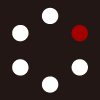
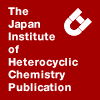
HETEROCYCLES
An International Journal for Reviews and Communications in Heterocyclic ChemistryWeb Edition ISSN: 1881-0942
Published online by The Japan Institute of Heterocyclic Chemistry
e-Journal
Full Text HTML
Received, 14th February, 2012, Accepted, 19th March, 2012, Published online, 27th March, 2012.
DOI: 10.3987/COM-12-12449
■ Photoinduced Selective Transformation of N-Acyl-α-dehydro(9-phenanthryl)alaninamides into Their Cyclization Intermediates in a Polar Protic Solvent
Yuki Kawanishi, Hiroko Yoshiie, Tetsutaro Igarashi, and Tadamitsu Sakurai*
Department of Material and Life Chemistry, Faculty of Engineering, Kanagawa University, 3-27-1 Rokkakubashi, Kanagawa-ku, Yokohama 221-8686, Japan
Abstract
Irradiation of the title compounds [(Z)-1] in methanol afforded trans-3,8a-dihydro-2-dibenzo[f,h]quinolinone derivatives (trans-2) in preference to cis-2, all of which serve as precursors of the corresponding 3,4-dihydrodibenzoquinolinones. An analysis of the substituent and solvent effects on the cyclization occuring from the excited-state (E)-1 showed that the reactivity of this (E)-isomer is greatly influenced by the steric bulk of the alkyl group attached to the N´-amide nitrogen of the starting 1. In addition, this analysis confirmed that the stabilization of the excited-state (E)-1 (having a zwitterionic structure), by hydrogen-bonding and charge-transfer interactions with methanol, is an essential prerequisite for the occurrence of the photocyclization reaction selectively forming trans-2 and cis-2.Recently, photoinduced electron transfer (PET) has attracted much attention as a means for activating organic molecules, owing to the potential for PET-initiated reactions of these molecules, to pave the way for the construction of various heteroatom-containing ring systems exhibiting potent pharmacological activities.1 In the course of a systematic study on the PET reactions of N-acyl-α-dehydroarylalaninamides and N-acyl-α-dehydroarylalanine alkyl esters, we found that these derivatives readily undergo one-electron reduction in the presence of a tertiary aliphatic amine to enable the highly selective construction of 3,4-dihydroquinolinone and 4,5-dihydrooxazole ring systems, respectively, through the corresponding reactive radical ion pair intermediates.2,3 However, all attempts to isolate and characterize the cyclization intermediate, the presumed precursor of the substituted 3,4-dihydroquinolinones, were unsuccessful.2 The elucidation of the structure and reactivity of this hypothetical intermediate would help to unravel the mechanism for the novel PET-initiated cyclization reaction, leading to these six-membered heterocyclic compounds. In other words, if the cyclized intermediate could be isolated, its stability and stereochemistry could be determined.
n previous studies, we reported that the selectivity for the formation of the 3,4-dihydrobenzoquinolinone derivatives is decreased with an increase in the steric bulk of the alkyl group attached to the N´-amide nitrogen of the starting α-dehydronaphthylalaninamides, the reactivities of which are much greater in methanol than in acetonitrile.2a In addition, the 1H NMR spectral analysis of the photocyclization processes for these naphthylalaninamides confirmed that the naphthalene ring is not able to stabilize a cyclization intermediate enough to permit its isolation. On the basis of the reasonable assumption that the π-electrons at the 9- and 10-positions on the phenanthrene ring are less delocalized than those on the naphthalene ring, we introduced a 9-phenanthryl group into the aryl moiety of the starting α-dehydroarylalaninamides for the purpose of enhancing the stability of the cyclization intermediate. In this communication, we report the effects of the substituent and solvent on the photoreactivity of (Z)-N-acyl-α-dehydro(9-phenanthryl)alaninamides [(Z)-1a–d, Chart 1] as well as on the composition and stability of the cyclization intermediates formed as the precursors of the corresponding 3,4-dihydroquinolinone derivatives.
