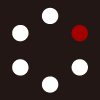
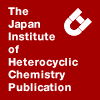
HETEROCYCLES
An International Journal for Reviews and Communications in Heterocyclic ChemistryWeb Edition ISSN: 1881-0942
Published online by The Japan Institute of Heterocyclic Chemistry
e-Journal
Full Text HTML
Received, 23rd January, 2012, Accepted, 2nd March, 2012, Published online, 14th March, 2012.
DOI: 10.3987/REV-12-730
■ Total Synthesis of Tetrahydropyran-Containing Natural Products Exploiting Intramolecular Oxa-Conjugate Cyclization
Haruhiko Fuwa*
Graduate School of Life Sciences, Tohoku University, 2-1-1, Katahira, Aoba-ku, Sendai, 980-8577, Japan
Abstract
A growing number of tetrahydropyran-containing biologically active substances are being discovered from nature. Intramolecular oxa-conjugate cyclization (IOCC) is known as one of the most powerful methodologies for the stereoselective synthesis of substituted tetrahydropyran derivatives, although there is clearly room for methodological improvement. In this review, we describe our successful total synthesis of tetrahydropyran-containing natural products by exploiting IOCC and the discoveries that we have made during the course of the synthetic campaigns.CONTENTS
1. Introduction
2. Total Synthesis of (+)-Neopeltolide
3. Total Synthesis of (−)-Aspergillides A and B
4. Total Synthesis of (−)-Exiguolide
5. Brønsted Acid-Catalyzed Intramolecular Oxa-Conjugate Cyclization of α,β-Unsaturated Ester Surrogates
6. Summary
1. INTRODUCTION
A growing number of tetrahydropyran-containing biologically active substances are being discovered from nature. Tetrahydropyran-containing natural products, isolated as the secondary metabolites of marine organisms, have attracted intense interest from chemists and biologists because of their extraordinarily complex molecular architecture and highly potent and characteristic biological activities.1,2 Two notable examples are macrolides and polycyclic ethers. These natural products are considered to be promising leads for the development of innovative therapeutic agents and/or useful molecular probes for elucidating complex biological phenomena at the molecular level. For example, halichondrin B, isolated from the marine sponge Halichondria okadaii by the Uemura/Hirata group,3 exhibits remarkably potent antitumor activity against human cancer cell lines as a cell cycle inhibitor that induces G2/M arrest and disrupts mitotic spindle organization4 (Figure 1). This natural product has successfully served as a lead compound for the development of eribulin mesylate (Halaven®), an anticancer agent for the treatment of late-stage breast cancer, by Eisai Research Laboratories on the basis of the total synthesis by the Kishi group.5,6 Due to their molecular complexity and biological significance, tetrahydropyran-containing natural products have been rewarding target molecules for the synthetic community. It is noteworthy that the tetrahydropyrans found in natural products are, in many cases, substituted at the C2 and C6 positions with cis configuration.
Over the past three decades, organic chemists have made a concerted effort to develop versatile methodologies for the synthesis of 2,6-cis-substituted tetrahydropyran derivatives,7 e.g., intramolecular oxa-conjugate cyclization (IOCC),8-12 Prins-type cyclization,13 modified Maitland—Japp reaction,14 intramolecular Pd(II)-catalyzed alkoxycarbonylation,15 and intramolecular Pd(II)-catalyzed SN2’ cyclization.16,17 Among these methodologies, IOCC of α,β-unsaturated ketones and esters has frequently been exploited for the total synthesis of complex tetrahydropyran-containing natural products.9-11 This is at least partly due to the synthetic versatility of the product tetrahydropyran that harbors carbonylmethyl functionality. Organocatalytic asymmetric IOCC reactions for the synthesis of tetrahydropyrans, flavones, and chromenes have also recently been reported.12
The mechanistic aspects of Brønsted base-catalyzed IOCC of α,β-unsaturated carbonyl compounds have been investigated independently by Martín, Banwell, and Schneider.18 It has been established that, in general, IOCC of α,β-unsaturated ketones and esters proceeds by the action of an appropriate base, such as NaH or KOt-Bu, to give 2,6-trans-substituted tetrahydropyrans under kinetic conditions (Scheme 1A).19 The corresponding 2,6-cis isomers would only be available via isomerization that involves a retro-IOCC/recyclization sequence under thermodynamic conditions. Accordingly, the stereoselectivity of IOCC of α,β-unsaturated ketones and esters may depend on their local structure and reaction conditions. Indeed, stereocontrolled synthesis of 2,6-cis-substituted tetrahydropyran derivatives has proved to be very difficult in some instances, even under thermodynamic conditions (vide infra).20 It seems this is particularly true for IOCC of α,β-unsaturated esters, possibly because of the low acidity of the α-protons of the ester carbonyl group. Meanwhile, it has also been shown that α,β-unsaturated ketones, but not esters, participate in IOCC under the influence of a Brønsted acid to provide 2,6-cis-substituted tetrahydropyran derivatives (Schemes 1B and 1C).21,22 Unfortunately, the mechanistic aspects of Brønsted acid-catalyzed IOCC of α,β-unsaturated carbonyl compounds have remained underexplored.
It has recently been reported that the tetrahydropyran substructures embedded in several polyketide natural products, including pederin, ambruticin, bryostatins, jerangolid, and sorangicin, are formed through IOCC catalyzed by “pyran synthase” during their biosynthesis.23 It is likely that biosynthetic IOCC involves carbonyl activation of α,β-unsaturated thioesters bound to acyl carrier proteins (ACPs) by H-bonding(s), thereby promoting intramolecular conjugate addition of the pendant hydroxy group to the conjugate acceptor (Scheme 2). The biosynthetic formation of tetrahydropyrans would be, at least in part, facilitated by enhanced electrophilicity of α,β-unsaturated thioesters when compared with the corresponding oxoesters.24 Thus, IOCC of α,β-unsaturated thioesters based on carbonyl activation would represent a “biomimetic” approach toward the synthesis of tetrahydropyran derivatives.
In recent years, there have been increasing interests in application of IOCC to stereoselective synthesis of complex tetrahydropyran derivatives. Roush and co-workers have described the synthesis of multi-substituted tetrahydropyran derivatives in their total synthesis of spongistatin 1 (Scheme 3).20e They initially found that oxidative cleavage of the double bond of 1 followed by Horner–Wadsworth–Emmons (HWE) olefination gave 2,6-trans-substituted tetrahydropyran trans-2 with 10:1 diastereoselectivity. The latter process involved migration of the TES group and concomitant IOCC to forge the tetrahydropyran ring. Unfortunately, all attempts at isomerization of trans-2 to thermodynamically more stable 2,6-cis-substituted tetrahydropyran cis-2 were unsuccessful. However, they found that the isomerization could be achieved after removal of the TES group. Thus, treatment of trans-3 with DBU in DMF at 80 °C led to cis-3 in 56% yield with 9:1 diastereoselectivity.
Forsyth et al. have synthesized the 2,6-cis-substituted tetrahydropyran subunit of phorboxazole A via IOCC under basic conditions (Scheme 4).20a Treatment of α,β-unsaturated ester 4 with TBAF•H2O in THF at room temperature for 12 h delivered two cyclization products trans-5 and cis-5, the former being favored under these conditions (75% combined yield, trans/cis = 7:1). Although they found that cis-5 could be obtained as the major diastereomer by extending the reaction time, the product yield declined to 29% as saponification of the ester and epimerization of the C23 stereogenic center occurred as side reactions. Exposure of trans-5 to NaH in the presence of methyl pivaolate (THF, room temperature, 5 h) resulted in epimerization to give cis-5 in 56% yield with 9:1 diastereoselectivity. However, epimerization at the C23 stereogenic center was still observed as a side reaction. They eventually developed a four-step sequence for the synthesis of cis-6 from 4. Thus, removal of the silyl groups with TBAF under low temperature conditions followed by treatment with NaH gave 2,6-trans-substituted tetrahydropyran trans-6. After silylation of the free hydroxy group, the resultant product was exposed to KH (THF, 0 °C, 1 h) to achieve isomerization to thermodynamically more stable 2,6-cis-substituted tetrahydropyran cis-6 (60% overall yield from 4).
Bates and Song have reported a stereoselective synthesis of the tetrahydropyran subunit of clavosolide A (Scheme 5).20b They initially investigated IOCC of α,β-unsaturated ester 7 in the presence of a base. However, upon treatment of 7 with NaOMe, the cyclization product 8 was obtained with a rather disappointing diastereoselectivity (yield not given). Changing the base to KOt-Bu resulted in material decomposition. Treatment of 7 with acidic methanol did not induce IOCC at all, presumably due to the low reactivity of the α,β-unsaturated ester. Accordingly, they resorted to more reactive α,β-unsaturated ketone 9, which upon exposure to Amberlyst 15 resin in methanol underwent clean desilylation and concomitant IOCC to deliver 2,6-cis-substituted tetrahydropyran 10 in 91% yield. This was successfully converted to ester 11 by periodic acid treatment in aqueous methanol.
The above examples highlight the feasibility of IOCC for the synthesis of complex tetrahydropyran derivatives. However, at the same time, it appears that the stereoselectivity of IOCC of α,β-unsaturated esters depends heavily on their structure and the reaction conditions employed. In addition, isomerization of 2,6-trans-substituted tetrahydropyrans to the corresponding 2,6-cis isomers may require the use of a strong base and/or harsh reaction conditions in some cases (e.g., trans-3 to cis-3, trans-6 to cis-6).
Tandem reactions involving IOCC provide an efficient means to synthesize complex tetrahydropyran derivatives. Evans and Andrews devised a tandem Mukaiyama aldol/Bi(NO3)3•5H2O-catalyzed IOCC reaction during the course of their total synthesis of (+)-leucascandrolide A (Scheme 6).21c Thus, treatment of acetate 12 with enol silane 13 in the presence of BF3•OEt2 followed by addition of a catalytic amount of Bi(NO3)3•5H2O provided ketone 15 in 78% yield with >19:1 diastereoselectivity. It can be envisaged that the initial Mukaiyama aldol reaction generated α,β-unsaturated ketone 14, and subsequent addition of Bi(NO3)3•5H2O effected IOCC with spontaneous loss of the silyl group. Notably, isomerization of 2,6-trans-substituted tetrahydropyran to thermodynamically more stable 2,6-cis isomer did not occur under the reaction conditions.
Hong et al. have synthesized the 2,6-cis-substituted tetrahydropyran embedded in (+)-neopeltolide based on their tandem allylic oxidation/IOCC reaction (Scheme 7).21b Exposure of allylic alcohol 16 to MnO2 gave α,β-unsaturated aldehyde 17, which underwent concomitant IOCC to deliver 2,6-cis-substituted tetrahydropyran 18. This was in situ oxidized to the corresponding ester 19. This methodology cleverly leverages the gem-disubstituent effect to realize exclusive formation of 2,6-cis-substituted tetrahydropyran 19.
In the following sections, we wish to describe our total synthesis of tetrahydropyran-containing natural products exploiting IOCC and illustrate what we discovered during these synthetic campaigns.
2. TOTAL SYNTHESIS OF (+)-NEOPELTOLIDE
(+)-Neopeltolide (20, Figure 2) was isolated from a deep-water sponge that belongs to the family Neopeltidae by Wright and co-workers.25 Its gross structure and relative configuration were proposed on the basis of extensive 2D NMR analysis. However, Panek and Scheidt later independently revised the assignment of the relative configuration at the C11 and C13 stereogenic centers and ultimately determined the complete stereostructure of this natural product through total synthesis.26 Wright et al. have shown that 20 exhibits highly potent antiproliferative activity against several cancer cell lines, including the P388 mouse leukemia, the A549 human lung adenocarcinoma, and the NCI-ADR-RES human ovarian carcinoma cell lines, with single-digit nanomolar concentrations. A flow cytometric analysis on the cell cycle progress of A549 cell lines revealed that 100 nM of 20 arrests the cell cycle at the G1 phase. In addition, 20 shows antifungal activity against the fungal pathogen Candida albicans. Kozmin and co-workers reported the cytochrome bc1 complex as the molecular target of 20 and inhibition of mitochondria ATP synthesis as the molecular basis of the potent biological activity.27 These structural and biological aspects of 20 make it an intriguing target molecule for the synthetic community. So far, nine total syntheses and five formal syntheses of 20 have been reported.28
We reported our first-generation total synthesis of 20 in 2008, which hinges on Suzuki–Miyaura coupling of an acetate-derived enol phosphate29,30 and subsequent ring-closing metathesis (RCM)31,32 to forge the 2,4,6-trisubstituted tetrahydropyran substructure.33 Unfortunately, our first-generation synthetic route was rather lengthy and not amenable to the development of a focused library of synthetic analogues to fully explore the structure–activity relationships of 20. Accordingly, we planned a second-generation synthetic approach toward 20 as shown in Scheme 8.11d As previously reported, we envisioned the oxazole-containing side chain 21 to be appended to the macrolactone core 22 at the final stage of the total synthesis via Mitsunobu esterfication.34 The most important feature of our second-generation synthetic plan was the formation of the 14-membered macrocycle by means of RCM of diene 23. Molecular modeling suggested that the Re face of the RCM product 22 would be more sterically encumbered than the Si face, implying that stereoselective hydrogenation of 22 would give 20 with the correct configuration at the C9 stereogenic center. The diene 23 would be available from alcohol 24 and carboxylic acid 25. We naturally thought that the latter fragment could be synthesized via IOCC of α,β-unsaturated ester 26 under thermodynamic control. We planned to obtain 26 from homoallylic alcohol 27 by means of chemoselective olefin cross-metathesis (CM),35 although we were fully aware that competitive RCM could occur as a side reaction.
