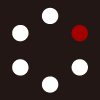
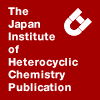
HETEROCYCLES
An International Journal for Reviews and Communications in Heterocyclic ChemistryWeb Edition ISSN: 1881-0942
Published online by The Japan Institute of Heterocyclic Chemistry
e-Journal
Full Text HTML
Received, 8th January, 2012, Accepted, 2nd February, 2012, Published online, 7th February, 2012.
DOI: 10.3987/REV-12-727
■ Total Synthesis of Aspergillides A, B, and C
Tomohiro Nagasawa and Shigefumi Kuwahara*
Graduate School of Agricultural Science, Tohoku University, 1-1, Tsutsumidori, Amamiya, Aoba-ku, Sendai, 981-8555, Japan
Abstract
Aspergillides A, B, and C are cytotoxic macrolides produced by the marine-derived fungus Aspergillus ostianus strain TUF 01F313. The unique molecular architectures of the aspergillides featuring 14-membered marocyclic structures embedded with a tetrahydro- or dihydropyran unit have attracted significant attention from the synthetic chemistry community, and thereby various synthetic approaches to these structurally as well as pharmacologically intriguing molecules have been reported by as many as 12 research groups in the past 3 years. This review describes all of the syntheses disclosed to date, focusing mainly on the methodologies employed for the diastereoselective installation of the pyran ring systems.CONTENTS
1. Introduction
2. First Synthesis and Stereochemical Revision of Aspergillide B by Hande and Uenishi
3. Synthesis of Aspergillides A, B, and C by Nagasawa and Kuwahara
4. Synthesis of Aspergillides A and B by the Marco Group
5. Synthesis of the Unnatural Enantiomer of Aspergillide B by She and Co-workers
6. Formal Synthesis of Aspergillide C by Panarese and Waters
7. Stereodivergent Synthesis of Aspergillides A and B by Fuwa and Co-workers
8. Formal Synthesis of Aspergillide A by Sabitha and Co-workers
9. Synthesis of the Unnatural Enantiomer of Aspergillide B by Hendrix and Jennings
10. Formal Synthesis of Aspergillide A by Takahashi and Co-workers
11. Stereodivergent Transannular Oxa-Michael Approach to Aspergillides A, B, and C by Shishido and Co-workers
12. Formal Synthesis of Aspergilide A by Fall and Co-workers
13. Synthesis of Both Enantiomers of Aspergillide C and Their Respective C4-Epimers by Srihari and Sridhar
1. INTRODUCTION
In 2003, Namikoshi and co-workers reported the identification of three new chlorine-containing γ- and δ-lactones with antibacterial activity from a seawater-containing 1/2 PD (potato-dextrose) culture medium of the marine-derived fungus Aspergillus ostianus strain TUF 01F313 isolated from an unidentified marine sponge collected at Pohnpei, Micronesia.1 This finding led Kusumi and co-workers to the expectation that some bromine-containing congeners might be obtained if the fungus is cultivated in a 1/2 PD medium containing bromine-modified artificial seawater instead of natural seawater. Contrary to their expectation, however, the modified cultivation conditions gave no such compounds, but instead, they succeeded in isolating three novel substances named aspergillides A–C,2,3 which exhibited significant cytotoxicity against mouse lymphocytic leukemia cells (L1210) with LD50 values of 2.1, 71.0, and 2.0 µg/mL, respectively. Based on extensive spectroscopic analyses including the modified Mosher method,4 the structures of the macrolides were initially proposed to be I, II, and III shown in Figure 1.
The molecular architectures of the aspergillides immediately captured the interest of synthetic organic chemists, since these compounds were the first examples of 14-membered macrolides incorporating a 2,6-trans-substituted tetrahydro- or dihydropyran ring as a nonhemiacetal form.5,6 The stereochemistries of aspergillides A and B (Figure 1) initially assigned by the Kusumi group were soon revised to those depicted in Figure 2 (compounds 1 and 2, respectively) through a synthetic study by Hande and Uenishi7 as well as X-ray crystallographic analysis of the corresponding m-bromonenzoates by Ooi and co-workers,8 while the originally proposed structure of aspergillide C (3) was confirmed by its total synthesis in our laboratory.9 Interestingly, the crystal structure of the m-bromobenzoate of 1 revealed that its tetrahydropyran moiety adopts the conformation shown in Figure 2 (mBrBz-1), in which the substituents at the C3, C4, and C7 positions are all axially oriented. As described later, this all-axial nature of the substituents in 1 made its synthesis by macrolactonization and ring-closing metathesis protocols quite difficult. The conformation of m-bromobenzoate of 2 (mBrBz-2) was, on the other hand, shown to accord with that predicted by Kusumi et al. from NOESY analysis of 2.2
The present review summarizes a variety of approaches to these structurally as well as pharmacologically intriguing molecules, which have culminated to date in seven, seven, and five total/formal syntheses of aspergilides A, B, and C, respectively.
2. FIRST SYNTHESIS AND STEREOCHEMICAL REVISION OF ASPERGILLIDE B BY HANDE AND UENISHI
Intrigued by the significant biological activity as well as the unique 2,6-trans-substituted tetrahydropyran structural motif embedded in the 14-membered macrocyclic ring, Hande and Uenishi embarked on the total synthesis of the originally proposed structures of aspergillides A and B (I and II in Figure 1). The most crucial step in their synthesis was the installation of the 2,6-trans tetrahydropyran ring system by a Pd(II)-catalyzed stereospecific ring-forming reaction developed by themselves.7
Their synthesis of I commenced with the protection of known olefinic ester 410 to 5 and subsequent Sharpless asymmetric dihydroxylation (ADH)11 to give 6 (Scheme 1). The lactone 6 was converted through 4 standard steps into 7, the cross-metathesis (CM)12 of which with allylic alcohol 813 using the second-generation Grubbs’ catalyst (Grubbs-II)14 followed by deprotection afforded 10 via 9. Treatment of 10 bearing a 2-heptene-1,7-diol structural unit with a catalytic amount of PdCl2(MeCN)2 in THF induced an efficient stereospecific cyclization accompanied by 1,3-chirality transfer from the C9 to C7 position,15 giving 3,7-trans-substituted tetrahydropyran derivative 11 in 76% yield along with a 3% yield of the corresponding 3,7-cis isomer (7-epi-11); the same reaction conditions, when applied to 9-epi-10, provided 7-epi-11 in an excellent yield of 80% together with a trace amount (2%) of 11. Methanolysis of 11 followed by TBS-protection in MeCN gave 12, the chain-elongation of which with 1316 by olefin cross-metathesis afforded an intermediate E-olefin exclusively. Hydrolysis of the two ester groups in the olefin then gave seco acid 14. Finally, the Yamaguchi lactonization17 of 14 and deprotection of the resulting macrolactone completed the total synthesis of I. Compound 12 was also converted into II by using ent-1316 as a coupling partner in the metathesis reaction via the same 4-step sequence employed for the conversion of 12 into I.