The starting α-dehydrophenanthrylalaninamides of the (Z)-configuration were prepared in 50–57% overall yields by a Knoevenagel-type condensation between 9-phenanthrene-carbaldehyde and N-benzoylglycine [(Z)-1a–c] or N-acetylglycine [(Z)-1d] in acetic anhydride containing sodium acetate, followed by ring-opening reactions of the resulting 2-substituted (Z)-4-(9-phenanthrylmethylene)-5(4H)-oxazolone, mediated by methylamine [(Z)-1a,d], benzylamine [(Z)-1b], or ammonia [(Z)-1c] in THF.2,4 To examine the distribution and composition of the photoproducts generated under the PET conditions, a nitrogen-saturated methanol solution (500 mL) containing (Z)-1a (4.0 x 10–3 mol dm–3) and triethylamine (TEA, 0.10 mol dm–3) was irradiated at wavelengths longer than 280 nm for 20 min at room temperature (conversion: 100%, light source: 400 W high-pressure Hg lamp). The resulting reaction mixture was immediately subjected to preparative thin layer chromatography using silica gel (eluent: EtOAc-hexane or EtOAc-CHCl3). Standard workup led to the isolation of trans-2a [an equimolar mixture of (3S,8aS)- and (3R,8aR)-3-benzoylamino-3,8a-dihydro-1-methyl-2(1H)-dibenzo[f,h]quinolinones, 60% yield] and cis-2a [an equimolar mixture of (3S,8aR)- and (3R,8aS)-3-benzoylamino-3,8a-dihydro-1-methyl-2(1H)-dibenzo[f,h]quinolinones, 18% yield], along with additional minor products.5 From the mixture of minor products, we isolated 3-benzoylamino-3,4-dihydro-1-methyl-2(1H)-dibenzo[f,h]quinolinone (3a, <1%) and its structural isomers by means of reverse-phase preparative HPLC (mobile phase: MeCN-H2O, 3:2 v/v).6 A summary of these results is shown in Scheme 1. The structures of the above products were confirmed by mass spectral analysis as well as 1H difference NOE (0% for H3-H8a of trans-2a and 5% for H3-H8a of cis-2a in CDCl3) and 1H-1H COSY NMR spectral analyses. In addition to the 1H NMR and mass spectral analyses of the structural isomers of dihydrodibenzoquinolinone derivative 3a, HPLC analysis of these isomers suggested the presence of at least two isomers possessing the same molecular weight as 3a ([M]+ 380.43, Maldi TOF-MS). As shown in Scheme 1, the same product distribution as that of (Z)-1a was observed for (Z)-1b–d. On the other hand, the (E)-isomer of 1a was isolated from the reaction mixture obtained by 30-min irradiation of a nitrogen-saturated methanol solution containing (Z)-1a (4.0 x 10–3 mol dm–3) and benzophenone (2.0 x 10–2 mol dm–3) at wavelengths longer than 340 nm (400 W high-pressure Hg lamp) by a similar workup.5,7
As described above, we isolated the predicted cyclization intermediates, trans-2a and cis-2a, as the major photoproducts, constituting 92% of the product mixture (1H NMR spectral analysis). These intermediates were stable for a long period of time in the absence of TEA, but slowly converted into 3a and its isomers in the presence of this amine, thus providing strong evidence that 2a is the precursor of 3a. In addition, this finding led us to predict that the irradiation of a methanol solution of (Z)-1a in the absence of TEA may yield trans-2a and cis-2a selectively, without forming any other products. Since these photoproducts underwent negligible decomposition under the irradiation conditions tested, the progress of the observed photocyclization was monitored by 1H NMR spectrometry. The results shown in Table 1 confirm that 2a is selectively and efficiently formed, being consistent with our prediction.8
Taking into account that the excited-state (E)-1 is highly reactive and serves as the precursor of 2, we assumed that the (Z)-isomer adopts a hydrogen bond- and charge-transfer-assisted zwitterion structure (shown in Figure 1), which is highly susceptible to isomerization to the (E)-isomer. As suggested in the introductory section, the less delocalized π-electrons at the 9- and 10-positions on the phenanthrene ring (as compared to π-electrons on the naphthalene ring) are more likely to migrate to the N´-amide carbonyl oxygen through conjugation in the excited state.