The synthesis of carboxylic acid 25, summarized in Scheme 9, started with silylation of the known homoallylic alcohol 2836 to give silyl ether 29. We planned to exploit a bulky silyl group for the protection of the C7 hydroxy group, as it would be helpful for preventing competitive RCM during the projected CM of 27 with methyl acrylate.37 Selective one-pot dihydroxylation/periodate cleavage38 delivered aldehyde 30. Brown allylation of 30 using B-allyl-(+)-diisopinocampheylborane39 provided homoallylic alcohol 27 as an inseparable 10:1 mixture of diastereomers. Installation of the requisite α,β-unsaturated ester unit was best accomplished by CM of 27 with excess methyl acrylate under the influence of the Grubbs’ second-generation catalyst (G-II)40 (CH2Cl2, room temperature), which led to α,β-unsaturated ester 31 in 82% yield, along with cyclohexene derivative 32 in 7% yield. It was critically important to leave the C5 hydroxy group free, since this functionality would form an H-bonding with one of the two chlorine atoms of the catalyst and direct the intermediary ruthenium alkylidene complex A41 to undergo the desired CM pathway, leading to α,β-unsaturated ester 31. In contrast, the RCM product 32 would be formed via the ruthenacyclobutane B that suffers from unfavorable steric repulsions, or via the ruthenacyclobutane C by breaking the H-bonding. Accordingly, the H-bonding formed between the C5 hydroxy group and the chlorine atom of the catalyst would act as a conformational constraint and make the RCM energetically less favored than the CM.42 Protection of the C5 hydroxy group as its benzyloxymethyl (BOM) ether (BOMCl, i-Pr2NEt, n-Bu4NI) followed by cleavage of the silyl group (tetra-n-butylammonium fluoride (TBAF), AcOH) gave α,β-unsaturated ester 26 as the IOCC precursor. We have examined a variety of conditions and eventually found that exposure of 26 to excess DBU in toluene at 100 °C for two days resulted in stereoselective formation of the desired 2,6-cis-substituted tetrahydropyran 33 in 73% yield with more than 20:1 diastereoselectivity. Careful monitoring of the cyclization revealed that a mixture of the 2,6-cis isomer and the corresponding 2,6-trans isomer was initially formed with low stereoselectivity, and the proportion of the desired 2,6-cis isomer increased slowly as the reaction time progressed. This observation suggests that the isomerization of the kinetically formed 2,6-trans isomer to the thermodynamically more stable 2,6-cis isomer required unexpectedly harsh reaction conditions and prolonged reaction time. Finally, saponification of the ester group using potassium trimethylsilanoate (TMSOK)43 provided carboxylic acid 25.
The second-generation total synthesis of (+)-neopeltolide (20) was completed as illustrated in Scheme 10. Esterification of 25 with alcohol 24 under Yamaguchi conditions44 delivered diene 23. Although RCM of 23 turned out to be a nontrivial matter, we eventually found that the RCM could be efficiently performed using G-II catalyst in the presence of 1,4-benzoquinone45 in toluene at 100 °C, leading to (Z)-olefin 22 in 85% yield. Syringe pump addition of a toluene solution of the catalyst over a period of 6 hours was imperative for achieving a satisfactory conversion. As expected, hydrogenation of the C8–C9 double bond occurred from the less hindered Si face of the molecule with concomitant loss of the BOM group to afford the neopeltolide macrolactone 34. Finally, as previously reported,33 coupling of 34 with the known carboxylic acid 21 under Mitsunobu conditions (diisopropyl azodicarboxylate (DIAD), Ph3P) furnished (+)-neopeltolide (20). The spectroscopic properties and specific rotation value of our synthetic material were in complete agreement with those of the natural product. Furthermore, the synthetic 20 exhibited potent antiproliferative activity against mouse P388 leukemia cells, in accordance with the natural product. Our second-generation synthesis proceeded in 13 linear steps from commercially available trans-cinnamaldehyde and represents the shortest asymmetric synthesis of 20 reported so far.
3. TOTAL SYNTHESIS OF (−)-ASPERGILLIDES A AND B
In 2008, Kusumi and co-workers reported the isolation and structure determination of aspergillides A–C (35–37, Figure 3), tetrahydropyran-containing 14-membered macrolides.46 These naturally occurring substances were isolated from the marine-derived fungus Aspergillus ostianus strain 01F313, cultured in a bromine-modified medium, as the moderately cytotoxic constituents. Kusumi et al. initially assigned the stereostructure of 35–37 on the basis of extensive 2D NMR experiments coupled with modified Mosher analysis. A year later, Hande and Uenishi reported the first total synthesis of the proposed structure of (−)-aspergillide A.47 However, they found that the spectroscopic properties of their synthetic material were not in accordance with those of the authentic natural product but instead identical with those of natural (−)-aspergillide B. Thus, the original structural assignment of (−)-aspergillide A by the Kusumi group has to be reconsidered. Finally, Kusumi et al. have successfully determined the complete stereostructure of (−)-aspergillides A and B based on X-ray crystallographic analysis of the respective m-bromobenzoate.48 The unique stereostructure of aspergillides immediately spurred the interest of the synthetic community. Nagasawa and Kuwahara reported the first total synthesis of (−)-aspergillide A49 and (+)-aspergillide C,50 and since then a number of total and formal syntheses of aspergillides and their synthetic analogues have appeared.51 The recent total synthesis of aspergillides A–C by Shishido and co-workers,10a,g,h who exploited transannular IOCC for the construction of the 14-membered macrolactone framework, is of particular interest (Scheme 11).
Intrigued by the structural and biological aspects, we embarked on the total synthesis of (−)-aspergillides A and B.11e,f Since the sole structural difference between these two natural products is the configuration at the C3 stereogenic center,52 we envisioned a unified synthetic strategy that relies on (i) the construction of the 14-membered macrocyclic framework via Yamaguchi macrolactonization and (ii) stereodivergent synthesis of the 2,6-cis and 2,6-trans-substituted tetrahydropyrans (cis-42 and trans-42, respectively), from a common precursor, i.e., α,β-unsaturated ester 43, by means of IOCC (Scheme 12).
The synthesis of α,β-unsaturated ester 43 started with the known homoallylic alcohol 44 (Scheme 13).36 This material could be prepared from trans-cinnamaldehyde by using Maruoka asymmetric allylation36 or by enzymatic resolution of the corresponding racemic alcohol (Amano lipase AK, vinyl acetate, i-Pr2O, 40 °C, 47% (>99% ee by chiral HPLC analysis); then K2CO3, MeOH, 93%). Protection of 44 with TBSCl/imidazole gave silyl ether 45 quantitatively. Hydroboration of the terminal olefin followed by oxidative workup gave alcohol 46. Oxidation with 2,2,6,6-tetramethylpiperidin-1-oxyl (TEMPO) and PhI(OAc)253 and in situ Wittig homologation of the derived aldehyde provided α,β-unsaturated ester 47, which was reduced with DIBALH to afford allylic alcohol 48. Sharpless asymmetric epoxidation using diethyl (+)-tartrate ((+)-DET) gave epoxy alcohol 49 as a single stereoisomer. Iodination followed by zinc reduction delivered allylic alcohol 50. Upon treatment of 50 with G-II catalyst in the presence of methyl acrylate, CM proceeded cleanly to afford α,β-unsaturated ester 51 in 90% yield, with no trace amount of the corresponding RCM product. The preference for the CM pathway could be ascribed to the “H-bonding effect,” similar to that observed for homoallylic alcohol 27 (Scheme 9). Thus, an H-bonding formed between the allylic hydroxy group and the catalyst would lock the conformation of the ruthenium alkylidene intermediate so that it could not undergo RCM. Protection of the hydroxy group of 51 (MOMCl, i-Pr2NEt) followed by desilylation (TBAF, AcOH) afforded α,β-unsaturated ester 43.
With the requisite α,β-unsaturated ester 43 available, we investigated the stereodivergent synthesis of 2,6-cis and 2,6-trans-substituted tetrahydropyrans cis-42 and trans-42, respectively, by means of IOCC (Table 1). At first, IOCC of 43 under kinetic conditions was examined. As expected, treatment of 43 with KOt-Bu in THF at −78 °C for 30 min led to trans-42 in 96% yield with 17:1 diastereoselectivity (entry 1). However, what we soon recognized was that the stereoselective formation of cis-42 was not trivial.
Exposure of 43 to KOt-Bu (THF, 0 °C, 2 h) resulted in a 2.5:1 mixture of trans-42 and cis-42 (entry 2). Extending the reaction time and/or elevating the reaction temperature induced degradation of the material. Changing the base to NaH (THF, room temperature, 2 h) was ineffective for improvement of the stereochemical outcome (entry 3), and forcing the reaction conditions caused material decomposition. Treatment of 43 with a catalytic amount of DBU (CH2Cl2, room temperature, 99 h) also resulted in an unsatisfactory diastereoselectivity (entry 4). Unlike KOt-Bu or NaH, DBU was found to isomerize trans-42 to cis-42 under forcing conditions without material degradation. Thus, heating a toluene solution of 43 in the presence of excess DBU at 100 °C for 54 h led to a 7:1 mixture of cis-42 and trans-42 in 85% yield (entry 5). Finally, by elevating the reaction temperature to 135 °C, we could isolate an 11:1 mixture of cis-42 and trans-42 in 81% yield (entry 6). These diastereomers were separable by flash chromatography on silica gel. The relative stereochemistry of cis-42 and trans-42 was established by NOE experiments and 3JH,H analysis (Figure 4). Thus, we were able to synthesize cis-42 and trans-42 in a stereodivergent manner simply by switching the reaction conditions. However, at the same time, we learned that stereoselective synthesis of 2,6-cis-substituted tetrahydropyrans by means of IOCC might at least in some cases be quite difficult even under thermodynamic conditions, probably because the propensity of 2,6-trans-substituted tetrahydropyrans to isomerize to the corresponding 2,6-cis isomers depends on their local structure.
The total synthesis of (−)-aspergillide B (36) is depicted in Scheme 14. Ozonolysis of the styryl group of trans-42 followed by Takai olefination (CrCl2, CHI3, 1,4-dioxane/THF, room temperature)54 delivered (E)-vinyl iodide 52. Suzuki—Miyaura coupling of 52 with an alkylborane derived from olefin 5355 (aqueous Cs2CO3, PdCl2(dppf)•CH2Cl2, Ph3As, THF/DMF, room temperature)56 gave (E)-olefin 54. Hydrolysis of the methyl ester and benzoyl group afforded hydroxy acid 41. Yamaguchi macrolactonization under high-dilution conditions proceeded without incident to provide macrolactone 55 in 73% yield. Finally, cleavage of the MOM group (LiBF4, aqueous CH3CN, 72 °C) furnished (−)-aspergillide B (36). The spectroscopic data, including the 1H, 13C NMR, HRMS and specific rotation value, of the synthetic material were in full accordance with those of the authentic sample.
The total synthesis of (−)-aspergillide A (35) was performed in the same way as that described for (−)-aspergillide B (36) (Scheme 15). In this case, however, Yamaguchi macrolactonization to forge the 14-membered macrocyclic skeleton proved to be quite challenging, since the conformation of the tetrahydropyran ring must change from an energetically favored “all-equatorial” chair to an energetically disfavored “all-axial” chair during the formation of the ester linkage. We were able to isolate the 14-membered macrolactone 58 only in low yield. The MOM group was then removed to complete the total synthesis of (−)-aspergillide A (35). The synthetic material was identical to the natural product in all respects ([α]D, 1H, 13C NMR, HRMS).