Unexpectedly, comparison of the spectral and specific rotation data of I and II with those reported for aspergillides A and B revealed that the data of I are identical with those reported for aspergillide B, and structure II represents neither aspergillide A nor aspergillide B. Based on these facts, the stereochemistry of aspergillide B was revised as shown in Figure 2 (compound 2), while the structure of aspergillide A was thrown into confusion. As mentioned above, this deadlock concerning the real structure of aspergillide A was solved through X-ray crystallographic studies by Ooi et al., and structure 1 was assigned to aspergillide A as its genuine structure.
3. SYNTHESIS OF ASPERGILLIDES A, B, AND C BY NAGASAWA AND KUWAHARA
The first synthesis of aspergillide C (3) was achieved in our laboratory by utilizing a Lewis acid-promoted nucleophilic substitution of a dihydropyranyl acetal intermediate with a silyl ketene acetal to construct its 2,6-trans-substituted dihydropyran core structure (Scheme 2).9
Sulfone segment 17 was prepared from known ester 15 via alcohol 16 in 75% overall yield by a straightforward 6-step sequence. The preparation of cyclic acetal fragment 21, which was required for chain-elongation with the sulfone unit 17, was carried out from commercially available glycidol derivative 18 via 3 steps including a one-pot sequence consisting of semi-hydrogenation, deprotection, and cyclic acetal formation (19 → 20). The Julia-Kocienski olefination18 of 21 with 17 gave 22 as a 10:1 E/Z mixture favoring the desired E isomer. BF3·OEt2-promoted substitution of the acetal 22 with silyl ketene acetal 23 via an oxocarbenium ion intermediate19 proceeded with moderate diastereoselectivity, providing the 2,6-trans-substituted dihydropyran 24 in 65% isolated yield along with the corresponding cis isomer (25%). Saponification of 24 followed by in situ iodolactonization gave 25, which was subjected to elimination to afford 26 in 45% yield for the 2 steps. The modest chemical yield was ascribed to the fact that the β-elimination of 25 requires an energetically unfavorable conformation in which the iodine atom and the olefinic side chain are in a 1,3-diaxial relationship. After a 3-step functional group manipulation of 26, the resulting seco acid 27 was exposed to the Yamaguchi lactonization and subsequent deprotection conditions to furnish aspergillide C (3), identical spectroscopically to natural aspergillide C. Subjection of the iodolactone intermediate 25 to radical reduction conditions, on the other hand, gave 28, which was converted into aspergillide B (2) in 55% overall yield by the same 5-step sequence as employed for the conversion of 26 into 3.20
For the synthesis of aspergillide A (1) incorporating the 2,6-cis-substituted tetrahydropyran core, an efficient proline-catalyzed trans-to-cis isomerization21 of intermediate 29 derived from 28 in 4 steps was employed, providing, via a retro-oxa-Michael/oxa-Michael sequence, the desired thermodynamically more stable product 30 with excellent selectivity (cis/trans = 95:5) in an isolated yield of 81% (Scheme 3). The aldehyde 30 was converted into seco acid 31 and subjected to the Yamaguchi macrolactonization conditions to furnish 32, albeit in a modest yield of 30%, together with dimeric macrodiolide 33 and some unidentified products. Several attempts to improve the yield of 32 by varying the reaction conditions did not give any better outcome, and the application of the Mukaiyama, Gerlach, or Shiina macrolactonization protocols22 afforded none of the desired product, resulting in the formation of complex mixtures or the production of 33 in low yield under Shiina’s conditions. Finally, removal of the silyl protecting group completed the first synthesis of aspergillide A (1).23
4. SYNTHESIS OF ASPERGILLIDES A AND B BY THE MARCO GROUP
Marco and co-workers accomplished the synthesis of apergillides A (1) and B (2) using two types of Lewis acid-induced nucleophilic substitution reactions for the installation of the 2,6-cis- and 2,6-trans-substituted tetrahydropyran moieties embedded in 1 and 2, respectively.24
Their synthesis of 2 began with the ozonolysis of the benzyl ether of known olefinic alcohol 3425 to deliver 35 (Scheme 4). The aldehyde 35 was subjected to Brown’s asymmetric allylboration26 to give 36 (dr >95:5) after TES-protection of the resulting alcohol. Migration of its terminal double bond to the adjacent internal position was implemented efficiently by Wipf’s procedure27 to give 37 as a ca. 9:1 E/Z mixture, which was then deprotected and oxidized28 to lactone 38. Reduction of 38 followed by in situ acetylation of the resulting lactol29 furnished 39, which was treated with the TMS enol ether derived from t-butyl thioacetate in the presence of BF3·OEt2 and TMSOTf to induce the addition of the nucleophile to an oxocarbenium ion intermediate generated from 39. The reaction proceeded with moderate diastereoselectivity, giving 40 and its C3-epimer in yields of 55% and 21%, respectively. Saponification of 40 followed by cross-metathesis with 4130 afforded seco acid 42 as a 7:3 E/Z mixture, which was then subjected to the Yamaguchi lactonizaion. The desired macrolide 43, obtained in 40% isolated yield after purification, was converted into aspergillide B (2) by debenzylation with DDQ in wet dichloromethane.24a
To obtain aspergillide A (1) bearing the 2,6-cis-substituted tetrahydropyran core, the lactone 38 was first exposed to an aldol reaction with ethyl acetate (Scheme 5), and the resulting lactol 44 was reduced with Et3SiH/BF3·OEt219 to furnish 45 with exclusive 3,7-cis selectivity. Hydrolysis of 45 and subsequent esterification with 41 gave 46, the ring-closing metathesis (RCM)31 of which using the first-generation Grubbs’ catalyst afforded 8,9-Z macrolactone 47 as a sole product in 99% yield. The exclusive formation of 47 was explained based on molecular mechanics calculations which indicated 47 to be much more stable than the corresponding E isomer and therefore the thermodynamically controlled product; the authors also deduced that the formation of 47 might be kinetically favored due to certain conformational restraints imposed by the presence of the bridged tetrahydropyran ring.32 Finally, deprotection of 47 and subsequent photochemical isomerization of the resulting alcohol 48 provided aspergillide A (1), albeit in a modest isolated yield of 30% [56% based on recovered starting material (brsm)]. It is worth mentioning that compound 48 exhibited much higher cytotoxicity (18–45 times) than 1 and 2 against the human cancer cell lines HL-60 and MDA-MB-231.24c