On the basis of the above consideration, we propose a mechanism that explains the observed product
distribution (Scheme 2). The nucleophilic attack of the R1NH nitrogen at the carbocation at the 10 position on the ring, which should occur in competition with the deactivation of the excited-state (E)-1 to the ground-state 1, may afford a zwitterionic cyclization intermediate, and subsequent proton shift and keto-enol tautomerization readily converts this intermediate into 2. The fact that the cyclization of (E)-1a in methanol does not occur without irradiation suggests the negligible contribution of a zwitterion structure in the ground-state (E)-isomer. Since the conjugated carbonyl oxygen in the excited-state (E)-1 is also potentially capable of participation in the cyclization step, we considered the possibility that the isolated cyclization intermediate does not possess a lactam ring but an iminolactone ring, as depicted in Scheme 2. The exocyclic carbon-nitrogen double bond in this intermediate is known to be susceptible to acid-catalyzed hydrolysis that transforms this double bond into the carbon-oxygen double bond.9 Thus, the observation that 2a is very stable for a long period of time in 75 vol% DMSO-H2O
containing 0.5 mol dm–3 HCl at room temperature (1H NMR spectral analysis) rules out the possibility that the conjugated carbonyl oxygen is involved in the cyclization step described above. It is likely that the intramolecular hydrogen bond formed between the R2C(=O)NH hydrogen and the N´-amide carbonyl oxygen enables the exclusive participation of the R1NH nitrogen in this step. Another feature of the process highlighted by the results given in Table 1 is the preferential formation of trans-2 with respect to cis-2. To elucidate the reason for this bias, the energy-minimized conformations of trans-2a and cis-2a were determined by MM2 and PM5 calculations.10 A comparison of the heats of formation for these two conformations (estimated by the latter calculation), as depicted in Figure 2, established that the former isomer is thermodynamically more stable than the latter. Therefore, we conclude that the photochemical conversion of the excited-state (E)-1 into 2 is a thermodynamically controlled process.
Next, we focused our attention on the effects of the substituent and solvent on the photoreactivity of 1 as well as on the distribution and composition of the photoproducts, which are summarized in Table 1.
While there was a tendency for the photoreactivity to decrease with an increase in the steric bulk of the substituent R1: 1a (R1 = Me) ≈ 1c (R1 = H) >> 1b (R1 = CH2Ph), the thermodynamically more stable trans-isomers were preferentially formed in all derivatives. This increase in the steric bulk may enhance the energy barrier to the photocyclization of the excited-state (E)-1 but may exert only a minor effect on the nucleophilicity of the R1NH nitrogen, resulting in an acceleration of the deactivation of this (E)-isomer. Interestingly, irradiation of (Z)-1c under the same conditions as those for (Z)-1a afforded a minor amount of the dihydrodibenzoquinolinone derivative 3c in addition to the expected cyclization intermediates trans-2c and cis-2c. A control experiment showed that these intermediates were less stable and slowly converted into 3c even in the absence of TEA at room temperature, suggesting that a decrease in the steric bulk of the substituent R1 lowers the energy barrier for hydrogen migration from the 8a-position to the 4-position on the dihydrodibenzoquinolinone ring. In contrast, the replacement of the benzoyl group in 1a by the less bulky and less electron-withdrawing acetyl group 1d exerted a negligible effect on the photoreactivity of 1, though it decreased the selectivity of trans-2 formation to some extent (74%–67%). Surprisingly, when a nitrogen-saturated acetonitrile solution of (Z)-1a was irradiated at wavelengths longer than 280 nm in the absence of TEA, it underwent only photoisomerization to the (E)-isomer without giving any products (Table 1). Since the polar aprotic solvent, acetonitrile, almost has the same polarity as that of methanol, this finding provided a strong piece of evidence, supporting the conclusion that the stabilization of a zwitterion structure [for the excited-state (E)-1] by hydrogen-bonding and charge-transfer interactions with methanol is a vital prerequisite for the occurrence of the observed photocyclization reaction.
ACKNOWLEDGMENTS
This research was partly supported by “Scientific Frontier Research Project” from the Ministry of Education, Culture, Sports, Science and Technology in Japan.
