossRef (e) J.-H. Liu, Q.-C. Yang, T. C. W. Mak, and H. N. C. Wong, J. Org. Chem., 2000, 65, 3587; CrossRef (f) M. Adamczyk, D. D. Johnson, and R. E. Reddy, J. Org. Chem., 2001, 66, 11; CrossRef (g) A. T. Kreipl, C. Reid, and W. Steglich, Org. Lett., 2002, 4, 3287; CrossRef (h) J. T. Kim and V. Gevorgyan, Org. Lett., 2002, 4, 4697; CrossRef (i) H. Hoffmann and T. Lindel, Synthesis, 2003, 1753; CrossRef (j) A. Fürstner, Angew. Chem. Int. Ed., 2003, 42, 3582. CrossRef
2. (a) Y. Gao, M. Shirai, and F. Sato, Tetrahedron Lett., 1996, 37, 7787; CrossRef (b) S. Jolivet-Fouchet, J. Hamelin, F. Texier-Boullet, L. Toupet, and P. Jacquault, Tetrahedron, 1998, 54, 4561; CrossRef (c) H. Tsutsui and K. Narasaka, Chem. Lett., 1999, 45; CrossRef (d) T. L. Gilchrist, J. Chem. Soc., Perkin Trans. 1, 1999, 2849; CrossRef (e) H. Shiraishi, T. Nishitani, T. Nishihara, S. Sakaguchi, and Y. Ishii, Tetrahedron, 1999, 55, 13957; CrossRef (f) M. Periasamy, G. Srinivas, and P. Bharathi, J. Org. Chem., 1999, 64, 4204; CrossRef (g) A. V. Kel’in, A.W. Sromek, and V. Gevorgyan, J. Am. Chem. Soc., 2001, 123, 2074; CrossRef (h) B. Quiclet-Sire, F. Wendeborn, and S. Z. Zard, Chem. Commun., 2002, 2214; CrossRef (i) B. C. Ranu and S. S. Dey, Tetrahedron Lett., 2003, 44, 2865; CrossRef (j) B. Ramanathan, A. J. Keith, D. Armstrong, and A. L. Odom, Org. Lett., 2004, 6, 2957; CrossRef (k) A. R. Bharadwaj and K. A. Scheidt, Org. Lett., 2004, 6, 2465. CrossRef
3. It has been reported that several imines are readily hydrolyzed to the parent carbonyl compounds. See, (a) V. Saggiomo and U. Lüning, Tetrahedron Lett., 2009, 50, 4663; CrossRef (b) C. Godoy-Alcántar, A. K. Yatsimirsky, and J.-M. Lehn, J. Phys. Org. Chem., 2005, 18, 979; CrossRef (c) M. Onaka, R. Ohno, N, Yanagiya, and Y. Izumi, Synlett, 1993, 141; CrossRef (d) A. C. Dash, B. Dash, and D. Panda, J. Org. Chem., 1985, 50, 2905. CrossRef
4. M. Shimizu, I. Hachiya, and I. Mizota, Chem. Commun., 2009, 874, and references cited therein. CrossRef
5. (a) M. Shimizu, A. Takahashi, and S. Kawai, Org. Lett., 2006, 8, 3585; CrossRef (b) A. Takahashi, S. Kawai, I. Hachiya, and M. Shimizu, Eur. J. Org. Chem., 2010, 191. CrossRef
6. (a) I. Mori, G. Iwasaki, Y. Kimura, S. Matsunaga, A. Ogawa, T. Nakano, H.-P. Buser, M. Hatano, S. Tada, and K. Hayakawa, J. Am. Chem. Soc., 1995, 117, 4411; CrossRef (b) S. D. Lindell, C. G. Ernshaw, B. J. Wright, D. S. Carver, M. J. O’Mahony, and E. A. Savillo-Stones, Bioorg. Med. Chem. Lett., 1996, 6, 547. CrossRef
7. (a) W. A. Maio, S. Sinishtaj, and G. H. Posner, Org. Lett., 2007, 9, 2673; CrossRef (b) P. J. M. Taylor, S. D. Bull, and P. C. Andrews, Synlett, 2006, 1347; CrossRef (c) J. F. Bower, J. Svenda, A. J. Williams, J. P. H. Charmant, R. M. Lawrence, P. Szeto, and T. Gallagher, Org. Lett., 2004, 6, 4727; CrossRef (d) M. Lautens, E. Tayama, and D. Nguyen, Org. Lett., 2004, 6, 345; CrossRef (e) M. Cellier, Y. Gelas-Mialhe, H.-P. Husson, B. Perrin, and R. Remuson, Tetrahedron: Asymmetry, 2000, 11, 3913; CrossRef (f) S. Kobayashi, R. Akiyama, and M. Moriwaki, Tetrahedron Lett., 1997, 38, 4819; CrossRef (g) J. Ezquerra, C. Pedregal, A. Escribano, M. C. Carreño, and J. L. García Ruano, Tetrahedron Lett., 1995, 36, 3247; CrossRef (h) K. Paulvannan and J. R. Stille, Tetrahedron Lett., 1993, 34, 8197; CrossRef (i) P. D. Bailey, R. D. Wilson, and G. R. Brown, Tetrahedron Lett., 1989, 30, 6781; CrossRef (j) S. R. Angle and D. O. Arnaiz, Tetrahedron Lett., 1989, 30, 515. CrossRef