4. TOTAL SYNTHESIS OF (−)-EXIGUOLIDE
The novel 20-membered macrolide (−)-exiguolide (59, Figure 5) was isolated from the methanol extract of the sponge Geodia exigua Thiele collected off Amami-Oshima by Ohta, Ikegami, and co-workers.57 The gross structure of 59 was determined by extensive 2D NMR analysis, and the relative configuration was established through conformational analysis mainly based on NOE correlations and 3JH,H values. The J-based configuration analysis (JBCA) method developed by Murata et al.58 was used to confirm the relative stereochemistry. The absolute configuration of 59 was finally determined by the total synthesis of (+)-exiguolide, the unnatural enantiomer, by Lee and co-workers.59 Ohta et al. have reported that (−)-exiguolide (59) specifically inhibits the fertilization of sea urchin (Hemicentrotus pulcherrimus) gametes at a concentration of 21 µM but not embryogenesis of the fertilized egg even at 100 µM. However, further detailed studies on the biological activity of 59 have been precluded due to its extremely limited availability in nature. The characteristic molecular structure reminiscent of potent antitumor agents bryostatins60,61 coupled with the natural scarcity heightened the interest of the synthetic community.10b,h,62
Our synthesis plan toward 59 is illustrated in Scheme 16. The triene side chain would be elaborated at the final stage of the total synthesis by means of Suzuki–Miyaura coupling of (Z)-vinyl boronate 60 and (E)-vinyl iodide 61.11b,h It was envisioned that the 20-membered macrolactone framework of 61 would be accessible from 63 through Julia–Kocienski olefination63 to forge the C16–C17 double bond followed by Yamaguchi macrolactonization. We planned to synthesize methylene bis(tetrahydropyran) 63 via reductive etherification64 of silyloxy ketone 64, which in turn would be available from α,β-unsaturated ketone 65 by means of IOCC under thermodynamic conditions. Finally, 65 should be obtainable from hydroxy olefin 66 and α,β-unsaturated ketone 67 through CM. It is noteworthy that our synthetic plan would enable a three-step access to the key synthetic intermediate methylene bis(tetrahydropyran) 63 from readily available acyclic fragments 66 and 67 by leveraging the high functional group tolerability and bond-forming ability of ruthenium-catalyzed olefin metathesis.
The synthesis of hydroxy olefin 66 commenced with protection of the known homoallylic alcohol 6865 as its MPM ether (MPMOC(=NH)CCl3, Sc(OTf)3)66 followed by oxidative cleavage of the double bond to deliver aldehyde 69 (Scheme 17). Diastereoselective allylation of 69 under chelate control (MgBr2•OEt2, allylSiMe3, CH2Cl2, 0 °C) provided homoallylic alcohol 70 as a single stereoisomer. Silylation (TIPSOTf, 2,6-lutidine) and deprotection of the MPM group using DDQ afforded hydroxy olefin 66.
The synthesis of α,β-unsaturated ketone 67 started with the known aldehyde 7167 (Scheme 18). Brown allylation of 71 using (+)-Ipc2Ballyl followed by CM with methyl acrylate in the presence of G-II gave α,β-unsaturated ester 72. Hydrogenation of the double bond, Weinreb amidation68 (MeONHMe•HCl, AlMe3), and silylation of the secondary hydroxy group (TESCl, Et3N, DMAP) provided amide 73. Finally, addition of vinyl lithium generated in situ from tetra(vinyl)stannane and methyl lithium afforded α,β-unsaturated ketone 67.69
Prior to coupling of 66 and 67, we examined CM of 66 with methyl vinyl ketone as a model reaction and encountered unexpected outcomes (Table 2). Upon treatment of a mixture of 66 and methyl vinyl ketone with 10 mol% of the Hoveyda–Grubbs second-generation catalyst (HG-II)70 in CH2Cl2 at 35 °C, α,β-unsaturated ketone 74 was isolated in 88% yield, as expected (entry 1). By contrast, when the reaction was carried out in 1,2-dichloroethane (DCE) at 80 °C, we were surprised to find that the only isolable product was 2,6-substituted tetrahydropyran 75 (80% yield, cis/trans 9:1, entry 2). We found that G-II catalyst is less effective for promoting the present reaction (entry 3). The best result was obtained by running the reaction in CH2Cl2 at 100 °C under microwave (MW) irradiation (94% yield, cis/trans 7:1, entry 4). These results indicated that domino CM/IOCC occurred under elevated temperature conditions, although the actual species responsible for the IOCC step remained unclear.
We have also studied IOCC of α,β-unsaturated ketone 74 under a variety of conditions (Table 3). Treatment of 74 with NaH (1.5 equiv) in THF at room temperature for 1 h gave 2,6-trans-substituted tetrahydropyran 75 as the major diastereomer with moderate diastereoselectivity (cis/trans 1:2, 89% combined yield, entry 1). Changing the base to KOt-Bu (0.2 equiv) and running the reaction in THF at 0 °C for 15 min resulted in isolation of 2,6-cis-substituted tetrahydropyran 75 in 72% yield with good diastereoselectivity (cis/trans 6:1, entry 2). The stereochemical outcome could be further improved simply by extending the reaction time so as to allow the reaction to reach thermodynamic equilibrium (cis/trans >20:1, 88% yield, entry 3). Since it is known that α,β-unsaturated ketones participate in Brønsted acid-catalyzed IOCC, we have also examined IOCC of 74 under acidic conditions: Exposure of 74 to p-TsOH•H2O (0.1 equiv) in CH2Cl2 at room temperature for 2 h delivered 2,6-cis-substituted tetrahydropyran 75 in 89% yield with greater than 20:1 diastereoselectivity (entry 4). This result and the previous studies demonstrate that Brønsted acid-catalyzed IOCC of α,β-unsaturated ketones generally provides 2,6-cis-substituted tetrahydropyrans with excellent diastereoselectivity. However, it remains elusive whether the stereoselectivity is kinetically or thermodynamically controlled.
Having gathered preliminary information on CM and IOCC, we proceeded to the synthesis of methylene bis(tetrahydropyran) 63 as summarized in Scheme 19. Treatment of a mixture of 66 and 67 with HG-II (10 mol%) in CH2Cl2 at 35 °C for 21 h led to α,β-unsaturated ketone 65 in 93% yield with excellent E/Z selectivity. Exposure of 65 to KOt-Bu (20 mol%) in THF at 0 °C for 1 h afforded thermodynamically favored silyloxy ketone 64 in 95% yield as a single stereoisomer. Finally, direct reductive etherification of 64 (BF3•OEt2, 1:5 Et3SiH/CH2Cl2, −60 to −25 °C, 45 min) furnished 63 in near quantitative yield with good stereoselectivity (d.r. 10:1). More importantly, 63 could be synthesized from 66 and 67 in one-pot by exploiting domino CM/IOCC that was discovered during the model experiments described above.
Thus, microwave irradiation of a mixture of 66, 67, and HG-II (10 mol%) in CH2Cl2 at 100 °C for 30 min cleanly generated silyloxy ketone 64, which without isolation was treated with BF3•OEt2/Et3SiH (CH2Cl2, −60 to −15 °C, 50 min) to afford 63 in 89% yield with approximately 10:1 diastereoselectivity at the C9 stereogenic center.
The synthesis of sulfone 62, delineated in Scheme 20, started with Sharpless asymmetric epoxidation of the known allylic alcohol 7671 to give epoxy alcohol 77 as a single stereoisomer. Chlorination72 followed by treatment with excess LDA according to the Takano procedure73 led to propargylic alcohol 78. Bromination of the terminal alkyne (NBS, AgNO3), palladium-catalyzed hydrostannylation (n-Bu3SnH, Pd(PPh3)4),74 and iododestannylation provided (E)-vinyl iodide 79. Protection of the secondary hydroxy group as its MPM ether and ensuing desilylation delivered alcohol 80, which was coupled with 1-phenyl-1H-tetrazole-5-thiol and oxidized with peroxide75 to afford the requisite sulfone 62.
As illustrated in Scheme 21, Julia–Kocienski olefination of aldehyde 81, derived from 63 in two steps, with an anion generated from sulfone 62 and LHMDS (4:1 THF/HMPA, −78 to 0 °C) to deliver (E)-olefin 82 in 63% yield (E/Z >20:1) along with recovered 81 (23%). Selective deprotection of the TBDPS group under basic conditions gave alcohol 83. Dess–Martin oxidation,76 Pinnick oxidation,77 and esterification led to ester 84. Cleavage of the MPM ether was best performed by treatment with BF3•OEt2/Et3SiH to provide alcohol 85. Saponification of the methyl ester followed by Yamaguchi macrolactonization of the derived seco-acid afforded 20-membered macrolactone 86 in 94% yield for the two steps. Although the macrolactonization necessitates the use of high-dilution conditions (final substrate concentration: 1 mM) to avoid dimerization, we could successfully synthesize more than 200 milligrams of 86.
he final stage of the total synthesis of (−)-exiguolide (59) is summarized in Scheme 22. Desilylation of 86 followed by oxidation provided ketone 87. HWE olefination using chiral phosphonate 88 developed by Fuji and co-workers78 afforded α,β-unsaturated ester 61 (Z/E = 5:1, 94% combined yield). The undesired (E)-isomer could be removed by flash chromatography on silica gel. Finally, Suzuki–Miyaura coupling of 61 with (Z)-vinylboronate 6079 (Pd2(dba)3, Ph3As, Ag2O, THF, room temperature)80 furnished (−)-exiguolide (59) in 73% yield. It was important to use moist THF as the solvent, since the coupling reaction did not proceed to an appreciable extent under strictly anhydrous conditions.81 The 1H, 13C NMR and HRMS spectra were in full accordance with those of the authentic sample. The specific rotation value of the synthetic material ([α]D24 −121.5 (c 0.22, CHCl3)) differed slightly from that of the natural product ([α]D25 −92.5 (c 0.069, CHCl3)) but matched well with that of the synthetic (+)-exiguolide ([α]D25 +119 (c 0.11, CHCl3)) except for the sign of the rotation. Thus, we have successfully completed the total synthesis of (−)-exiguolide, the natural enantiomer, for the first time. Notably, through our total synthesis, more than 40 milligrams of synthetic (−)-exiguolide was made available for detailed biological assessment.
We initially assessed the antiproliferative activity of 59 against a panel of 39 human cancer cell lines82,83 and found that this natural product inhibits the proliferation of NCI-H460 human lung large cell carcinoma, A549 human lung adenocarcinoma, SK-OV-3 human ovarian carcinoma, and MKN74 human gastric carcinoma cells, with GI50 values of 0.01, 0.65, 0.70, and 0.69 μM, respectively. Importantly, 59 may be cytostatic to these sensitive cell lines, since it did not completely abolish the cell viability even at higher concentrations (LC50 >100 µM). Furthermore, the biological mode-of-action of 59 was suggested to be unique and does not share similarity with that of more than 100 anticancer agents on the basis of the COMPARE analysis. These results strongly suggest that 59 is a novel anticancer agent with a unique mechanism that warrants further biological investigations.
5. BRØNSTED ACID-CATALYZED INTRAMOLECULAR OXA-CONJUGATE CYCLIZATION OF α,β-UNSATURATED ESTER SURROGATES
From our synthetic studies on (+)-neopeltolide, (−)-aspergillides A and B, and (−)-exiguolide, we have learned that Brønsted base-catalyzed IOCC of α,β-unsaturated ketones gives easy access to 2,6-cis-substituted tetrahydropyrans under thermodynamic conditions, while that of α,β-unsaturated esters requires, at least in some cases, harsh reaction conditions and extended reaction times for selective formation of 2,6-cis-substituted tetrahydropyrans. The observed reactivity difference can be ascribed to the fact that the α-hydrogen atoms of esters are less acidic than those of ketones. In addition, Brønsted acid-catalyzed IOCC of α,β-unsaturated ketones provides 2,6-cis-substituted tetrahydropyrans in a highly stereoselective manner, while that of less electrophilic α,β-unsaturated esters does not take place in general. In gathering all the available inforation, it occurred to us that it might be possible to synthesize 2,6-cis-substituted tetrahydropyrans by means of Brønsted acid-catalyzed IOCC of α,β-unsaturated ester surrogates with enhanced reactivity. Our idea was supported by recent biosynthetic studies which suggested that the tetrahydropyran subunits of several polyketide natural products are formed via IOCC of α,β-unsaturated thioesters promoted by carbonyl activation through H-bonding(s).23 Accordingly, we decided to investigate IOCC of α,β-unsaturated thioesters under acid catalysis (Scheme 23),11a,c although there have been only a handful of reports that describe the use of α,β-unsaturated thioesters as conjugate acceptors.84
We began our investigation by treating a series of α,β-unsaturated thioesters with various acids. Preliminary experiments showed that Brønsted acids such as CSA, p-TsOH, CH3SO3H, or TFA are able to promote the cyclization, whereas Lewis acids including MgBr2, InCl3, Sc(OTf)3, Zn(OTf)2, Cu(OTf)2 and Yb(OTf)3 are uniformly ineffective. Our observation is in accordance with that of Spencer et al., who reported that protons are the actual species responsible for Lewis acid-mediated hetero-Michael additions to α,β-unsaturated carbonyl compounds.85 Among the Brønsted acids examined, CSA was found to be the acid of choice. Thus, IOCC of a series of α,β-unsaturated thioesters, prepared by CM,86 was examined by using CSA as the catalyst (Table 4).87 In all cases, the reaction was performed in the presence of CSA (20 mol%) in CH2Cl2 at room temperature and stopped before full conversion, because partial loss of the MPM group was observed even under these mild conditions. From these experiments, we found that S-aryl thioesters 90b-d,f (entries 2—4, and 6) are more reactive than S-ethyl thioester 90a (entry 1), and S-(p-tolyl)thioester 90c turned out to be the most reactive of the thioesters examined (entry 3).