5. SYNTHESIS OF THE UNNATURAL ENANTIOMER OF ASPERGILLIDE B BY SHE AND CO-WORKERS
The synthesis of the unnatural enantiomer of aspergillide B (ent-2) by She and co-workers exploited a Lewis acid-promoted nucleophilic substitution of d-galactose pentaacetate 53 with allylsilane to construct the 2,6-trans-substituted tetrahydropyran structural motif (Scheme 6).33
Sulfone segment 52 was prepared in 4 steps consisting of ring-opening of the starting epoxide 49, protection to form 50, hydroboration–oxidation to alcohol 51, and sulfoxide formation via the Mitsunobu reaction.18,34 The construction of the 2,6-trans-substituted tetrahydropyran ring system in ent-2 was performed by nucleophilic addition of allylsilane to a cyclic oxocarbenium ion intermediate generated from 53 followed by solvolytic deprotection and TBS-protection to give 54.35 The cis-diol moiety of 54 was selectively converted into its cyclic orthoester, and the remaining β-oriented hydroxy group was protected as its MOM ether 55. Ozonolysis of 55 in methanolic NaOH directly afforded the corresponding methyl ester,36 the orthoester functionality of which was then pyrolytically converted to a double bond to furnish 56.37 After a 3-step functional group manipulation of 56, the product 57 was coupled with the sulfone 52 to give 58 in 57% yield along with the corresponding Z isomer (5%). Removal of the TBS protecting group of 58 was followed by ester hydrolysis to deliver seco acid 59. Exposure of 59 to the Mitsunobu inversion conditions afforded macrolactone 60, which was smoothly deprotected by treatment with LiBF4 in aqueous MeCN to furnish the target molecule ent-2.
6. FORMAL SYNTHESIS OF ASPERGILLIDE C BY PANARESE AND WATERS
In their formal synthesis of aspergillide C (3), Waters and co-workers combined a ZnCl2-mediated hetero-Diels–Alder reaction with a Ferrier-type substitution to construct the 2,6-trans-substituted tetrahydropyran moiety in 3, and exploited a Pd(II)-catalyzed oxidative cyclization to install the C4 oxygen functionality as well as the C5–C6 double bond (Scheme 7).38
The sulfone segment 17 was prepared in a straightforward fashion from commercially available olefinic alcohol 61 via 16. Preparation of its coupling partner 69 was, on the other hand, commenced with the hetero-Diels–Alder reaction of the Danishefsky–Kitahara diene 62 and aldehyde 63 prepared from l-arabinose to give 64 as a single diastereomer.39 The product 64 was reduced and then acetylated to afford 65, which was exposed to the TBS enol ether of acetaldehyde in EtOAc in the presence of LiClO4. The resulting Ferrier reaction product 66 obtained as an almost exclusive diastereomer was oxidized to γ,δ-unsaturated carboxylic acid 67, the Pd(II)-catalyzed oxidative cyclization of which according to Larock’ protocol40 afforded lactone 68 with double bond migration. Hydrolysis of the acetonide in 68 and oxidative cleavage of the resulting diol intermediate with silica gel-supported NaIO4 gave aldehyde 69, which was then condensed with the sulfone 17 to furnish almost exclusively the E-olefin 26, an intermediate in the total synthesis of aspergillide C by Nagasawa and Kuwahara (Scheme 2).
7. STEREODIVERGENT SYNTHESIS OF ASPERGILLIDES A AND B BY FUWA AND CO-WORKERS
Fuwa and co-workers achieved the total synthesis of aspergillides A and B based on a stereodivergent strategy which exploited thermodynamic and kinetic intramolecular oxa-conjugate addition reactions on a common acyclic intermediate for the installation of the 2,6-cis- and 2,6-trans-substituted tetrahydropyran cores, respectively.41
Known alcohol 70 was converted into allylic alcohol 71 by a 4-step sequence consisting of protection, chemoselective hydroboration/oxidation, TEMPO oxidation followed by in situ Wittig olefination (E/Z >20:1), and DIBAL reduction (Scheme 8). The Sharpless asymmetric epoxidation of 71 gave 72 as a single diastereomer, and the epoxy alcohol 72 was converted into 73 in 2 steps via iodination and zinc reduction. Treatment of 73 with methyl acrylate in the presence of the second-generation Grubbs’ catalyst furnished 74 as a sole isolable product without giving even a trace amount of the possible six-membered RCM product. This remarkable chemoselectivity was ascribed to the formation of a hydrogen bonding between the OH at the C4 position and one of the two chlorine atoms on the ruthenium atom in an intermediary ruthenium alkylidene complex, which would render the RCM process unfavorable. Protection of 74 as its MOM ether and subsequent desilyation afforded hydroxy enoate 75, the pivotal intermediate for this stereodivergent synthesis of 1 and 2. Exposure of 75 to a catalytic amount of KOt-Bu in THF at –78 °C for 30 min provided trans-76 as a kinetically controlled product with an excellent diastereoselectivity of 17:1. The preparation of the corresponding cis-isomer (cis-76) from the common intermediate 75, on the other hand, proved to be difficult contrary to their expectation that the thermodynamically more favored isomer cis-76 would be obtained predominantly by just elevating the reaction temperature. After several unsuccessful attempts, they found the best conditions (DBU, toluene, 135 °C, 36 h) to efficiently promote the equilibration between trans- and cis-76, providing cis-76 in good yield (81%) and diastereoselectivity (11:1), and thus realizing their stereodivergent strategy to obtain both trans- and cis-76 (precursors to 2 and 1, respectively) from the common intermediate 75.