8. (a) I. Mizota, S. Agatani, I. Hachiya, and M. Shimizu, Tetrahedron Lett., 2011, 52, 5388; CrossRef (b) M. Shimizu, M. Kawanishi, A. Itoh, I. Mizota, and I. Hachiya, Chem. Lett., 2011, 40, 862; CrossRef (c) M. Shimizu, M. Kawanishi, I. Mizota, and I. Hachiya, Org. Lett., 2010, 12, 3571. CrossRef
9. (a) I. Mizota, Y. Matsuda, I. Hachiya, and M. Shimizu, Eur. J. Org. Chem., 2009, 4073; CrossRef (b) I. Mizota, Y. Matsuda, I. Hachiya, and M. Shimizu, Org. Lett., 2008, 10, 3977. CrossRef
10. H.-B. Yu and W.-Y. Huang, J. Fluorine Chem., 1998, 87, 69. CrossRef
11. B. G. Van den Hoven and H. Alper, J. Am. Chem. Soc., 2001, 123, 1017. CrossRef
12. K. Sangu, K. Fuchibe, and T. Akiyama, Org. Lett., 2004, 6, 353. CrossRef
13. M. Movassaghi and M. D. Hill, J. Am. Chem. Soc., 2006, 128, 4592. CrossRef
14. M. D. Stadnichuk, A. V. Khramchikhin, Y. L. Piterskaya, and I. V. Suvorova, Russ. J. Gen. Chem., 1999, 69, 593.
15. I. Hachiya, K. Ogura, and M. Shimizu, Org. Lett., 2002, 4, 2755. CrossRef
16. For examples, see: (a) A. Z. Schultz, Chem. Rev., 1973, 73, 385; CrossRef (b) D. P. Curran and H. Liu, J. Am. Chem. Soc., 1992, 114, 5863; CrossRef (c) A. P. Kozikowski, G. Campiani, L.-Q. Sun, S. Wang, A. Saxena, and B. P. Doctor, J. Am. Chem. Soc., 1996, 118, 11357; CrossRef (d) D. R. Williams, P. D. Lowder, and Y.-G. Gu, Tetrahedron Lett., 1997, 38, 327; CrossRef (e) M.-L. Bennasar, E. Zulaica, C. Juan, Y. Alonso, and J. Bosch, J. Org. Chem., 2002, 67, 7465; CrossRef (f) G. Semple, B.-M. Andersson, V. Chhajlani, J. Georgsson, M. J. Johansson, A. Rosenquist, and L. Swanson, Bioorg. Med. Chem. Lett., 2003, 13, 1141; CrossRef (g) J. J. Parlow and M. S. South, Tetrahedron, 2003, 59, 7695; CrossRef (h) J. J. Parlow, R. G. Kurumbail, R. A. Stegeman, A. M. Stevens, W. C. Stallings, and M. S. South, J. Med. Chem., 2003, 46, 4696; CrossRef (i) P. Fossa, G. Menozzi, P. Dorigo, M. Floreani, and L. Mosti, Bioorg. Med. Chem., 2003, 11, 4749. CrossRef
17. (a) Y. Su, M. Zhao, K. Han, G. Song, and X. Li, Org. Lett., 2010, 12, 5462; CrossRef (b) L. Ackermann, A. V. Lygin, and N. Hofmann, Org. Lett., 2011, 13, 3278; CrossRef (c) X. Yang, Y. Cheng, F. Zhao, Y. Li, Y. Ding, Y. Liang, F. Gan, and D. Dong, Tetrahedron, 2011, 67, 8343. CrossRef
18. I. Hachiya, K. Ogura, and M. Shimizu, Synthesis, 2004, 1349. CrossRef
19. A. H. Hunt, J. S. Mynderse, S. K. Samlaska, D. S. Fukuda, G. M. Maciak, H. A. Kirst, J. L. Occolowitz, J. K. Swartzendruber, and N. D. Jones, J. Antibiot., 1988, 41, 771 and references therein. CrossRef
20. For a synthesis of (-)-A58365A, see: (a) F. G. Fang and S. J. Danishefsky, Tetrahedron Lett., 1989, 30, 3621; CrossRef (b) K. D. Moeller and P. L. Wong, Bioorg. Med. Chem. Lett., 1992, 2, 739; CrossRef (c) P. L. Wong and K. D. Moeller, J. Am. Chem. Soc., 1993, 115, 11434; CrossRef (d) D. L. J. Clive, D. M. Coltart, and Y. Zhou, J. Org. Chem., 1999, 64, 1447; CrossRef (e) C. S. Straub and A. Padwa, Org. Lett., 1999, 1, 83; CrossRef (f) A. Padwa, S. M. Sheehan, and C. S. Straub, J. Org. Chem., 1999, 64, 8648; CrossRef (g) A. Reichelt, S. K. Bur, and S. F. Martin, Tetrahedron, 2002, 58, 6323. CrossRef