The scope of Brønsted acid-catalyzed IOCC of α,β-unsaturated thioesters was investigated as summarized in Table 5.87 A variety of substrates underwent smooth cyclization upon treatment with 20 mol% of CSA in DCE at 70 °C, giving the respective 2,6-cis-substituted tetrahydropyrans in excellent yields with high diastereoselectivity (d.r. from 14:1 to >20:1). The cyclization of δ-substituted α,β-unsaturated thioesters was found to be more facile than that of the non-substituted ones. One drawback of the present IOCC is the elevated temperature conditions required to complete the reaction within a reasonable reaction time. Unfortunately, it appears that acid-sensitive substrates are not compatible with these reaction conditions.
To overcome the shortcomings of Brønsted acid-catalyzed IOCC of α,β-unsaturated thioesters, we strove to discover more reactive α,β-unsaturated ester surrogates.11a We were particularly interested in α,β-unsaturated amides and imides because of their easy accessibility and convertibility.88 Thus, various substrates were efficiently prepared by means of CM (HG-II (5—10 mol%), CH2Cl2, 35 °C)89 and treated with CSA (20 mol%) in CH2Cl2 at room temperature (Table 6).
Not unexpectedly, pyrrolidyl amide 112a did not participate in IOCC because of its low reactivity toward conjugate additions (entry 1). On the other hand, imide 112b displayed high reactivity and gave 2,6-cis-substituted tetrahydropyran 113b in 94% yield with good diastereoselectivity (entry 2). This result demonstrated the importance of the carbonyl group of the pyrrolidine ring. A slight improvement of the diastereoselectivity was observed for IOCC of 2-oxazolidinone imide 112c (entry 3). The reaction of 112c proceeded more slowly than that of 112b but gave the corresponding 2,6-cis-substituted tetrahydropyran 113c in 94% yield with 13:1 diastereoselectivity. The above results suggested that the reactivity of α,β-unsaturated amides/imides toward IOCC might be inversely correlated with the electron-donating ability of the nitrogen atom, while maintaining sufficient Lewis basicity of the carbonyl oxygen and robustness of the C–N bond.90 Accordingly, we chose to examine α,β-unsaturated amides/imides of aromatic amines. IOCC of 2-benzoxazolidinone imide 112d gave 2,6-cis-substituted tetrahydropyran 113d as a single stereoisomer but unfortunately did not complete even after 40 h (entry 4). Indole amide 112e cyclized smoothly to provide 2,6-cis-substituted tetrahydropyran 113e in 90% yield with excellent diastereoselectivity (entry 5, d.r. 15:1), whereas indoline amide 112f was much less reactive than 112e and gave 113f in moderate yield (49%) after 25 h (entry 6). Finally, IOCC of 2,5-dimethylpyrrole amide 112g91 completed within 24 h and provided 2,6-cis-substituted tetrahydropyran 113g as a single stereoisomer in 90% yield (entry 7).
Taking the reactivity, stereoselectivity, and convertibility into consideration, the subsequent substrate scope experiments were carried out for α,β-unsaturated 2-oxazolidinone imides and 2,5-dimethylpyrrole amides.92
As summarized in Table 7, a series of α,β-unsaturated 2-oxazolidinone imides and 2,5-dimethylpyrrole amides were prepared from the corresponding olefins by means of CM (HG-II (10 mol%), CH2Cl2, 35 °C). Upon treatment of α,β-unsaturated 2-oxazolidinone imides with CSA (20 mol%) in CH2Cl2 at room temperature, the respective 2,6-cis-substituted tetrahydropyrans were obtained in high yields
with a synthetically useful level of diastereoselectivity (from 9:1 to >20:1). Moreover, Brønsted acid-catalyzed IOCC of α,β-unsaturated 2,5-dimethylpyrrole amides proceeded smoothly under the same reaction conditions to afford 2,6-cis-substituted tetrahydropyrans with excellent stereoselectivity (d.r. >20:1 in all but one case).
Thus, we have successfully demonstrated that the reactivity of α,β-unsaturated 2-oxazolidinone imides and 2,5-dimethylpyrrole amides toward Brønsted acid-catalyzed IOCC is much higher than that of the thioester counterparts and provides an efficient access to 2,6-cis-substitued tetrahydropyrans in a highly stereoselective manner.
We were intrigued with the mechanistic basis for the stereoselectivity of Brønsted acid-catalyzed IOCC of α,β-unsaturated ester surrogates. We first examined whether the stereoselectivity is kinetically or thermodynamically controlled (Scheme 24). Treatment of 2,6-trans-substituted tetrahydropyrans with CSA under the cyclization conditions did not induce isomerization at all, and only resulted in recovery of starting materials. These experiments have clearly shown that the stereochemical outcome of the Brønsted acid-catalyzed IOCC of α,β-unsaturated ester surrogates is kinetically controlled rather than as a consequence of thermodynamic equilibration.
Several arguments have been put forward in regard to the stereoselectivity of Brønsted base-catalyzed IOCC of α,β-unsaturated esters. Since Banwell et al. have shown that the olefin geometry of α,β-unsaturated esters has a profound influence on the stereochemical outcome of the cyclization,18a,d the following discussion will focus on commonly used (E)-α,β-unsaturated esters. Previous studies have shown that IOCC of α,β-unsaturated esters under basic conditions provides 2,6-trans-substituted tetrahydropyrans as the kinetic product.18a-e Martín and co-workers proposed, on the basis of theoretical and experimental studies, a chelate-controlled transition state model, where an alkali metal cation (e.g., Na+, K+) coordinates with the carbonyl oxygen atom and incoming alkoxide (Scheme 25A).18b,c In the transition state A (TS-A), the pro-equatorial α,β-unsaturated carbonyl moiety needs to be in energetically disfavored s-trans conformation93 to form the chelate complex, while in TS-B the pro-axial conjugate acceptor in s-cis conformation well accommodates the chelation. However, Yonemitsu et al. have shown that IOCC of α,β-unsaturated esters catalyzed by a quaternary ammonium base also leads to 2,6-trans-substituted tetrahydropyrans under kinetic control,18g indicating that the Martín’s chelate-controlled model does not fully account for the origin of the stereoselectivity. Schneider and Schuffenhauer have explained the stereochemical outcome of Brønsted base-catalyzed IOCC of α,β-unsaturated esters by a stereoelectronic effect.18e Semi-empirical calculations suggested that in TS-B the LUMO of the pro-axial conjugate acceptor would effectively overlap with the lone pair orbital of the incoming alkoxide with a nearly tetrahedral attack angle (the Bürgi–Dunitz trajectory),94 while the HOMO/LUMO interaction would be weaker in TS-A because the attack angle in TS-A is estimated to be smaller than that in TS-B.18b,c,e Thus, it appears that the stereoelectronic effect would primarily govern the stereoselectivity of Brønsted base-catalyzed IOCC of α,β-unsaturated esters.
We provided a model that accounts for the excellent diastereoselectivity observed for Brønsted acid-catalyzed IOCC of α,β-unsaturated ester surrogates on the basis of the frontier molecular orbital theory (Scheme 25B). Houk and Strozier have shown that protonation of acrolein renders the energy levels and coefficients of the frontier molecular orbitals “more like those of an allylic cation mixed with a lone-pair orbital of oxygen.”95 Jensen and co-workers have postulated that acid-catalyzed hydration of aliphatic α,β-unsaturated ketones proceeds via protonation of the carbonyl oxygen followed by addition of H2O to the resultant allylic cationic species.96 Inspired by these previous studies, we propose that Brønsted acid-catalyzed IOCC of α,β-unsaturated ester surrogates would also involve an allylic cationic transition structure and proceed via an SN1-like mechanism.97 Thus, we considered two chair-like transition structures, TS-C and TS-D, and assumed that the conformations of TS-C and TS-D would be basically similar to those of TS-A and TS-B, respectively. Taking the coefficients of the frontier molecular orbitals of allylic cations into account, the stabilizing HOMO/LUMO interaction in TS-C would be greater than that in TS-D. In addition, TS-D would suffer from unfavorable 1,3-diaxial steric repulsions. Accordingly, we concluded that TS-C would be energetically favored over TS-D by both stereoelectronic and steric effects. Our transition state model well explains the observed stereoselectivity of Brønsted acid-catalyzed IOCC of α,β-unsaturated ester surrogates.
On the basis of our NMR experiments, the reactivity order of α,β-unsaturated ester surrogates toward Brønsted acid-catalyzed IOCC was established to be: 2,5-dimethylpyrrole amides > 2-oxazolidinone imides > S-(p-tolyl)thioesters (data not shown). In contrast to α,β-unsaturated esters, these surrogates are reactive enough to undergo Brønsted acid-catalyzed IOCC.
The high reactivity of these surrogates could be explained by their propensity to form the corresponding allylic cationic species upon protonation of the carbonyl group. It is known that keto–enol tautomerization of aliphatic esters is not facile because the lone pair of the ester oxygen delocalizes to the carbonyl group and reduces the acidity of the α-hydrogens.98 When compared to aliphatic oxoesters, the corresponding thioesters, 2-oxazolidinone imides, and 2,5-dimethylpyrrole amides would be more prone to keto–enol tautomerization because of the low electron-donating ability of the sulfur or nitrogen atom as well as sufficient Lewis basicity of the carbonyl oxygen.98 On the basis of these arguments, it is conceivable that protonation of α,β-unsaturated ester surrogates would easily generate the corresponding allylic cationic species that react intramolecularly with the proximal hydroxy group, while it would be difficult to form such an allylic cationic species from less reactive α,β-unsaturated esters.
Lastly, we have confirmed that the cyclization products (e.g., 105, 113c, and 113g) could be converted to the corresponding aldehydes, amides, carboxylic acids, esters, and ketones by exploiting well-established methods.99-108
6. SUMMARY
We have completed total syntheses of oxacyclic natural products, (+)-neopeltolide,11d (−)-aspergillides A and B,11e,f (−)-exiguolide,11b,h and (±)-centrolobine,11i by exploiting IOCC for the stereoselective synthesis of their tetrahydropyran substructures. During these synthetic campaigns, we have learned that Brønsted base-catalyzed IOCC of α,β-unsaturated esters requires, at least in some cases, harsh reaction conditions and extended reaction times for stereoselective synthesis of 2,6-cis-substituted tetrahydropyrans. It appears that this shortcoming limits the utility of IOCC in the context of complex molecule synthesis. Accordingly, we strove for the development of new methodologies that enable the synthesis of 2,6-cis-substituted tetrahydropyrans in a highly stereoselective manner and eventually discovered two new processes: (1) a domino CM/IOCC reaction catalyzed by the HG-II complex11g and (2) Brønsted acid-catalyzed IOCC of α,β-unsaturated ester surrogates.11a,c The ready availability of precursors, high functional group compatibility, high diastereoselectivity, and operational simplicity are the hallmarks of our methodologies. Further studies on the application of our newly developed methodologies to the total synthesis of natural products are currently underway.