Ozonolytic cleavage of the double bond of trans-76 and subsequent application of Takai’ protocol afforded 77 (E/Z = ca. 5:1) (Scheme 9). The (E)-vinyl iodide 77 was, after separation, subjected to B-alkyl Suzuki–Miyaura coupling with olefin 78,42 prepared in 2 steps from (S)-propylene oxide, to give 79. Hydrolysis of 79 and treatment of the resulting seco acid trans-80 under the Yamaguchi lactonization conditions furnished mactolactone trans-81, the deprotection of the MOM group of which with LiBF4 completed the total synthesis of aspergillide B (2). Exposure of cis-76 to the same 6-steps sequence employed for the conversion of trans-76 into 2 gave aspergillide A (1), although the macrolactonization of cis-80 to cis-81 was low-yielding as in the case of the synthesis by Nagasawa and Kuwahara (Scheme 3);23 they reasoned the difficulty would be ascribable to the fact that cis-80 with an all-equatorial conformation needs to flip to an energetically unfavorable all-axial conformer, which is adopted by aspergillide A itself (Figure 2), for the macrolactonization to take place.
8. FORMAL SYNTHESIS OF ASPERGILLIDE A BY SABITHA AND CO-WORKERS
Sabitha and co-workers reported two approaches to 2,6-cis-substituted tetrahydropyran core 87 (Scheme 10) which was successfully transformed into two intermediates, 31 and 48 (Scheme 11), employed in previous syntheses of aspergillide A (1).43
Their first approach to 87 began with ring-opening homologation of terminal epoxide 8244 obtained from the corresponding racemate by Jacobsen’s kinetic resolution.45 Conjugate addition of the resulting allylic alcohol 83 to methyl propiolate afforded 84, the treatment of which with acidic methanol followed by IBX oxidation provided 85. Subjection of the β-alkoxy acrylate 85 containing an aldehyde group in the molecule to Nakata’s SmI2-induced reductive cyclization furnished 86 as a single diastereomer,46 which was then protected and hydrolyzed to the key intermediate 87.
The other approach to 87 featuring an intramolecular oxa-Michael addition under thermodynamic conditions commenced with the preparation of 89 from epoxy alcohol 8847 in 2 steps consisting of iodination and reductive epoxide ring-opening. The allylic alcohol 89 was converted by a 3-step sequence via 90 into aldehyde 91, which on exposure to proline-catalyzed asymmetric α-aminooxylation conditions48 followed by in situ Horner–Wadsworth–Emmons olefination and subsequent Cu(II)-catalyzed N–O bond cleavage49 gave 94 with excellent diastereoselectivity (>95:5) via 92 and 93. Treatment of 94 with TBAF induced deprotection of the TBS group and concomitant intramolecular oxa-Michael addition to exclusively form 86, which was converted into 87 as described above.
The olefinic carboxylic acid 87 was esterified with the known alcohol 4130 to give 95, the treatment of which with the first-generation Grubbs’ catalyst resulted in the exclusive formation of Z-macrolactone 96 in 90% yield in accord with the result observed in the corresponding Bn-protected congener (Scheme 5). Finally, deprotection of 96 gave 48, the penultimate intermediate in the previous synthesis of 1 by Marco’s group (Scheme 5). In addition, the cross-metathesis of 87 with 41 provided a mixture of 31 and the corresponding Z-isomer in a ratio of 9:1, the former which had previously been converted into aspergillide A (1) by Nagasawa and Kuwahara (Scheme 3).
9. SYNTHESIS OF THE UNNATURAL ENANTIOMER OF ASPERGILLIDE B BY HENDRIX AND JENNINGS
The synthesis of the unnatural enantiomer of aspergillide B (ent-2) by Hendrix and Jennings features the formation of the tetrahydropyran core by ring-closing metathesis coupled with nucleophilic substitution at the C7-position via an oxocarbenium ion intermediate (Scheme 12).50
They employed Brown’s asymmetric alkoxyallylboration on aldehyde 97 as the chirality-inducing step,51 providing 98 with excellent diastereomeric and enantiomeric purity (dr >95:5, 94% ee). Esterification of 98 with acryloyl chloride gave 99, which was then subjected to ring-closing metathesis using the second-generation Grubbs’ catalyst to furnish 100. The unsaturated lactone 100 was converted straightforwardly into 101, the oxocarbenium ion precursor. Treatment of 101 with allylsilane in the presence of BF3·OEt2 afforded 2,6-trans tetrahydropyran derivative 102 as a single diastereomer. Isomerization of the terminal double of 102 was effected by its treatment with the second-generation Grubbs’ catalyst according to Hanessian’s procedure52 to give 103 as an 8:1 E/Z mixture, the cross-metathesis of which with olefin 104 derived from (R)-propylene oxide then afforded 105 with high geometrical selectivity (E/Z >20:1). Selective deprotection of its primary TBS group gave 106, which was converted into 107 via 3 conventional steps. Finally, the seco acid 107 was exposed to the Yamaguchi lactonization conditions to provide ent-2 after removal of the MOM protecting group of the macrolactonization product 60 (She’s intermediate, Scheme 6).
10. FORMAL SYNTHESIS OF ASPERGILLIDE A BY TAKAHASHI AND CO-WORKERS
The formal synthesis of aspergillide A (1) by Takahashi and co-workers exploited, as key steps, the CBS reduction of an enone intermediate to install a stereogenic center and SmI2-induced reductive cyclization of a formyl-containing β-alkoxy acrylate derivative, originally developed by their group and employed in Sabitha’s approach to 1 (Scheme 10), for the construction of the 2,6-cis-substituted tetrahydropyran ring system (Scheme 13).53
Enone 109, obtained from known aldehyde 10854 by a 2-step sequence, was treated with terminal olefin 11055 in the presence of the second-generation Grubbs’ catalyst to give 111, which upon subjection the CBS reduction56 afforded allylic alcohol 112 with good diastereoselectivity (dr 94:6). Oxa-conjugate addition of 112 to ethyl propiolate in the presence of N-methylmorpholine (NMM) gave β-alkoxy enoate 113, and its dithiane group was hydrolyzed to provide 114. Upon exposure to SmI2 in THF in the presence of MeOH,46 114 was transformed in 96% yield into a 10:1 mixture of 115 and its (3S,4R)-isomer. Treatment of the mixture with MOMBr in dichloromethane in the presence of the Hünig base and subsequent purification of the product gave a diastereomerically pure MOM-protected intermediate, which was subjected to a 2-step deprotection sequence to furnish seco-acid cis-80, an intermediate in Fuwa’s synthesis of aspergillide A (Scheme 9).