References
1. a) ‘Synthetic Organic Photochemistry,’ ed. by W. M. Horspool, Plenum, New York, 1984; b) ‘Synthetic Organic Photochemistry,’ ed. by A. G. Griesbeck and J. Mattay, Marcel Dekker, New York, 2005; c) A. G. Griesbeck, N. Hoffmann, and K. Warzecha, Acc. Chem. Res., 2007, 40, 128. CrossRef
2. a) K. Maekawa, T. Igarashi, K. Kubo, and T. Sakurai, Tetrahedron, 2001, 57, 5515; CrossRef b) T. Motohashi, K. Maekawa, K. Kubo, T. Igarashi, and T. Sakurai, Heterocycles, 2002, 57, 269; CrossRef c) K. Maekawa, H. Kajiwara, Y. Iseya, T. Igarashi, and T. Sakurai, Heterocycles, 2003, 60, 637; CrossRef d) K. Maekawa, A. Shinozuka, M. Naito, T. Igarashi, and T. Sakurai, Tetrahedron, 2004, 60, 10293; CrossRef e) K. Maekawa, K. Fujita, K. Iizuka, T. Igarashi, and T. Sakurai, Heterocycles, 2005, 65, 117. CrossRef
3. a) K. Maekawa, T. Sasaki, K. Kubo, T. Igarashi, and T. Sakurai, Tetrahedron Lett., 2004, 45, 3663; CrossRef b) K. Maekawa, N. Hishikawa, K. Kubo, T. Igarashi, and T. Sakurai, Tetrahedron, 2007, 63, 11267; CrossRef c) Y. Sato, A. Yoshida, T. Igarashi, and T. Sakurai, Heterocycles, 2010, 81, 997. CrossRef
4. a) Y. S. Rao and R. Filler, Synthesis, 1975, 749; CrossRef b) K. Noda, Y. Shimohigashi, and N. Izumiya, ‘The Peptides–Analysis, Synthesis, Biology,’ ed. by E. Gross and J. Meienhofer, Academic Press, New York, 1983, pp. 285–339; Data for (Z)-1a: mp 236.5–237.0 °C; IR (KBr) ν/cm–1 = 3300, 3218, 1633; 1H NMR (600 MHz, DMSO-d6) δ = 2.76 (3H, d, J = 4.1 Hz), 7.39 (2H, dd, J = 7.6, 7.6 Hz), 7.48 (1H, dd, J = 7.6, 7.6 Hz), 7.58 (1H, dd, J = 7.6, 8.2 Hz), 7.66 (1H, dd, J = 7.6, 8.2 Hz), 7.68 (1H, dd, J = 7.6, 8.2 Hz), 7.72 (1H, dd, J = 7.6, 8.2 Hz), 7.75 (1H, s), 7.75 (1H, d, J = 8.2 Hz), 7.81 (2H, d, J = 7.6 Hz), 7.92 (1H, s), 8.09 (1H, d, J = 8.2 Hz), 8.23 (1H, q, J = 4.1 Hz), 8.80 (1H, d, J = 8.2 Hz), 8.86 (1H, d, J = 8.2 Hz), 9.78 (1H, s); 13C NMR (150 MHz, DMSO-d6) δ = 26.4, 122.8, 123.3, 125.1, 126.2, 126.9 (2C), 127.0, 127.1, 127.3, 127.8 (2C), 128.1 (2C), 128.5, 129.7, 129.8, 130.0, 130.2, 130.8, 131.5, 132.9, 133.9, 165.1, 166.3. Anal. Calcd for C25H20N2O2: C, 78.93; H, 5.30; N, 7.36. Found: C, 78.97; H, 5.22; N, 7.27.
5. Data for trans-2a: mp 204.0–205.0 °C; IR (KBr) ν/cm-1 = 3253, 1657; 1H NMR (600 MHz, DMSO-d6): δ = 3.18 (3H, s), 5.20 (1H, ddd, J = 2.5, 5.0, 8.0 Hz), 5.40 (1H, dd, J = 2.0, 5.0 Hz), 5.87 (1H, dd, J = 2.0, 2.5 Hz), 7.30 (1H, d, J = 7.5 Hz), 7.39–7.52 (7H, m), 7.57 (1H, dd, J = 7.0, 7.5 Hz), 7.88 (1H, d, J = 7.5 Hz), 7.94 (2H, d, J = 7.0 Hz), 7.99 (1H, d, J = 7.5 Hz), 9.05 (1H, d, J = 8.0 Hz); 13C NMR (150 MHz, DMSO-d6) δ = 35.4, 47.2, 62.5, 120.9, 122.8, 123.7, 124.4, 125.3, 127.4 (2C), 127.7,128.0, 128.3 (2C), 128.6, 129.1, 131.4, 132.5, 133.1, 133.4, 133.9, 134.0, 136.1, 166.0, 166.9. HR EI-MS m/z calcd for C25H20N2O2: 403.1422 [M + Na]+. Found: 403.1421; Data