21. I. Hachiya, S. Fukushima, and M. Shimizu, Heterocycles, 2006, 69, 43. CrossRef
22. I. Hachiya, M. Atarashi, and M. Shimizu, Heterocycles, 2006, 67, 523. CrossRef
23. (a) J. T. Kuethe and A. Padwa, J. Org. Chem., 1997, 62, 774; CrossRef (b) S. I. Goldberg and A. H. Lipkin, J. Org. Chem., 1972, 37, 1823; CrossRef (c) E. E. V. Tamelen and J. S. Baran, J. Am. Chem. Soc., 1958, 80, 4659. CrossRef
24. S. M. Sheehan and A. Padwa, J. Org. Chem., 1997, 62, 438. CrossRef
25. I. Hachiya, W. Maehara, Y. Yamada, T. Kamiki, and M. Shimizu, Synlett, 2006, 3271. CrossRef
26. I. Hachiya, Y. Minami, T. Aramaki, and M. Shimizu, Eur. J. Org. Chem., 2008, 1411. CrossRef
27. (a) C. Hamdouchi, J. de Blas, M. de Prado, J. Gruber, B. A. Heinz, and L. Vance, J. Med. Chem., 1999, 42, 50; CrossRef (b) M. A. Ismail, R. Brun, T. Wenzler, F. A. Tanious, W. D. Wilson, and D. W. Boykin, J. Med. Chem., 2004, 47, 3658. CrossRef
28. A. H. Abadi and H. A. Al-Khamees, Arch. Pharm. Med. Chem., 1998, 331, 319. CrossRef
29. (a) Y. M. Elkholy and A. W. Erian, Heteroat. Chem., 2003, 14, 503; CrossRef (b) A.-Z. A.-A. Elassar, Heteroat. Chem., 2004, 15, 293. CrossRef
30. (a) P. Hong and H. Yamazaki, Tetrahedron Lett., 1977, 18, 1333; CrossRef (b) H. Hoberg and G. Burkhart, Synthesis, 1979, 525; CrossRef (c) P. Diversi, G. Ingrosso, A. Lucherini, and S. Malquori, J. Mol. Catal., 1987, 40, 267; CrossRef (d) D. D. Young and A. Deiters, Angew. Chem. Int. Ed., 2007, 46, 5187; CrossRef (e) T. Takahashi, F.-U. Tsai, Y. Li, H. Wang, Y. Kondo, M. Yamanaka, K. Nakajima, and M. Korota, J. Am. Chem. Soc., 2002, 124, 5059. CrossRef
31. For examples in transformation of 2-iminopyridines to 2-aminopyridines, see: (a) R. Adams and I. J. Pachter, J. Am. Chem. Soc., 1952, 74, 4906; CrossRef (b) K. Hartke, M. Sauebier, and W. F. Richter, Arch. Pharm., 1992, 325, 279. CrossRef
32. For recent examples, see: (a) S. A. S. Ghozlan and A. Z. A. Hassanien, Tetrahedron, 2002, 58, 9423; CrossRef (b) O. S. Moustafa and R. A. Ahmad, Phosphorus, Sulfur Silicon Relat. Elem., 2003, 178, 475; CrossRef (c) J. L. Marco, C. del los Ríos, A. G. García, M. Villarroya, M. C. Carreiras, C. Martins, A. Eleutério, A. Morreale, M. Orozco, and F. J. Luque, Bioorg. Med. Chem., 2004, 12, 2199; CrossRef (d) R. León, J. Marco-Contelles, A. G. García, and M. Villarroya, Bioorg. Med. Chem., 2005, 13, 1167; CrossRef (e) A.-G. E. Amr, A. M. Mohamed, S. F. Mohamed, N. A. Abel-Hafez, and A. E.-F. G. Hammam, Bioorg. Med. Chem., 2006, 14, 5481. CrossRef
33. I. Hachiya, T. Yoshitomi, Y. Yamaguchi, and M. Shimizu, Org. Lett., 2009, 11, 3266. CrossRef
34. For a review on the β-lactam synthon method, see: B. Alcaide, P. Almendros, and C. Aragoncillo, Chem. Rev., 2007, 107, 4437. CrossRef
35. 'Chemistry and Biology of β-Lactam Antibiotics,' eds. by R. B. Morin and M. Gorman, Academic Press, New York, 1982, Vols. 1-3.
36. For a review on the synthesis of β-lactams, see: A. Brandi, S. Cicchi, and F. M. Cordero, Chem. Rev., 2008, 108, 3988. CrossRef
37. For a recent example of thermal rearrangement of cyclobutenones, see: D. C. Harrowven, D. D. Pascoe, and I. L. Guy, Angew. Chem. Int. Ed., 2007, 46, 425. CrossRef