11. STEREODIVERGENT TRANSANNULAR OXA-MICHAEL APPROACH TO ASPERGILLIDES A, B, AND C BY SHISHIDO AND CO-WORKERS
Shishido and co-workers reported a remarkable approach to aspergillides A (1) and B (2) which exploited highly efficient stereodivergent transannular oxa-Michael reactions of a common macrocyclic intermediate (125) involving the formation of either the 2,6-cis- or 2,6-trans-tetrahydropyran ring system depending on the reaction conditions (Scheme 14).57
Their synthesis of 1 and 2 began with the stereoselective reduction of known enone 11658 to form allylic alcohol 117, which was converted into sulfide 118 according to Hata’s protocol.59 Application of allyl sulfoxide–sulfenate rearrangement to 118 afforded 119 after TBS-protection. Hydrogenation of 119 accompanied by concomitant debenzylation and a subsequent mesylation/iodination sequence provided iodide 120. Treatment of 120 with Zn in ethanol induced reductive ring-opening, furnishing cyclic hemiacetal 121. Reduction of 121 with LiBH4 gave a diol intermediate, the primary hydroxy group of which was protected as its pivaloyl ester and the remaining secondary one as its TES ether. The ester was then reduced to alcohol 122, which was subjected to cross-metathesis with olefin 123, prepared from (S)-2-methyloxirane in 2 steps, affording 124 with high geometrical selectivity (E/Z >20:1). Oxidation of the alcohol 124 to an aldehyde followed by intramolecular olefination under the Masamune–Roush conditions60 and deprotection in acidic medium delivered macrocyclic hydroxy enoate 125, the pivotal common substrate for the stereodivergent transannular oxa-Michael reactions leading either to 1 or to 2.
Upon exposure to DBU in MeCN in the presence of LiCl at room temperature for 1.5 h, 125 was quantitatively converted into 126 with the 3,7-cis stereochemistry as a single product. On the other hand, treatment of 125 with KH in THF in the presence of 18-crown-6 at 0 °C for 0.5 h gave the corresponding 3,7-trans isomer 127 as a single diastereomer in a very high yield of 96%. The addition of LiCl in the formation 126 enabled the transannular reaction to proceed much faster than the reaction without it; the authors reasoned this effect by suggesting lithium-chelated transition state 125’, the conformation of which is quite similar to that of the crystal structure of aspergillide A. Furthermore, it was revealed that the transformation of the 3,7-cis isomer 126 to the 3,7-trans isomer 127 could be achieved in very high yield (94%), while the reverse reaction from 127 to 126 was not realized, resulting the recovery of 127 in 90% yield. These results as well as some additional experiments indicated that the trans-isomer 127 was thermodynamically favored. Finally, hydrolytic removal of the TBS protecting group in 126 and 127 completed the total synthesis of aspergillides A (1) and B (2), respectively. Very interestingly, the conversion of 1 into 2 was also found to be possible by treating 1 with the same conditions as employed for the transformation of 126 to 127, while the exposure of 1 to SiO2 in MeOH/CHCl3 (isolation conditions for the aspergillides) did not produce 2 at all, suggesting aspergillide B (2) is not an artifact.
Shishido and co-workers also disclosed two syntheses of aspergillide C (3) (Scheme 15), the first one of which utilized, as the key step, an intramolecular oxa-Michael reaction before macrolactonization, leading exclusively to a 2,6-trans-substituted pyran intermediate,61 and the second one adopted the highly efficient transannular oxa-Michael strategy as employed for their synthesis of aspergillides A and B described above.62
Their first approach to 3 commenced with ring-opening of epoxide 128 with vinylmagnesium chloride followed by acrylation of the resulting alcohol to deliver 129.61 Ring-closing metathesis of 129 gave lactone 130, which was converted by a conventional 3-steps sequence into hydroxy enoate 131. Intramolecular oxa-Michael reaction of 131 by exposure to LiOH in aqueous THF (THF/H2O = 3:1) proceeded very efficiently, furnishing cyclic carboxylic acid 132 with 3,7-trans stereochemistry as a single diastereomer in 94% yield. They revealed that the presence of water in this transformation was essential for acceleration of the reaction and for realizing the high yield and excellent diastereoselectivity, and also that the hydrolysis of the ester group took place after the ring formation. The use of NaOH and KOH resulted in lower yields (79% and 85%, respectively) and diastereoselectivity (6.1:1 and 6.3:1, respectively). From these results, the authors proposed a lithium-chelated transition state responsible for the exclusive formation of the 3,7-trans isomer 132. Reduction of 132 in 2 steps and a subsequent 3-step functional group manipulation on the resulting alcohol 133 gave aldehyde 134, the Julia–Kocienski olefination of which with sulfone 5233 afforded E-olefin 135 predominantly (E/Z >10:1). Installation of the double bond on the tetrahydropyran ring to form 136 was conducted by the Fuchs modification of Noyori’s protocol.63 After selective removal of the two TBS groups in 136, the resulting diol 137 was converted by a straightforward 5-step sequence, via γ-lactone 138 and MOM ester 139, into seco acid 140, the macrolactonization of which followed by deprotection furnished aspergillide C (3).