ACKNOWLEDGEMENTS
The author is grateful to Professor Makoto Sasaki (Graduate School of Life Sciences, Tohoku University) for his generous support throughout this work. The author thanks Hiroshi Yamaguchi, Asami Saito, Kenkichi Noto, and Naoki Ichinokawa (Graduate School of Life Sciences, Tohoku University) for their significant contributions to the work summarized in this review. The author appreciates Professor Keiichi Konoki and Professor Mari Yotsu-Yamashita (Graduate School of Agricultural Science, Tohoku University) for evaluating the antiproliferative activity of synthetic (+)-neopeltolide and its analogues. Professor Hiroshi Kubo (Tohoku University Graduate School of Medicine), Dr. Takaya Suzuki (Institute of Development, Aging and Cancer, Tohoku University), and Dr. Takao Yamori (Cancer Chemotherapy Center, The Japanese Foundation for Cancer Research) are gratefully acknowledged for their assessment of the antiproliferative activity of synthetic (−)-exiguolide and its analogues. The author thanks Professor Shigefumi Kuwahara (Graduate School of Agricultural Science, Tohoku University) and Professor Takenori Kusumi (Department of Chemistry, Tokyo Institute of Technology) for the copies of the 1H and 13C NMR spectra of authentic (−)-aspergillides A and B. The author is thankful to Professor Shinji Ohta (Nagahama Institute for Bio-science and Technology) for providing the copies of the 1H and 13C NMR spectra of natural (−)-exiguolide and for fruitful discussions on the spectroscopic data. This work was supported in part by a Grant-in-Aid for Young Scientists (A) from the Japan Society for Promotion of Science (JSPS).
References
1. For recent reviews of marine natural products, see: (a) O. K. Radjasa, Y. M. Vaske, G. Navarro, H. C. Vervoort, K. Tenney, R. G. Linington, and P. Crews, Bioorg. Med. Chem., 2011, 19, 6658; CrossRef (b) R. Montaser and H. Luesch, Future Med. Chem., 2011, 3, 1475; CrossRef (c) J. W. Blunt, B. R. Copp, M. H. G. Munro, P. T. Northcote, and M. R. Prinsep, Nat. Prod. Rep., 2011, 28, 196; CrossRef (d) M. Kita, O. Ohno, C. Han, and D. Uemura, Chem. Rec., 2010, 10, 57; CrossRef (e) T. F. Molinski, D. S. Dalisay, S. L. Lievens, and J. P. Saludes, Nature Rev. Drug Discov., 2009, 8, 69; CrossRef (f) K. Nakamura, M. Kitamura, and D. Uemura, Heterocycles, 2009, 78, 1 and references cited therein. CrossRef
2. For recent reviews of the synthetic aspects of marine natural products, see: (a) J. C. Morris and A. J. Phillips, Nat. Prod. Rep., 2011, 28, 269; CrossRef (b) K.-S. Yeung and I. Paterson, Chem. Rev., 2005, 105, 4237. CrossRef
3. (a) D. Uemura, K. Takahashi, T. Yamamoto, C. Katayama, J. Tanaka, Y. Okumura, and Y. Hirata, J. Am. Chem. Soc., 1985, 107, 4796; CrossRef (b) Y. Hirata and D. Uemura, Pure Appl. Chem., 1986, 58, 701. CrossRef
4. (a) R. Bai, K. D. Paull, C. L. Herald, L. Malspeis, G. R. Pettit, and E. Hamel, J. Biol. Chem., 1991, 266, 15882; (b) R. F. Luduena, M. C. Roach, V. Prasad, and G. R. Pettit, Biochem. Pharmacol., 1993, 45, 421. CrossRef
5. (a) T. D. Aicher, K. R. Buszek, F. G. Fang, C. J. Forsyth, S. H. Jung, Y. Kishi, M. C. Matelich, P. M. Scola, D. M. Spero, and S. K. Yoon, J. Am. Chem. Soc., 1992, 114, 3162; CrossRef (b) C.-G. Dong, J. A. Henderson, Y. Kaburagi, T. Sasaki, D.-S. Kim, J. T. Kim, D. Urabe, H. Guo, and Y. Kishi, J. Am. Chem. Soc., 2009, 131, 15642; CrossRef (c) D.-S. Kim, C-.G. Dong, J. T. Kim, H. Guo, J. Huang, P. S. Tiseni, and Y. Kishi, J. Am. Chem. Soc., 2009, 131, 15636. CrossRef
6. (a) M. J. Towle, K. A. Salvato, J. Budrow, B. F. Wels, G. Kuznetsov, K. K. Aalfs, S. Welsh, W. Zheng, B. M. Seletsky, M. H. Palme, G. J. Habgood, L. A. Singer, L. V. DiPietro, Y. Wang, J. J. Chen, D. A. Quincy, A. Davis, K. Yoshimatsu, Y. Kishi, M. J. Yu, and B. A. Littlefield, Cancer Res., 2001, 61, 1013; (b) G. Kuznetsov, M. J. Towle, H. Cheng, T. Kawamura, K. TenDyke, D. Liu, Y. Kishi, M. J. Yu, and B. A. Littlefield, Cancer Res., 2004, 64, 5760; CrossRef (c) M. A. Jordan, K. Kamath, T. Manna, T. Okouneva, H. P. Miller, C. Davis, B. A. Littlefield, and L. Wilson, Mol. Cancer Ther., 2005, 4, 1086; CrossRef (d) D. A. Dabydeen, J. C. Burnett, R. Bai, P. Verdier-Pinard, S. J. H. Hickford, G. R. Pettit, J. W. Blunt, M. H. G. Munro, R. Gussio, and E. Hamel, Mol. Pharmacol., 2006, 70, 1866. CrossRef
7. For reviews of tetrahydropyran synthesis, see: (a) I. Larossa, P. Romea, and F. Urpí, Tetrahedron, 2008, 64, 2683; CrossRef (b) P. A. Clarke and S. Santos, Eur. J. Org. Chem., 2006, 2045. CrossRef
8. For reviews of oxa-conjugate addition, see: (a) C. F. Nising and S. Bräse, Chem. Soc. Rev., 2008, 37, 1218; CrossRef (b) C. F. Nising and S. Bräse, Chem. Soc. Rev., 2012, 41, 988. CrossRef
9. For pioneering studies of IOCC, see: (a) B. Maurer, A. Grieder, and W. Thommen, Helv. Chim. Acta, 1979, 62, 44; CrossRef (b) D. Seebach and M. Pohmakotr, Helv. Chim. Acta, 1979, 62, 843; CrossRef (c) A. P. Kozikowski, R. J. Schmiesing and K. L. Sorgi, J. Am. Chem. Soc., 1980, 102, 6577; CrossRef (d) R. D. Dawe and B. Fraser-Reid, J. Org. Chem., 1984, 49, 522; CrossRef (e) A. Giannis and K. Sandhoff, Carbohydr. Res., 1987, 171, 201; CrossRef (f) T. D. Aicher and Y. Kishi, Tetrahedron Lett., 1987, 28, 3463; CrossRef (g) P. Allevi, P. Ciuffreda, D. Colombo, D. Monti, G. Speranza, and P. Manitto, J. Chem. Soc., Perkin Trans. 1, 1989, 1281. CrossRef
10. For recent examples, see: (a) H. Kobayashi, M. Kanematsu, M. Yoshida, and K. Shishido, Chem. Commun., 2011, 47, 7440; CrossRef (b) M. Pellicena, K. Krämer, P. Romea, and F. Urpí, Org. Lett., 2011, 13, 5350; CrossRef (c) J. S. Yadav and G. Rajendar, Eur. J. Org. Chem., 2011, 6781; CrossRef (d) J. Robertson, C. North, and J. E. R. Sadig, Tetrahedron, 2011, 67, 5011; CrossRef (e) D. Gill, N. H. Taylor, E. J. Thomas, Tetrahedron, 2011, 67, 5034; CrossRef (f) F. Hilli, J. M. White, and M. A. Rizzacasa, Tetrahedron, 2011, 67, 5054; CrossRef (g) M. Kanematsu, M. Yoshida, and K. Shishido, Angew. Chem. Int. Ed., 2011, 50, 2618;; CrossRef (h) M. Kanematsu, M. Yoshida, and K. Shishido, Tetrahedron Lett., 2011, 52, 1372; CrossRef (i) H. Park, H. Kim, and J. Hong, Org. Lett., 2011, 13, 3742; CrossRef (j) K. Lee, H. Kim, and J. Hong, Org. Lett., 2011, 13, 2722; CrossRef (k) M. Wohland and M. E. Maier, Synlett, 2011, 1523; CrossRef (l) S. Pabbaraja, K. Satyanarayana, B. Ganganna, and J. S. Yadav, J. Org. Chem., 2011, 76, 1922; CrossRef (m) C. R. Reddy and B. Srikanth, Synlett, 2010, 1536; CrossRef (n) L. Wang, P. Li, and D. Menche, Angew. Chem. Int. Ed., 2010, 49, 9270; CrossRef (o) H. Kim and J. Hong, Org. Lett., 2010, 12, 2880; CrossRef (p) Z. E. Wilson and M. A. Brimble, Org. Biomol. Chem., 2010, 8, 1284; CrossRef (q) M.-A. Hiebel, B. Pelotier, and O. Piva, Tetrahedron Lett., 2010, 51, 5091; CrossRef (r) H. Kim, Y. Park, and J. Hong, Angew. Chem. Int. Ed., 2009, 48, 7577; CrossRef (s) F. Yakushiji, J. Maddaluno, M. Yoshida, and K. Shishido, Tetrahedron Lett., 2009, 50, 1504; CrossRef (t) H. Lee, K. W. Kim, J. Park, H. Kim, S. Kim, D. Kim, X. Hu, W. Yang, and J. Hong, Angew. Chem. Int. Ed., 2008, 47, 4200; CrossRef (u) H. H. Jung and P. E. Floreancig, J. Org. Chem., 2007, 72, 7359 and references cited therein. See also refs. 19-22. CrossRef
11. For recent reports from our group, see: (a) H. Fuwa, N. Ichinokawa, K. Noto, and M. Sasaki, J. Org. Chem., in press. DOI: 10.1021/jo202179s; CrossRef (b) H. Fuwa, T. Suzuki, H. Kubo, T. Yamori, and M. Sasaki, Chem. Eur. J., 2011, 17, 2678; CrossRef (c) H. Fuwa, K. Noto, and M. Sasaki, Org. Lett., 2011, 13, 1820; CrossRef (d) H. Fuwa, A. Saito, and M. Sasaki, Angew. Chem. Int. Ed., 2010, 49, 3041; CrossRef (e) H. Fuwa, H. Yamaguchi, and M. Sasaki, Tetrahedron, 2010, 66, 7492; CrossRef (f) H. Fuwa, H. Yamaguchi, and M. Sasaki, Org. Lett., 2010, 12, 1848; CrossRef (g) H. Fuwa, K. Noto, and M. Sasaki, Org. Lett., 2010, 12, 1636; CrossRef (h) H. Fuwa and M. Sasaki, Org. Lett., 2010, 12, 584; CrossRef (i) H. Fuwa, K. Noto, and M. Sasaki, Heterocycles, 2010, 82, 641. CrossRef
12. For recent examples of organocatalytic asymmetric IOCC for the synthesis of tetrahydropyran derivatives and related compounds, see: (a) S. R. Byeon, H. Park, H. Kim, and J. Hong, Org. Lett., 2011, 13, 5816; CrossRef (b) R. L. Farmer, M. M. Biddle, A. E. Nibbs, X. Huang, R. C. Bergan, and K. A. Scheidt, ACS Med. Chem. Lett., 2010, 1, 400; CrossRef (c) B.-C. Hong, P. Kotame, C.-W. Tsai, and J.-H. Liao, Org. Lett., 2010, 12, 776; CrossRef (d) D. Díez, M. G. Núñez, A. Benéitez, R. F. Moro, I. S. Marcos, P. Basabe, H. B. Broughton, and J. G. Urones, Synlett, 2009, 390; CrossRef (e) A. Massi, A. Nuzzi, and A. Dondoni, J. Org. Chem., 2007, 72, 10279; CrossRef For a review, see: M. G. Núñez, P. García, R. F. Moro, and D. Díez, Tetrahedron, 2010, 66, 2089. CrossRef