for cis-2a: mp 120.0–121.0 °C; IR (KBr) ν/cm-1 = 3276, 1669; 1H NMR (600 MHz, DMSO-d6) δ = 3.20 (3H, s), 5.24 (1H, ddd, J = 4.0, 4.0, 8.0 Hz), 5.39 (1H, dd, J = 2.0, 4.0 Hz), 5.94 (1H, dd, J = 2.0, 4.0 Hz), 7.34 (1H, dd, J = 5.0, 5.0 Hz), 7.39–7.43 (5H, m), 7.45–7.51 (2H, m), 7.59 (1H, d, J = 7.5 Hz), 7.80 (2H, d, J = 7.5 Hz), 7.85–7.87 (1H, m), 7.99 (1H, d, J = 7.5 Hz), 9.07 (1H, d, J = 8.0 Hz); 13C NMR (150 MHz, DMSO-d6) δ = 37.3, 48.4, 62.4, 119.6, 124.0, 124.6, 125.4, 125.5, 127.9, 128.0 (2C), 128.3, 128.7 (2C), 129.0, 129.8, 131.8, 133.1, 133.4, 134.5, 134.6, 134.7, 136.8, 166.6, 167.2. HR EI-MS m/z calcd for C25H20N2O2: 403.1422 [M + Na]+. Found: 403.1365; Data for (E)-1a: mp 180.0–181.0 °C; IR (KBr) ν/cm-1 = 3421, 3253, 1647; 1H NMR (600 MHz, DMSO-d6) δ = 2.45 (3H, d, J = 4.5 Hz), 7.34 (1H, s), 7.56 (2H, dd, J = 7.0, 8.0 Hz), 7.60–7.76 (6H, m), 7.88 (1H, d, J = 8.0 Hz), 7.93 (1H, q, J = 5.0 Hz), 8.01 (2H, d, J = 7.0 Hz), 8.15 (1H, d, J = 8.0 Hz), 8.81 (1H, d, J = 8.0 Hz), 8.89 (1H, d, J = 8.0 Hz), 10.33 (1H, s); 13C NMR (150 MHz, DMSO-d6) δ = 26.4, 116.6, 123.3, 123.8, 125.9, 126.5, 127.36, 127.39, 127.4, 127.6, 128.4 (2C), 128.9 (2C), 129.0, 129.9, 130.3, 131.2, 131.3, 131.7, 132.4, 134.4, 135.8, 165.5, 165.6. HR EI-MS m/z calcd for C25H20N2O2: 403.1422 [M + Na]+. Found: 403.1389.
6. Data for 3a: 1H NMR (600 MHz, DMSO-d6) δ = 3.36 (1H, dd, J = 14.5, 16.0 Hz), 3.44 (3H, s), 3.69 (1H, dd, J = 6.0, 16.0 Hz), 4.95 (1H, ddd, J = 6.0, 8.0, 14.5 Hz), 7.54 (2H, dd, J = 7.0, 7.5 Hz), 7.60 (1H, dd, J = 7.5, 7.5 Hz), 7.67–7.76 (4H, m), 8.00 (2H, d, J = 7.0 Hz), 8.06 (1H, d, J = 7.5 Hz), 8.15 (1H, d, J = 7.5Hz), 8.87 (2H, d, J = 8.0 Hz), 8.91 (1H, d, J = 8.0 Hz); 13C NMR (150 MHz, DMSO-d6) δ = 37.9, 49.1, 67.0, 121.8, 123.2, 123.7, 123.8, 124.2, 124.9, 126.1, 126.6, 126.7, 127.4 (2C), 127.6, 127.9, 128.4 (2C), 129.3, 130.5, 131.5, 134.0, 135.3, 166.2, 171.2. MALDI TOF-MS m/z calcd for C25H20N2O2: 380.47 [M]+. Found: 380.31.
7. H. Hoshina, H. Tsuru, K. Kubo, T. Igarashi, and T. Sakurai, Heterocycles, 2000, 53, 2261. CrossRef
8. To monitor the progress of the reaction for (Z)-1a, nitrogen-saturated methanol solutions of (Z)-1a (1.0 × 10–3 mol dm–3, 10 mL × 3) were irradiated in parallel using a merry-go-round irradiation apparatus (light source: 400 W high-pressure Hg lamp, filter: Pyrex glass) for a given period of time at room temperature. After irradiation, the solutions were concentrated in vacuo to dryness and the resulting residues were subjected to 1H NMR spectral analysis. The composition of the photoproducts was estimated on the basis of the area ratio of the signals characteristic of each product.
9. B. A. Cunningham and G. L. Schmir, J. Am. Chem. Soc., 1966, 88, 551. CrossRef
10. MM2 and PM5 calculations were performed by using CAChe 5.0 for Windows available from Fujitsu Ltd, 2002.