The second-generation synthesis of 3 by Shishido and co-workers adopted 119, an intermediate in their synthesis of aspergillides A and B (Scheme 14), as the starting point (Scheme 16).62 Debenzylation of 119 followed by a 3-step transformation gave lactol 141, the cross-metathesis of which with terminal olefin 14264 to provide 143 and a subsequent olefination/protection/deprotetion sequence furnished seco acid 144. The Shiina macrolactonization of 144 proceeded in a better yield of 92% than the Yamaguchi protocol (56%),22 affording 146, the desilylation of which provided dihydroxy macrolactone 147. Treatment of 147 with KH in THF in the presence of 18-crown-6 for 10 min at 0 °C gave aspergillide C (3) as a single diastereomer in 86% yield, thus realizing the unified total synthesis of all of the aspergillides based on their transannular oxa-Michael strategy. Exposure of 147 to DBU in MeCN in the presence of LiCl for 15 min at room temperature, on the other hand, led to the exclusive formation of the corresponding 3,7-cis isomer (3-epi-3) in 97% yield. Upon subjection to the same conditions as employed for the conversion of 147 into 3, 3-epi-3 was transformed into 3, which, coupled with some additional experiments, clearly indicated that aspergillide C (3) and its C3-epimer (3-epi-3) were thermodynamic and kinetic products, respectively.
12. FORMAL SYNTHESIS OF ASPERGILLIDE A BY FALL AND CO-WORKERS
Fall and co-workers reported the synthesis of the 2,6-cis-substituted tetrahydropyran derivative 87, an intermediate in Sabitha’s formal synthesis of aspergillide A (1) (Scheme 10), using commercially available tri-O-acetyl-d-glucal 148 as the starting material and the Claisen rearrangement as the key step to install the stereochemistry at the C7 position (Scheme 17).65
The glucal 148 was converted into allyl vinyl ether 149 via solvolytic removal of the acetate groups, protection of the resulting 1,3-diol moiety, and finally enol ether formation. The Claisen rearrangement of 149 afforded 150 with the desired stereochemical relationship, the double bond and the aldehyde groups of which were reduced in 2 steps to give 151. Iodination of the alcohol 151 followed by elimination and desilylation furnished diol 152. Its secondary hydroxy group was protected by a 2-step sequence, delivering 153, which was then transformed, by a conventional 4-step sequence of reactions via nitrile 154, into the target molecule 87.
13. SYNTHESIS OF BOTH ENANTIOMERS OF ASPERGILLIDE C AND THEIR RESPECTIVE C4-EPIMERS BY SRIHARI AND SRIDHAR
The most recent approach to aspergillides was disclosed by Srihari and Sridhar in their total synthesis of aspergillide C (3) and its stereoisomers, which included the Sharpless kinetic resolution of a furfuryl alcohol derivative as the chirality-inducing step and a Lewis acid-promoted nucleophilic substitution of a dihydropyranyl acetate intermediate with a silyl acetylene to construct the 2,6-trans-substituted dihydropyran ring system (Schemes 18 and 19).66
The silyl acetylene 155 was prepared in a 5-step straightforward manner from (S)-propylene oxide 49, via olefinic alcohol 41. To obtain its coupling partner 159, they utilized the Sharpless kinetic resolution of the furfuryl alcohol derivative rac-157 derived from furfural 156,67 providing oxidation product 158 along with enantiopure (R)-157. The lactol 158 was acetylated to 159 and then treated with the silyl acetylene 155 in dichloromethane in the presence of SnCl4,68 furnishing 2,6-trans-substituted dihydropyran derivative 160 as a single diastereomer in 4 steps from the simple starting material 156. The installation of the E double bond at the C8 position was conducted by Trost’s hydrosilylation/protodesilylation protocol,69 resulting in the exclusive formation of E-olefin 161. Subjection of the enone 161 to the CBS reduction conditions gave allylic alcohol 162 in 80% yield together with the corresponding C4-epimer (9%). After MOM-protection of 162 followed by hydrolysis, the resulting seco acid 140 (Shishido’s intermediate, Scheme 15) was converted via 2 steps into aspergillide C (3).
They also synthesized the 4-epi-aspergillide C (4-epi-3) as well as the enantiomers of aspergillide C and 4-epi-3 (ent-3 and ent-4-epi-3, respectively) for future evaluation of their biological activity (Scheme 19). The syntheses are based on their findings that compound 161, when exposed to NaBH4/CeCl3/MeOH, gave a separable mixture of the 4-epi-162 and 162 in a ratio of 4:1 favoring the former, and that (R)-157 was readily convertible into the enantiomer of 158 (ent-158) by NBS-oxidation in aqueous medium.
14. CONCLUSION
This review described all of the synthetic approaches to aspergillides A (1), B (2), and C (3) reported in the past 3 years by 12 research groups, focusing mainly on the methodologies for the construction of the 2,6-cis- and 2,6-trans-substituted tetrahydropyran ring systems contained in 1 and 2, respectively, and of 2,6-trans-substituted dihydropyran core incorporated in 3. The methodologies may be categolized into 6 groups: (1) Pd(II)-catalyzed stereospecific ring formation (Scheme 1); (2) nucleophilic substitution via an oxocarbenium ion intermediate (Schemes 2, 4, 5, 6, 7, 12, 18); (3) intramolecular oxa-Michael addition (Schemes 3, 8, 10, 15); (4) SmI2-induced intramolecular reductive cyclization (Schemes 10, 13); (5) stereodivergent transannular oxa-Michael addition (Schemes 14, 16); and (6) the Claisen rearrangement (Scheme 17). The Pd(II)-catalyzed cyclization employed in the first synthesis of 2 by Hande and Uenishi (Scheme 1) deserves special mention in that the reaction is stereospecific and therefore can be applied to the construction of both 2,6-cis- and 2,6-trans-substituted tetrahydropyran units contained in various natural products. Among the intramlecular oxa-Michael approaches (Category 3), the one reported by Fuwa and co-workers (Scheme 8) is also worthy to note in that the methodology is stereodivergent, giving rise to either the trans- or the cis-substituted ring system at will by choosing reaction conditions. In addition, the synthesis of 3 and its stereoisomers by Srihari and Sridhar that nicely incorporated the readily available cyclic hemiacetal 158 in their concise synthetic design (Scheme 18) could be of importance from a viewpoint of stereochemistry–activity relationship. Finally, the strategy presented by Shishido and co-workers (Schemes 14, 16) that enabled the highly efficient stereodivergent synthesis of aspergillides A–C and overcame the difficulty in the macrocyclization of aspergillide A precursors (Schemes 3, 5, 9, 11) is quite useful and will be applied, as a general methodology, to the synthesis of various macrocyclic natural products incorporating a small ring in their structures.