13. For selected recent examples of Prins-type cyclization for the synthesis of tetrahydropyran derivatives, see: (a) E. A. Crane, T. P. Zabawa, R. L. Farmer, and K. A. Scheidt, Angew. Chem. Int. Ed., 2011, 50, 9112; CrossRef (b) Y. Lu, S. K. Woo, and M. J. Krische, J. Am. Chem. Soc., 2011, 133, 13876; CrossRef (c) M. R. Gesinski and S. D. Rychnovsky, J. Am. Chem. Soc., 2011, 133, 9727; CrossRef (d) P. A. Wender and A. J. Schrier, J. Am. Chem. Soc., 2011, 133, 9228; CrossRef (e) G. E. Keck, Y. B. Poudel, T. J. Cummins, A. Rudra, and J. A. Covel, J. Am. Chem. Soc., 2011, 133, 744; CrossRef (f) S. Bondalapati, U. C. Reddy, P. Saha, and A. K. Saikia, Org. Biomol. Chem., 2011, 9, 3428; CrossRef (g) J. S. Yadav, N. Thrimurtulu, M. A. Rahman, J. S. Reddy, A. R. Prasad, and B. V. S. Reddy, Synthesis, 2010, 3657; CrossRef (h) S. K. Woo and E. Lee, J. Am. Chem. Soc., 2010, 132, 4564; CrossRef (i) H. Zhou and T.-P. Loh, Tetrahedron Lett., 2009, 50, 4368; CrossRef and references cited therein. For reviews, see: (j) E. A. Crane and K. A. Scheidt, Angew. Chem. Int. Ed., 2010, 49, 8316; CrossRef (k) C. Olier, M. Kaafarani, S. Gastaldi, and M. P. Bertrand, Tetrahedron, 2010, 66, 413. CrossRef
14. For recent examples of the modified Maitland—Japp reaction for the synthesis of tetrahydropyran derivatives, see: (a) J. M. Tenenbaum, W. J. Morris, D. W. Custar, and K. A. Scheidt, Angew. Chem. Int. Ed., 2011, 50, 5892; CrossRef (b) S. Huang, G. Du, and C-.S. Lee, J. Org. Chem., 2011, 76, 6534; CrossRef (c) M. Iqbal, N. Mistry, and P. A. Clarke, Tetrahedron, 2011, 67, 4960 and references cited therein. CrossRef
15. For recent examples of palladium-catalyzed intramolecular alkoxycarbonylation for the synthesis of tetrahydropyran derivatives, see: (a) Z. Yang, B. Zhang, G. Zhao, J. Yang, X. Xie, and X. She, Org. Lett., 2011, 13, 5916; CrossRef (b) O. Karlubíková, M. Babjak, and T. Gracza, Tetrahedron, 2011, 67, 4980; CrossRef (c) Z. Yang, X. Xie, P. Jing, G. Zhao, J. Zheng, C. Zhao, and X. She, Org. Biomol. Chem., 2011, 9, 984; CrossRef (d) J. D. White, P. Kuntiyong, and T. H. Lee, Org. Lett., 2006, 8, 6039 and references cited therein. CrossRef
16. For recent examples of palladium-catalyzed intramolecular SN2’ cyclization for the synthesis of tetrahydropyran derivatives, see: (a) S. S. Palimkar and J. Uenishi, Org. Lett., 2010, 12, 4160; CrossRef (b) S. Hanessian, T. Focken, and R. Oza, Org. Lett., 2010, 12, 3172; CrossRef (c) J. Uenishi and Y. S. Vikhe, Heterocycles, 2010, 80, 1463; CrossRef (d) A. He, N. Sutivisedsak, and C. D. Spilling, Org. Lett., 2009, 11, 3124; CrossRef (e) J. Uenishi, Y. S. Vikhe, and N. Kawai, Chem. Asian J., 2008, 3, 473; CrossRef (f) M. Reiter, H. Turner, and V. Gouverneur, Chem. Eur. J., 2006, 12, 7190 and references cited therein. CrossRef
17. Other selected recent methodologies for the synthesis of tetrahydropyran derivatives: FeCl3·6H2O-catalyzed cyclization: (a) A. Guérinot, A. Serra-Muns, C. Bensoussan, S. Reymond, and J. Cossy, Tetrahedron, 2011, 67, 5024; CrossRef Mukaiyama—Michael addition: (b) S.-S. Chua, A. Alni, L.-T. J. Chan, M. Yamane, and T.-P. Loh, Tetrahedron, 2011, 67, 5079; CrossRef Multicomponent asymmetric Henry/Michael reaction/acetalization/Lewis-acid catalyzed allylation reaction: (c) H. Ishikawa, S. Sawano, Y. Yasui, Y. Shibata, and Y. Hayashi, Angew. Chem. Int. Ed., 2011, 50, 3774; CrossRef Oxidative C—H activation: (d) W. Tu and P. E. Floreancig, Angew. Chem. Int. Ed., 2009, 48, 4567; CrossRef (e) W. Tu, L. Liu, and P. E. Floreancig, Angew. Chem. Int. Ed., 2008, 47, 4184; CrossRef One-pot hetero-Diels—Alder/Mukaiyama—Michael reaction: (f) M. Anada, T. Washio, Y. Watanabe, K. Takeda, and S. Hashimoto, Eur. J. Org. Chem., 2010, 6850; CrossRef Oxidative cyclization via quinone methides: (g) F. Rogano and P. Rüedi, Helv. Chim. Acta, 2010, 93, 1281; CrossRef Tandem Mukaiyama aldol/lactonization: (h) T. A. Mitchell, C. Zhao, and D. Romo, J. Org. Chem., 2008, 73, 9544; CrossRef Tandem olefin cross-metathesis/intramolecular SN2’ cyclization: (i) K. Lee, H. Kim, and J. Hong, Org. Lett., 2009, 11, 5202. CrossRef
18. For discussions on the stereochemical outcome of IOCC, see: (a) M. G. Banwell, C. T. Bui, H. T. T. Pham, and G. W. Simpson, J. Chem. Soc., Perkin Trans. 1, 1996, 967; CrossRef (b) J. M. Betancort, V. S. Martín, J. M. Padrón, J. M. Palazón, M. A. Ramírez, and M. A. Soler, J. Org. Chem., 1997, 62, 4570; CrossRef (c) M. A. Ramírez, J. M. Padrón, J. M. Palazón, and V. S. Martín, J. Org. Chem., 1997, 62, 4584; CrossRef (d) M. G. Banwell, B. D. Bissett, C. T. Bui, G. W. Simpson, and H. T. T. Pham, Aust. J. Chem., 1998, 51, 9; CrossRef (e) C. Schneider and A. Schuffenhauer, Eur. J. Org. Chem., 2000, 73; CrossRef See also: (f) B. W. Gung and M. B. Francis, J. Org. Chem., 1993, 58, 6177; CrossRef (g) O. Yonemitsu, T. Yamazaki, and J. Uenishi, Heterocycles, 1998, 49, 89; CrossRef (h) L. Vares and T. Rein, J. Org. Chem., 2002, 67, 7226; CrossRef (i) D. Strand, P.-O. Norrby, and T. Rein, J. Org. Chem., 2006, 71, 1879; CrossRef (j) V. Aucagne, A. Tatibouët, and P. Rollin, Tetrahedron Lett., 2008, 49, 4750. CrossRef
19. It has been known that IOCC of α,β-unsaturated esters having a pro-axial δ-substituent leads to 2,6-cis-substituted tetrahydropyrans under kinetic conditions. See: (a) G. Pattenden, M. A. González, P. B. Little, D. S. Millan, A. T. Plowright, J. A. Tornos, and T. Ye, Org. Biomol. Chem., 2003, 1, 4173; CrossRef (b) M. T. Crimmins and P. Siliphaivanh, Org. Lett., 2003, 5, 4641; CrossRef (c) H. Fuwa, N. Kainuma, K. Tachibana, and M. Sasaki, J. Am. Chem. Soc., 2002, 124, 14983. CrossRef
20. For recent examples, see: (a) B. Wang, T. M. Hansen, T. Wang, D. Wu, L. Weyer, L. Ying, M. M. Engler, M. Sanville, C. Leitheiser, M. Christmann, Y. Lu, J. Chen, N. Zunker, R. D. Cink, F. Ahmed, C.-S. Lee, and C. J. Forsyth, J. Am. Chem. Soc., 2011, 133, 1484; CrossRef (b) R. W. Bates and P. Song, Synthesis, 2010, 2935; CrossRef (c) J. Uenishi, T. Iwamoto, and J. Tanaka, Org. Lett., 2009, 11, 3262; CrossRef (d) L. Ferrié, L. Boulard, F. Pradaux, S. Bouzbouz, S. Reymond, P. Capdevielle, and J. Cossy, J. Org. Chem., 2008, 73, 1864; CrossRef (e) G. C. Micalizio, A. N. Pinchuk, and W. R. Roush, J. Org. Chem., 2000, 65, 8730 and references cited therein. CrossRef
21. For recent examples, see: (a) C. R. Reddy and N. N. Rao, Tetrahedron Lett., 2010, 51, 5840; CrossRef (b) H. Kim, Y. Park, and J. Hong, Angew. Chem. Int. Ed., 2009, 48, 7577; CrossRef (c) P. A. Evans and W. J. Andrews, Angew. Chem. Int. Ed., 2008, 47, 5426; CrossRef (d) R. W. Bates and P. Song, Tetrahedron, 2007, 63, 4497; CrossRef (e) J. Liu, J. H. Yang, C. Ko, and R. P. Hsung, Tetrahedron Lett., 2006, 47, 6121; CrossRef (f) S. Chandrasekhar, S. J. Prakash, and T. Shaymsunder, Tetrahedron Lett., 2005, 46, 6651 and references cited therein. See also ref 20b. CrossRef
22. A single example of acid-catalyzed IOCC of an α,β-unsaturated ester, presumably promoted by the Thorpe—Ingold effect, has recently been reported: A. K. Hajare, V. Ravikumar, S. Khaleel, D. Bhuniya, and D. S. Reddy, J. Org. Chem., 2011, 76, 963. CrossRef
23. (a) H. Irschik, M. Kopp, K. J. Weissman, K. Buntin, J. Piel, and R. Müller, ChemBioChem, 2010, 11, 1840; CrossRef (b) S. Sudek, N. B. Lopanik, L. E. Waggoner, M. Hildebrand, C. Anderson, H. Liu, A. Patel, D. H. Sherman, and M. G. Haygood, J. Nat. Prod., 2007, 70, 67; CrossRef (c) B. Julien, Z.-Q. Tian, R. Reid, and C. D. Reeves, Chem. Biol., 2006, 13, 1277; CrossRef (d) J. Piel, Proc. Natl. Acad. Sci. U.S.A., 2002, 99, 14002. CrossRef
24. (a) M. W. Cronyn, M. P. Chang, and R. A. Wall, J. Am. Chem. Soc., 1955, 77, 3031; CrossRef (b) T. L. Amyes and J. P. Richard, J. Am. Chem. Soc., 1992, 114, 10297; CrossRef (c) M. Dai, Y. Feng, and P. J. Tonge, J. Am. Chem. Soc., 2001, 123, 506. CrossRef
25. A. E. Wright, J. C. Botelho, E. Gunzmán, D. Harmody, P. Linley, P. J. McCarthy, T. P. Pitts, S. A. Pomponi, and J. K. Reed, J. Nat. Prod., 2007, 70, 412. CrossRef
26. (a) W. Youngsaye, J. T. Lowe, F. Pohlki, P. Ralifo, and J. S. Panek, Angew. Chem. Int. Ed., 2007, 46, 9211; CrossRef (b) D. W. Custar, T. P. Zabawa, and K. A. Scheidt, J. Am. Chem. Soc., 2008, 130, 804. CrossRef
