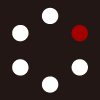
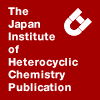
HETEROCYCLES
An International Journal for Reviews and Communications in Heterocyclic ChemistryWeb Edition ISSN: 1881-0942
Published online by The Japan Institute of Heterocyclic Chemistry
e-Journal
Full Text HTML
Received, 16th February, 2012, Accepted, 21st March, 2012, Published online, 30th March, 2012.
DOI: 10.3987/REV-12-735
■ Reactions of Pyridinium N-Ylides and Their Related Pyridinium Salts
Akikazu Kakehi*
Department of Chemistry and Material Engineering, Faculty of Engineering, Shinshu University, 4-17-1 Wakasato, Nagano 380-8553, Japan
Abstract
This review describes some reactions of pyridinium N-ylides and their closely related pyridinium salts and the formation of various nitrogen-containing heterocycles which are not easily obtainable by other methods and are of high synthetical and pharmaceutical interest.1. INTRODUCTION
Various reactions of pyridinium N-ylides and related pyridinium salts have been documented by many researchers since the first formation of pyridinium phenacylides by Dr. F. Kröhnke,1 and some reviews of these compounds have also been provided.2 From the mutual structural relations between the corresponding pyridinium methylides and aminides (I) and pyridinium salts (II) shown in Figure 1, four major reactions for pyridinium N-ylides (I), 1) the dipolar cycloaddition and cyclization of Ib or Id, 2) the nucleophilic addition of the ylidic anion of Ia, 3) the electrophilic addition of a positive carbon at the 2- (Ib), 4- (Ic), or 6-position (Id) on the pyridine ring, and 4) the ylidic bond fission, can be expected, while two major ones for pyridinium salts (II), 1) the electrophilic addition of a positive carbon at the 2- (IIb), 4- (IIc) or 6-position (IId) on the pyridine ring and 2) the nucleophilic addition of the carbanion or amide of IIIa or IVa generated by the deprotonation of the acidic methylene or amino group at the 2-, 4-, or 6-position, can be anticipated. This review covers the theoretically and synthetically important reactions of pyridinium N-ylides (I) and their related pyridinium salts (II and others) as of early 2012.
2. SYNTHESIS OF PYRIDINIUM YLIDES AND THEIR RELATED SALTS
Synthetic routes to pyridinium methylides 3−7, 3-(1-pyridinio)thiophene-2-thiolates 8−10 and pyridinium aminides 12−17 are shown for compounds which will be used in this review as their representatives in Scheme 1. These routes consist of the formation of simple types of pyridinium methylides (3) and aminides (12) via the alkaline treatment of the pyridinium salts 2 and 11 which are easily obtainable by
the reactions of pyridine derivatives (1) with various electrophiles such as alkyl halides, hydroxylamine O-sulfonic acid (HAS)3 or hydroxylamine O-mesitylenesulfonate (HMes),4 followed by their functionalization. For example, pyridinium N-ylides 4−7 and 13−17 can be easily synthesized by the introduction reactions of an acyl,5 vinyl,6 imidoyl,7 or thiocarbonyl group8 onto the ylidic anion atom of ylides 3 and 12, and the intramolecular dehydration or nucleophilic addition of the functionalized pyridinium methylides such as 6 obtained thus provide the corresponding 3-(1-pyridinio)thiophene-2- thiolates (8−10).9 The nucleophilicity of the ylidic anion atom (nitrogen) in pyridinium aminides 12 in these reactions is higher to some extent than that in pyridinium methylides 3, because methylides 3 did not attack an ester carbonyl10 and an imidate carbon atom7 but aminides 12 reacted with them. On the other hand, pyridinium dicyanomethylides (4) have been generally prepared by the reactions of tetracyanoethylene oxide (TCNEO) with pyridine derivatives (1).11 Though the direct formation of pyridinium acylaminides (13) from the thermolysis of acyl azides in the presence of pyridine derivatives (1) are also known,12 such methods are not usually used except for a few examples because of their handling difficulty and their narrow scope of application.