References
1. M. Namikoshi, R. Negishi, H. Nagai, A. Dmitrenok, and H. Kobayashi, J. Antibiot., 2003, 56, 755.
2. K. Kito, R. Ookura, S. Yoshida, M. Namikoshi, T. Ooi, and T. Kusumi, Org. Lett., 2008, 10, 225. CrossRef
3. The compound name “aspergillide” had previously been given to metabolites of Aspergillus terreus with structures totally different from those of the aspergillides discussed in this review. For details, see: B. T. Golding and R. W. Rickards, J. Chem. Soc., Perkin Trans. 1, 1975, 1961.
4. I. Ohtani, T. Kusumi, Y. Kashman, and H. Kakisawa, J. Am. Chem. Soc., 1991, 113, 4902. CrossRef
5. For the only natural 14-membered macrolide (neopeltolide) containing a 2,6-cis-substituted tetrahydropyran ring as a nonhemiacetal form, see: (a) A. E. Wright, J. C. Botelho, E. Guzmán, D. Harmody, P. Linley, P. J. McCarthy, T. P. Pitts, S. A. Pomponi, and J. K. Reed, J. Nat. Prod., 2007, 70, 412; CrossRef (b) W. Youngsaye, J. T. Lowe, F. Pohlki, P. Ralifo, and J. S. Panek, Angew. Chem. Int. Ed., 2007, 46, 9211. CrossRef
6. A benzo-fused 14-membered macrolide (pochonin J) embedded with a 2,6-trans-substituted tetrahydropyran ring as a nonhemiacetal form has recently been reported by Shinonaga and co-workers. For details, see: H. Shinonaga, Y. Kawamura, A. Ikeda, M. Aoki, N. Sakai, N. Fujimoto, and A. Kawashima, Tetrahedron Lett., 2009, 50, 108. CrossRef
7. S. M. Hande and J. Uenishi, Tetrahedron Lett., 2009, 50, 189. CrossRef
8. R. Ookura, K. Kito, Y. Saito, T. Kusumi, and T. Ooi, Chem. Lett., 2009, 38, 384. CrossRef
9. T. Nagasawa and S. Kuwahara, Org. Lett., 2009, 11, 761. CrossRef
10. C. S. Pak, E. Lee, and G. H. Lee, J. Org. Chem., 1993, 58, 1523. CrossRef
11. H. C. Kolb, M. S. VanNieuwenhze, and K. B. Sharpless, Chem. Rev., 1994, 94, 2483. CrossRef
12. S. J. Connon and S. Blechert, Angew. Chem. Int. Ed., 2003, 42, 1900. CrossRef
13. I. Sato, N. Asakura, and T. Iwashita, Tetrahedron: Asymmetry, 2007, 18, 2638. CrossRef
14. M. Scholl, S. Ding, C. W. Lee, and R. H. Grubbs, Org. Lett., 1999, 1, 953. CrossRef
15. (a) J. Uenishi and M. Ohmi, Angew. Chem. Int. Ed., 2005, 44, 2756; CrossRef (b) J. Uenishi, Y. S. Vikhe, and N. Kawai, Chem. Asian J., 2008, 3, 473, and references cited therein. CrossRef
16. (a) H. Takahata, Y. Yotsui, and T. Momose, Tetrahedron, 1998, 54, 13505; CrossRef (b) A. Fürstner, O. R. Thiel, and L. Ackermann, Org. Lett., 2001, 3, 449. CrossRef
17. J. Inanaga, K. Hirata, H. Saeki, T. Katsuki, and M. Yamaguchi, Bull. Chem. Soc. Jpn., 1979, 52, 1989. CrossRef
18. P. R. Blakemore, W. J. Cole, P. J. Kocienski, and A. Morley, Synlett, 1998, 26. CrossRef
19. M. D. Lewis, J. K. Cha, and Y. Kishi, J. Am. Chem. Soc., 1982, 104, 4976. CrossRef
20. T. Nagasawa and S. Kuwahara, Biosci. Biotechnol. Biochem., 2009, 73, 1893. CrossRef
21. A. Massi, A. Nuzzi, and A. Dondoni, J. Org. Chem., 2007, 72, 10279. CrossRef
22. For a recent review on macrolactonization, see: A. Parenty, X. Moreau, and J.-M. Campagne, Chem. Rev., 2006, 106, 911. CrossRef
23. (a) T. Nagasawa and S. Kuwahara, Tetrahedron Lett., 2010, 51, 875; CrossRef (b) T. Nagasawa, T. Nukada, and S. Kuwahara, Tetrahedron, 2011, 67, 2882. CrossRef
24. (a) S. Díaz-Oltra, C. A. Angulo-Pachón, M. N. Kneeteman, J. Murga, M. Carda, and J. A. Marco, Tetrahedron Lett., 2009, 50, 3783; CrossRef (b) S. Díaz-Oltra, C. A. Angulo-Pachón, J. Murga, M. Carda, and J. A. Marco, J. Org. Chem., 2010, 75, 1775; CrossRef (c) S. Díaz-Oltra, C. A. Angulo-Pachón, J. Murga, E. Falomir, M. Carda, and J. A. Marco, Chem. Eur. J., 2011, 17, 675. CrossRef
25. D. J. Dixon, S. V. Ley, and D. J. Reynolds, Chem. Eur. J., 2002, 8, 1621. CrossRef
26. P. V. Ramachandran, Aldrichimica Acta, 2002, 35, 23.
27. P. Wipf, S. R. Rector, and H. Takahashi, J. Am. Chem. Soc., 2002, 124, 14848. CrossRef
28. T. M. Hansen, G. J. Florence, P. Lugo-Mas, J. Chen, J. N. Abrams, and C. J. Forsyth, Tetrahedron Lett., 2003, 44, 57. CrossRef
29. V. H. Dahanukar and S. D. Rychnovsky, J. Org. Chem., 1996, 61, 8317. CrossRef
30. D. J. Dixon, S. V. Ley, and E. W. Tate, J. Chem. Soc., Perkin Trans. 1, 2000, 2385. CrossRef
31. A. H. Hoveyda and A. R. Zhugralin, Nature, 2007, 450, 243. CrossRef
32. Analogous results were obtained in RCM approaches to 1 and 2 by Fuwa and co-workers (not described in this review), in which more detailed discussions on preferential formation of 8,9-Z macrolides were made on the basis of conformational analysis by NOE experiments and theoretical calculations (see Ref. 41b).