27. O. A. Ulanovskaya, J. Janjic, M. Suzuki, S. S. Sabharwal, P. T. Schumacker, S. J. Kron, and S. A. Kozmin, Nat. Chem. Biol., 2008, 4, 418. CrossRef
28. (a) V. V. Vintonyak and M. E. Maier, Org. Lett., 2008, 10, 1239; CrossRef (b) S. K. Woo, M. S. Kwon, and E. Lee, Angew. Chem. Int. Ed., 2008, 47, 3242; CrossRef (c) I. Paterson and N. A. Miller, Chem. Commun., 2008, 4708; CrossRef (d) R. Kartika, T. R. Gruffi, and R. E. Taylor, Org. Lett., 2008, 10, 5047; CrossRef (e) V. V. Vintonyak, B. Kunze, F. Sasse, and M. E. Maier, Chem. Eur. J., 2008, 14, 11132; CrossRef (f) D. W. Custar, T. P. Zabawa, J. Hines, C. M. Crews, and K. A. Scheidt, J. Am. Chem. Soc., 2009, 131, 12406; CrossRef (g) T. Wangyang and P. E. Floreancig, Angew. Chem. Int. Ed., 2009, 48, 4567; CrossRef (h) Ref 21b; (i) X. Guinchard and E. Roulland, Org. Lett., 2009, 11, 4700; CrossRef (j) J. S. Yadav and G. G. K. S. N. Kumar, Tetrahedron, 2010, 66, 480; CrossRef (k) Y. Cui, W. Tu, and P. E. Floreancig, Tetrahedron, 2010, 66, 4867; CrossRef (l) D. Martinez-Solorio and M. P. Jennings, J. Org. Chem., 2010, 75, 4095; CrossRef (m) Z. Yang, B. Zhang, G. Zhao, J. Yang, X. Xie, and X. She, Org. Lett., 2011, 13, 5916; CrossRef For synthetic studies on neopeltolide, see: (n) G. J. Florence and R. F. Cadou, Tetrahedron Lett., 2010, 51, 5761; CrossRef (o) E. Hartmann and M. Oestreich, Angew. Chem., Int. Ed., 2010, 49, 6195; CrossRef See also: (p) J. Gallon, S. Reymond, and J. Cossy, C. R. Chim., 2008, 11, 1463. CrossRef
29. For reviews of Suzuki—Miyaura coupling, see: (a) N. Miyaura and A. Suzuki, Chem. Rev., 1995, 95, 2457; CrossRef (b) A. Suzuki, ‘Metal-Catalyzed Cross-Coupling Reactions’ ed. by F. Diederich and P. J. Stang, Wiley-VCH, Weinheim, 1998, pp. 49; (c) A. Suzuki, J. Organometal. Chem., 1999, 576, 147; CrossRef (d) S. R. Chemler, D. Trauner, and S. J. Danishefsky, Angew. Chem. Int. Ed., 2001, 40, 4544; CrossRef (e) N. Miyaura, ‘Topics in Current Chemistry’, Vol. 219, Springer-Verlag, Heidelberg, 2002, pp. 11; (f) A. Suzuki and H. C. Brown, ‘Organic Syntheses via Boranes’, Vol. 3, Aldrich Chem. Co. Inc., Milwaukee, Wisconsin, 2003; (g) S. Kotha, K. Lahiri, and D. Kashinath, Tetrahedron, 2002, 58, 9633; CrossRef (h) A. Suzuki, Chem. Commun., 2005, 4759; CrossRef (i) A. Suzuki, Angew. Chem. Int. Ed., 2011, 50, 6722. CrossRef
30. For reviews of Suzuki—Miyaura coupling of enol phosphates, see: (a) J. D. Sellars and P. G. Steel, Chem. Soc. Rev., 2011, 40, 5170; CrossRef (b) B.-J. Li, D.-G. Yu, C.-L. Sun, and Z.-J. Shi, Chem. Eur. J., 2011, 17, 1728; CrossRef (c) H. Fuwa, Synlett, 2011, 6; CrossRef (d) H. Fuwa, Bull. Chem. Soc. Jpn., 2010, 83, 1401; CrossRef (e) M. Sasaki and H. Fuwa, Nat. Prod. Rep., 2008, 25, 401; CrossRef (f) M. Sasaki, Bull. Chem. Soc. Jpn., 2007, 80, 856; CrossRef (g) M. Sasaki and H. Fuwa, Synlett, 2004, 1851. CrossRef
31. For selected recent reviews of olefin metathesis, see: (a) A. Fürstner, Angew. Chem. Int. Ed., 2000, 39, 3012; CrossRef (b) A. Deiters and S. F. Martin, Chem. Rev., 2004, 104, 2199; CrossRef (c) K. C. Nicolaou, P. G. Bulger, and D. Sarlah, Angew. Chem. Int. Ed., 2005, 44, 4490; CrossRef (d) A. Gradillas and J. Pérez-Castells, Angew. Chem. Int. Ed., 2006, 45, 6086; CrossRef (e) A. H. Hoveyda and A. R. Zhugralin, Nature, 2007, 450, 243. CrossRef
32. For a review of synthetic strategies relying on Suzuki–Miyaura coupling and ring-closing metathesis, see: S. Kotha and K. Mandal, Chem. Asian J., 2009, 4, 354. CrossRef
33. (a) H. Fuwa, S. Naito, T. Goto, and M. Sasaki, Angew. Chem. Int. Ed., 2008, 47, 4737; CrossRef (b) H. Fuwa, A. Saito, S. Naito, K. Konoki, M. Yotsu-Yamashita, and M. Sasaki, Chem. Eur. J., 2009, 15, 12807. CrossRef
34. For reviews of Mitsunobu reaction, see: (a) O. Mitsunobu, Synthesis, 1981, 1; CrossRef (b) K. C. K. Swamy, N. N. B. Kumar, E. Balaraman, and K. V. P. P. Kumar, Chem. Rev., 2009, 109, 2551. CrossRef
35. For a review of CM, see: S. J. Connon and S. Blechert, Angew. Chem. Int. Ed., 2003, 42, 1900. CrossRef
36. H. Hanawa, D. Uraguchi, S. Konishi, T. Hashimoto, and K. Maruoka, Chem. Eur. J., 2003, 9, 4405. CrossRef
37. (a) S. BouzBouz, R. Simmons, and J. Cossy, Org. Lett., 2004, 6, 3465; CrossRef (b) C. C. Marvin, E. A. Voight, J. M. Suh, C. L. Paradise, and S. D. Burke, J. Org. Chem., 2008, 73, 8452. CrossRef
38. W. Yu, Y. Mei, Y. Kang, Z. Hua, and Z. Jin, Org. Lett., 2004, 6, 3217. CrossRef
39. H. C. Brown and P. K. Jadav, J. Am. Chem. Soc., 1983, 105, 2092. CrossRef
40. M. Scholl, S. Ding, C. W. Lee, and R. H. Grubbs, Org. Lett., 1999, 1, 953. CrossRef
41. For the role of H-bonding in olefin metathesis reactions of allylic alcohols, see: (a) A. H. Hoveyda, P. J. Lombardi, R. V. O’Brien, and A. R. Zhugralin, J. Am. Chem. Soc., 2009, 131, 8378; CrossRef (b) B. Schmidt and L. Staude, J. Org. Chem., 2009, 74, 9237; CrossRef (c) T. Imahori, H. Ojima, Y. Yoshimura, and H. Takahata, Chem. Eur. J., 2008, 14, 10762; CrossRef (d) D. A. Clark, J. R. Clark, and S. T. Diver, Org. Lett., 2008, 10, 2055; CrossRef (e) B. Schmidt and S. Nave, Chem. Commun., 2006, 2489; CrossRef (f) T. R. Hoye and H. Zhao, Org. Lett., 1999, 1, 1123; CrossRef For a review, see: Y. A. Lin and B. G. Davis, Beilstein J. Org. Chem., 2010, 6, 1219; CrossRef On the other hand, only a little is known about the impact of unprotected homoallylic alcohols on selectivity issues in olefin metathesis reactions. See: (g) F. C. Engelhardt, M. J. Schmitt, and R. E. Taylor, Org. Lett., 2001, 3, 2209; CrossRef (h) M. Lautens and M. L. Maddess, Org. Lett., 2004, 6, 1883. CrossRef
42. Treatment of a mixture of a benzyloxymethyl-protected derivative of 27 and methyl acrylate with G-II (CH2Cl2, room temperature) provided the corresponding RCM product as the major product in 71% yield. The desired CM product was isolated in only 25% yield.
43. E. D. Laganis and B. L. Chenard, Tetrahedron Lett., 1984, 25, 5831. CrossRef
44. J. Inanaga, K. Hirata, H. Saeki, T. Katsuki, and M. Yamaguchi, Bull. Chem. Soc. Jpn., 1979, 52, 1989. CrossRef
45. S. H. Hong, D. P. Sanders, C. W. Lee, and R. H. Grubbs, J. Am. Chem. Soc., 2005, 127, 17160. CrossRef
46. K. Kito, R. Ookura, S. Yoshida, M. Namikoshi, T. Ooi, and T. Kusumi, Org. Lett., 2008, 10, 225. CrossRef
47. S. M. Hande and J. Uenishi, Tetrahedron Lett., 2009, 50, 189. CrossRef
48. R. Ookura, K. Kito, Y. Saito, T. Kusumi, and T. Ooi, Chem. Lett., 2009, 38, 384. CrossRef
49. (a) T. Nagasawa and S. Kuwahara, Tetrahedron Lett., 2010, 51, 875; CrossRef (b) T. Nagasawa, T. Nukada, and S. Kuwahara, Tetrahedron, 2011, 67, 2882. CrossRef
50. T. Nagasawa and S. Kuwahara, Org. Lett., 2009, 11, 761. CrossRef
51. (a) P. Srihari and Y. Sridhar, Eur. J. Org. Chem., 2011, 6690; CrossRef (b) A. Zúñiga, M. Pérez, M. González, G. Gómez, and Y. Fall, Synthesis, 2011, 3301; CrossRef (c) Y. Izuchi, N. Kanomata, H. Koshino, Y. Hongo, T. Nakata, and S. Takahashi, Tetrahedron: Asymmetry, 2011, 22, 246; CrossRef (d) S. Díaz-Oltra, C. A. Angulo-Pachón, J. Murga, E. Falomir, M. Carda, and J. A. Marco, Chem. Eur. J., 2011, 17, 675; CrossRef (e) G. Sabitha, D. V. Reddy, A. S. Rao, and J. S. Yadav, Tetrahedron Lett., 2010, 51, 4195; CrossRef (f) A. J. M. Hendrix and M. P. Jennings, Tetrahedron Lett., 2010, 51, 4260; CrossRef (g) S. Díaz-Oltra, C. A. Angulo-Pachón, J. Murga, M. Carda, and J. A. Marco, J. Org. Chem., 2010, 75, 1775; CrossRef (h) S. Díaz-Oltra, C. A. Angulo-Pachón, M. N. Kneeteman, J. Murga, M. Carda, and J. A. Marco, Tetrahedron Lett., 2009, 50, 3783; CrossRef (i) J. Liu, K. Xu, J. He, L. Zhang, X. Pan, and X. She, J. Org. Chem., 2009, 74, 5063; CrossRef (j) J. D. Panarese and S. P. Waters, Org. Lett., 2009, 11, 5086; CrossRef (k) T. Nagasawa and S. Kuwahara, Biosci. Biotechnol. Biochem., 2009, 73, 1893; CrossRef See also: T. Nagasawa and S. Kuwahara, Heterocycles, 2012, 85, 587. CrossRef
52. Kusumi et al. reported that interconversion between 35 and 36 under the isolation conditions was not possible. See ref. 48. Shishido and co-workers later elucidated that treatment of trans-39 with KH (18-crown-6, THF, 0 °C, 0.5 h) gave cis-39 in 94% yield. See ref. 10f.
53. A. D. Mico, R. Margarita, L. Parlanti, A. Vescovi, and G. Piancatelli, J. Org. Chem., 1997, 62, 6974. CrossRef
54. (a) K. Takai, K. Nitta, and K. Utimoto, J. Am. Chem. Soc., 1986, 108, 7408; CrossRef (b) D. A. Evans and W. C. Black, J. Am. Chem. Soc., 1993, 115, 4497. CrossRef
55. Prepared from (S)-propylene oxide in two steps (vinylMgBr, THF, −20 °C; then BzCl, pyridine, Et2O, room temperature, 87% yield for the two steps).