33. J. Liu, K. Xu, J. He, L. Zhang, X. Pan, and X. She, J. Org. Chem., 2009, 74, 5063. CrossRef
34. (a) O. Mitsunobu, Synthesis, 1981, 1; CrossRef (b) K. C. K. Swamy, N. N. B. Kumar, E. Balaraman, and K. V. P. P. Kumar, Chem. Rev., 2009, 109, 2551. CrossRef
35. K. Fujiwara, S. Souma, H. Mishima, and A. Murai, Synlett, 2002, 1493. CrossRef
36. J. A. Marshall and A. W. Garofalo, J. Org. Chem., 1993, 58, 3675. CrossRef
37. M. Ando, H. Ohhara, and K. Takase, Chem. Lett., 1986, 879. CrossRef
38. J. D. Panarese and S. P. Waters, Org. Lett., 2009, 11, 5086. CrossRef
39. S. Danishefsky, S. Kobayashi, and J. F. Kerwin, Jr., J. Org. Chem., 1982, 47, 1981. CrossRef
40. R. C. Larock and T. R. Hightower, J. Org. Chem., 1993, 58, 5298. CrossRef
41. (a) H. Fuwa, H. Yamaguchi, and M. Sasaki, Org. Lett., 2010, 12, 1848; CrossRef (b) H. Fuwa, H. Yamaguchi, and M. Sasaki, Tetrahedron, 2010, 66, 7492. CrossRef
42. C. R. Johnson and M. P. Braun, J. Am. Chem. Soc., 1993, 115, 11014. CrossRef
43. G. Sabitha, D. V. Reddy, A. S. Rao, and J. S. Yadav, Tetrahedron Lett., 2010, 51, 4195. CrossRef
44. L. Alcaraz, J. J. Harnett, C. Mioskowski, J. P. Martel, T. Le Gall, D.-S. Shin, and J. R. Falck, Tetrahedron Lett., 1994, 35, 5449. CrossRef
45. S. E. Schaus, B. D. Brandes, J. F. Larrow, M. Tokunaga, K. B. Hansen, A. E. Gould, M. E. Furrow, and E. N. Jacobsen, J. Am. Chem. Soc., 2002, 124, 1307. CrossRef
46. (a) N. Hori, H. Matsukura, and T. Nakata, Org. Lett., 1999, 1, 1099; CrossRef (b) N. Hori, H. Matsukura, G. Matsuo, and T. Nakata, Tetrahedron, 2002, 58, 1853. CrossRef
47. G. Sabitha, V. Bhaskar, K. Yadagiri, and J. S. Yadav, Synth. Commun., 2007, 37, 2491. CrossRef
48. I. K. Mangion and D. W. C. MacMillan, J. Am. Chem. Soc., 2005, 127, 3696. CrossRef
49. N. Momiyama and H. Yamamoto, J. Am. Chem. Soc., 2003, 125, 6038. CrossRef
50. A. J. M. Hendrix and M. P. Jennings, Tetrahedron Lett., 2010, 51, 4260. CrossRef
51. H. C. Brown, P. K. Jadhav, and K. S. Bhat, J. Am. Chem. Soc., 1988, 110, 1535. CrossRef
52. S. Hanessian, S. Giroux, and A. Larsson, Org. Lett., 2006, 8, 5481. CrossRef
53. Y. Izuchi, N. Kanomata, H. Koshino, Y. Hongo, T. Nakata, and S. Takahashi, Tetrahedron: Asymmetry, 2011, 22, 246. CrossRef
54. R. Tokunoh, H. Tomiyama, M. Sodeoka, and M. Shibasaki, Tetrahedron Lett., 1996, 37, 2449. CrossRef
55. K. Nagasawa, A. Georgieva, H. Takahashi, and T. Nakata, Tetrahedron, 2001, 57, 8959. CrossRef
56. E. J. Corey and C. J. Helal, Angew. Chem. Int. Ed., 1998, 37, 1986. CrossRef
57. M. Kanematsu, M. Yoshida, and K. Shishido, Angew. Chem. Int. Ed., 2011, 50, 2618. CrossRef
58. (a) M. Takeuchi, T. Taniguchi, and K. Ogasawara, Synthesis, 1999, 341; CrossRef (b) T. Yoshimura, F. Yakushiji, S. Kondo, X. Wu, M. Shindo, and K. Shishido, Org. Lett., 2006, 8, 475. CrossRef
59. I. Nakagawa, K. Aki, and T. Hata, J. Chem. Soc., Perkin Trans. 1, 1983, 1315. CrossRef
60. M. A. Blanchette, W. Choy, J. T. Davis, A. P. Essenfeld, S. Masamune, W. R. Roush, and T. Sakai, Tetrahedron Lett., 1984, 25, 2183. CrossRef
61. M. Kanematsu, M. Yoshida, and K. Shishido, Tetrahedron Lett., 2011, 52, 1372. CrossRef
62. H. Kobayashi, M. Kanematsu, M. Yoshida, and K. Shishido, Chem. Commun., 2011, 47, 7440. CrossRef
63. M. H. Nantz and P. L. Fuchs, J. Org. Chem., 1987, 52, 5298. CrossRef
64. W. Lin and C. K. Zercher, J. Org. Chem., 2007, 72, 4390. CrossRef
65. A. Zúñiga, M. Pérez, M. González, G. Gómez, and Y. Fall, Synthesis, 2011, 3301. CrossRef
66. P. Srihari and Y. Sridhar, Eur. J. Org. Chem., 2011, 6690. CrossRef
67. M. Kusakabe, Y. Kitano, Y. Kobayashi, and F. Sato, J. Org. Chem., 1989, 54, 2085. CrossRef
68. (a) M. Isobe, W. Phoosaha, R. Saeeng, K. Kira, and C. Yenjai, Org. Lett., 2003, 5, 4883; CrossRef (b) J. S. Yadav, A. K. Raju, and V. Sunitha, Tetrahedron Lett., 2006, 47, 5269. CrossRef
69. B. M. Trost, Z. T. Ball, and T. Jöge, J. Am. Chem. Soc., 2002, 124, 7922. CrossRef