56. C. R. Johnson and M. P. Braun, J. Am. Chem. Soc., 1993, 115, 11014. CrossRef
57. S. Ohta, M. M. Uy, M. Yanai, E. Ohta, T. Hirata, and S. Ikegami, Tetrahedron Lett., 2006, 47, 1957. CrossRef
58. N. Matsumori, D. Kaneno, M. Murata, H. Nakamura, and K. Tachibana, J. Org. Chem., 1999, 64, 866. CrossRef
59. M. S. Kwon, S. K. Woo, S. W. Na, and E. Lee, Angew. Chem. Int. Ed., 2008, 47, 1733. CrossRef
60. G. R. Pettit, C. L. Herald, D. L. Doubek, D. L. Herald, E. Arnold, and J. Clardy, J. Am. Chem. Soc., 1982, 104, 6846; CrossRef For a recent review on the chemistry and biology of bryostatins, see: K. J. Hale and S. Manaviazar, Chem. Asian J., 2010, 5, 704. CrossRef
61. J. Cossy, C. R. Chimie, 2008, 11, 1477. CrossRef
62. Scheidt et al. have recently disclosed the total synthesis of (−)-exiguolide that relies on Prins-type macrocyclization (ref. 13a). Roulland and co-workers reported their total synthesis of (−)-exiguolide almost simultaneously with our report: (a) C. Cook, X. Guinchard, F. Liron, and E. Roulland, Org. Lett., 2010, 12, 744; CrossRef For a synthetic study on (−)-exiguolide, see: (b) C. R. Reddy and N. N. Rao, Tetrahedron Lett., 2010, 51, 5840. CrossRef
63. (a) P. R. Blakemore, W. J. Cole, P. J. Kocienski, and A. Morley, Synlett, 1998, 26; CrossRef (b) P. R. Blakemore, J. Chem. Soc., Perkin Trans. 1, 2002, 2563; CrossRef (c) C. Aїssa, Eur. J. Org. Chem., 2009, 1831. CrossRef
64. M. D. Lewis, J. K. Cha, and Y. Kishi, J. Am. Chem. Soc., 1982, 104, 4976. CrossRef
65. L. V. Heumann and G. E. Keck, Org. Lett., 2007, 9, 4275. CrossRef
66. A. N. Rai and A. Basu, Tetrahedron Lett., 2003, 44, 2267. CrossRef
67. A. K. Ghosh, Y. Wang, and J. T. Kim, J. Org. Chem., 2001, 66, 8973. CrossRef
68. A. Basha, M. Lipton, and S. M. Weinreb, Tetrahedron Lett., 1977, 18, 4171. CrossRef
69. S. Nahm and S. M. Weinreb, Tetrahedron Lett., 1981, 22, 3815. CrossRef
70. S. B. Garber, J. S. Kingsbury, B. L. Gray, and A. H. Hoveyda, J. Am. Chem. Soc., 2000, 122, 8168. CrossRef
71. S. Chandrasekhar, S. R. Yaragorla, L. Sreelakshmi, and Ch. R. Reddy, Tetrahedron, 2008, 64, 5174. CrossRef
72. T. Nagamitsu, D. Takano, M. Seki, S. Arima, M. Ohtawa, K. Shiomi, Y. Harigaya, and S. Ōmura, Tetrahedron, 2008, 64, 8117. CrossRef
73. S. Takano, K. Samizu, T. Sugihara, and K. Ogasawara, J. Chem. Soc., Chem. Commun., 1989, 1344. CrossRef
74. H. X. Zhang, F. Guibe, and G. Balavoine, J. Org. Chem., 1990, 55, 1857. CrossRef
75. H. S. Schultz, H. B. Freyermuth, and S. R. Buc, J. Org. Chem., 1963, 28, 1140. CrossRef
76. (a) D. B. Dess and J. C. Martin, J. Org. Chem., 1983, 48, 4155; CrossRef (b) D. B. Dess and J. C. Martin, J. Am. Chem. Soc., 1991, 113, 7277. CrossRef
77. B. S. Bal, W. E. Childers, Jr., and H. W. Pinnick, Tetrahedron, 1981, 37, 2091. CrossRef
78. (a) K. Tanaka, Y. Ohta, and K. Fuji, Tetrahedron Lett., 1993, 34, 4071; CrossRef (b) D. A. Evans, P. H. Carter, E. M. Carreira, A. B. Charette, J. A. Prunet, and M. Lautens, J. Am. Chem. Soc., 1999, 121, 7540; CrossRef (c) K. Ohmori, Y. Ogawa, T. Obitsu, Y. Ishikawa, S. Nishiyama, and S. Yamamura, Angew. Chem. Int. Ed., 2000, 39, 2290. CrossRef
79. (Z)-Vinylboronate 60 was synthesized from the corresponding alkyne59 by means of trans-hydroboration developed by Miyaura and co-workers. See: T. Ohmura, Y. Yamamoto, and N. Miyaura, J. Am. Chem. Soc., 2000, 122, 4990. CrossRef
80. (a) Y. K. Reddy and J. R. Falck, Org. Lett., 2002, 4, 969; CrossRef (b) T. Gillmann and T. Weeber, Synlett, 1994, 649; CrossRef (c) J. Uenishi, J.-M. Beau, R. W. Armstrong, and Y. Kishi, J. Am. Chem. Soc., 1987, 109, 4756. CrossRef
81. The beneficial effects of H2O have been documented in several review articles. See ref. 29.
82. T. Yamori, A. Matsunaga, S. Sato, K. Yamazaki, A. Komi, K. Ishizu, I. Mita, H. Edatsugi, Y. Matsuba, K. Takezawa, O. Nakanishi, H. Kohno, Y. Nakajima, H. Komatsu, T. Andoh, and T. Tsuruo, Cancer Res., 1999, 59, 4042.
83. T. Yamori, Cancer Chemother. Pharmacol., 2003, 52 (Suppl. 1), 74. CrossRef
84. (a) C. L. Rigby and D. J. Dixon, Chem. Commun., 2008, 3798; CrossRef (b) R. D. Mazery, M. Pullez, F. López, S. R. Harutyunyan, A. J. Minnaard, and B. L. Feringa, J. Am. Chem. Soc., 2005, 127, 9966; CrossRef (c) M. Bandini, A. Melloni, S. Tommasi, and A. Umani-Ronchi, Helv. Chim. Acta, 2003, 86, 3753; CrossRef (d) K. Agapiou and M. J. Krische, Org. Lett., 2003, 5, 1737; CrossRef (e) G. E. Keck and D. S. Welch, Org. Lett., 2002, 4, 3687; CrossRef (f) E. Emori, T. Arai, H. Sasai, and M. Shibasaki, J. Am. Chem. Soc., 1998, 120, 4043. CrossRef
85. T. C. Wabnitz, J.-Q. Yu, and J. B. Spencer, Chem. Eur. J., 2004, 10, 484. CrossRef
86. The following α,β-unsaturated thioesters were used in the CM: S-Ethyl 2-propenthioate: C. R. Noller and J. J. Gordon, J. Am. Chem. Soc., 1933, 55, 1090; CrossRef S-(Substituted aryl) 2-propenthioates: K. Yamamoto, I. Kawabata, E. Fujisawa, and M. Suzuki, Japanese Patent JP2003-146967. S-(1-Naphthyl) 2-propenthioate: ref. 84a.
87. Note that olefins 89 and 92 are racemic materials.
88. For selected recent examples of hetero-Michael addition of α,β-unsaturated amides and imides, see: (a) Y. Hamashima, S. Suzuki, T. Tamura, H. Somei, and M. Sodeoka, Chem. Asian J., 2011, 6, 658; CrossRef (b) C. B. W. Phippen, J. K. Beattie, and C. S. P. McErlean, Chem. Commun., 2010, 8234; CrossRef (c) S. Khaliel, M. V. Nandakumar, H. Krautscheid, and C. Schneider, Synlett, 2008, 2705; CrossRef (d) I. Reboule, R. Gil, and J. Collin, Eur. J. Org. Chem., 2008, 532; CrossRef (e) M. P. Sibi and T. Soeta, J. Am. Chem. Soc., 2007, 129, 4522; CrossRef (f) S. Kikuchi, H. Sato, and S. Fukuzawa, Synlett, 2007, 2436; CrossRef (g) C. D. Vanderwal and E. N. Jacobsen, J. Am. Chem. Soc., 2004, 126, 14724; CrossRef (h) T. C. Wabnitz and J. B. Spencer, Org. Lett., 2003, 5, 2141; CrossRef (i) W. Zhuang, R. G. Hazell, and K. A. Jørgensen, Chem. Commun., 2001, 1240 and references cited therein. CrossRef
89. The following α,β-unsaturated amides/imides were used in the CM: 1-(2-Propenoyl)pyrrolidine: K. Tanaka, Y. Hagiwara, and K. Noguchi, Angew. Chem. Int. Ed., 2005, 44, 7260; CrossRef 1-(3-Propenoyl)-2-pyrrolidinone: M. P. Sibi and H. Miyabe, Org. Lett., 2002, 4, 3435; CrossRef 3-(2-Propenoyl)-2-oxazolidinone, 3-(2-propenoyl)-2-benzoxazolidinone, 1-(2-propenoyl)indole, and 1-(2-propenoyl)indoline: J. Magolan, C. A. Carson, and M. A. Kerr, Org. Lett., 2008, 10, 1437; CrossRef 1-(2-Propenoyl)-2,5-dimethylpyrrole: ref. 88b..
90. For a discussion on the reactivity of α,β-unsaturated ester surrogates, see: S. Matsunaga, T. Kinoshita, S. Okada, S. Harada, and M. Shibasaki, J. Am. Chem. Soc., 2004, 126, 7559. CrossRef
91. For a review of N-acylpyrroles, see: A. M. Goldys and C. S. P. McErlean, Eur. J. Org. Chem., in press. DOI: 10.1002/ejoc.201101470. CrossRef
92. Here, we use the terms “α,β-unsaturated 2-oxazolidinone imides” and “α,β-unsaturated 2,5-dimethylpyrrole amides” in contradistinction to “α,β-unsaturated (thio)esters”, although they may not be correct in light of the IUPAC nomenclature.
93. (a) R. J. Loncharich, T. R. Schwartz, and K. N. Houk, J. Am. Chem. Soc., 1987, 109, 14; CrossRef (b) M. F. Ruiz-López, X. Assfeld, J. I. García, J. A. Mayoral, and L. Salvatella, J. Am. Chem. Soc., 1993, 115, 8780. CrossRef
94. K. N. Houk, M. N. Paddon-Row, N. G. Rondan, Y.-D. Wu, F. K. Brown, D. C. Spellmeyer, J. T. Metz, Y. Li, and R. J. Loncharich, Science, 1986, 231, 1108. CrossRef
95. K. N. Houk and R. W. Strozier, J. Am. Chem. Soc., 1973, 95, 4094. CrossRef
96. (a) J. L. Jensen and D. J. Carré, J. Org. Chem., 1974, 39, 2103; CrossRef (b) J. L. Jensen and A. T. Thibeault, J. Org. Chem., 1977, 42, 2168. CrossRef
97. The Reymond/Cossy group recently reported a FeCl3-catalyzed stereoselective synthesis of 2,6-cis-substituted tetrahydropyrans from ζ-hydroxy allylic alcohol derivatives. They proposed that the cyclization involves an allylic cation as the intermediate, which is similar to our mechanistic proposal. See ref. 17a.
98. (a) M. W. Cronyn, M. P. Chang, and R. A. Wall, J. Am. Chem. Soc., 1955, 77, 3031; CrossRef (b) F. G. Bordwell and H. E. Fried, J. Org. Chem., 1991, 56, 4218; CrossRef (c) M. C. Kohler, J. M. Yost, M. R. Garnsey, and D. M. Coltart, Org. Lett., 2010, 12, 3376. CrossRef
99. T. Fukuyama, S. C. Lin, and L. Li, J. Am. Chem. Soc., 1990, 112, 7050. CrossRef
100. M. Kurosu, Tetrahedron Lett., 2000, 41, 591. CrossRef
101. (a) H. Prokopcová and C. O. Kappe, Angew. Chem. Int. Ed., 2009, 48, 2276; CrossRef (b) T. Fukuyama and H. Tokuyama, Aldrichimica Acta, 2004, 37, 87.
102. (a) R. Wittenberg, J. Srogl, M. Egi, and L. S. Liebeskind, Org. Lett., 2003, 5, 3033; CrossRef (b) H. Li, H. Yang, and L. S. Liebeskind, Org. Lett., 2008, 10, 4375. CrossRef
103. L. S. Liebeskind and J. Srogl, J. Am. Chem. Soc., 2000, 122, 11260. CrossRef
104. H. Tokuyama, T. Miyazaki, S. Yokoshima, and T. Fukuyama, Synlett, 2003, 1512. CrossRef
105. (a) H. Fuwa, K. Mizunuma, S. Matsukida, and M. Sasaki, Tetrahedron, 2011, 67, 4995; CrossRef (b) H. Fuwa, S. Matsukida, and M. Sasaki, Synlett, 2010, 1239. CrossRef
106. A. Basha, M. Lipton, and S. M. Weinreb, Tetrahedron Lett., 1977, 18, 4171. CrossRef
107. D. A. Evans, T. C. Britton, and J. A. Ellman, Tetrahedron Lett., 1987, 28, 6141. CrossRef
108. (a) S. D. Lee, M. A. Brook, and T. H. Chan, Tetrahedron Lett., 1983, 24, 1569; CrossRef (b) S. Brandange and B. Rodriguez, Acta Chem. Scand. Ser. B, 1987, 41, 740; CrossRef (c) E. Arai, H. Tokuyama, M. S. Linsell, and T. Fukuyama, Tetrahedron Lett., 1998, 39, 71; CrossRef (d) D. A. Evans, G. Borg, and K. A. Scheidt, Angew. Chem. Int. Ed., 2002, 41, 3188. CrossRef