It is important to notice that pyridinium ylides such as 3, 4, and 13 have planar or near planar structures (dipole like) but other ylides 5−10 and 14−17 have non-planar structures owing to the cation-anion and cation-π interactions (Figure 2). The cation-anion interaction of pyridinium ylides 3 and 13 having an acyl group on the ylidic anion atom as shown in its betaine structures 3’ and 13’ is negligible, but that of pyridinium ylides 6, 7, 16, and 17 possessing a thiocarbonyl group at the same position is not so and has significant influence on the structures, since the carbon-sulfur bond is considerably longer than the carbon-oxygen bond and this situation is favorable for the intramolecular cation-anion interaction. In addition pyridinium ylides 5 and 14 which have an aryl group on the anionic terminal of the 1,5-dipoles should have nearly perpendicular structures (allyl anion like) due to the strong cation-π interactions between the pyridinium ring and the aryl ring.13
Pyridinium salts related to pyridinium methylides and aminides can be formed by their reactions with various alkyl halides, α-haloacetates, and α-haloketones. Pyridinium ylides 3, 12, and 13 are alkylated at the anion atom of the 1,3-dipolar structure and ylides 5−10 and 14−17 at that of the 1,5-dipolar structure.
3. REACTIONS OF PYRIDINIUM YLIDES
3.1. REACTIONS AS DIPOLES
Thermal 1,3-dipolar cycloadditions (π4s+π2s) of pyridinium (monosubstituted)methylides (3) and (N-monosubstituted)aminides (12) with various electron-poor alkenes, alkynes, and nitriles are well known routes for obtaining indolizines, pyrazolo[1,5-a]pyridines, and 1,2,4-triazolo[1,5-a]pyridines. For example, the 1,3-dipolar form (3’) of methylides 3 reacted with acrylates, acrylonitriles, and maleimides to form the corresponding 1,2,3,8a-tetrahydroindolizine derivatives 18 which then underwent stepwise elimination to afford indolizines 20 (Scheme 2).14 The isolation of some non-aromatic compounds 1814b,c and 1914a has been also described. Similarly, the reactions of 3 with electron poor alkynes such as dialkyl
acetylenedicarboxylates and methyl phenylpropiolates, and with benzyne provided the corresponding indolizine and benzo[a]indolizine derivatives 20 via the smooth dehydrogenation of the primary 3,8a-dihydroindolizines 21.15 Pyridinium (1,1-disubstituted)methylides 22 can be also used in such cycloadditions and their reactions with the same dipolarophiles provided the corresponding indolizine derivatives 20 with the elimination of one electron-withdrawing group at the 3-position.16 Pyridinium dicyanomethylides (4) have been often employed as useful substrates for the synthesis of indolizine-3-carbonitriles (20 (R1=CN)) because of smooth elimination of a hydrogen cyanide from primary bicycloadducts 23 (EGW1=EGW2=CN).11,17
In contrast with pyridinium methylides 3 and 22, the 1,3-dipolar cycloadditions of aminides 12 and 13 with olefinic compounds were scarcely reported due to the high nucleophilicity of the anionic nitrogen atom which will be described later. However, the reactions of 12 with acetylenic compounds such as acetylenedicarboxylates and propiolates18 or with nitriles19 proceeded smoothly to give the corresponding pyrazolo[1,5-a]pyridines 26 or 1,2,4-triazolo[1,5-a]pyridines 28 via the aromatization of the resulting 1,3a-dihydro-adducts 25 or 27 respectively (Scheme 3).
As in the case of pyridinium (1,1-disubstituted)methylides 22, 1,3-dipolar cycloadditions of (2-unsubstituted)pyridinium (ethoxycarbonyl)aminides 29 with DMAD afforded the corresponding dimethyl pyrazolo[1,5-a]pyridine-2,3-dicarboxylates (31), but their yields were very low due to the difficulty in eliminating the N-ethoxycarbonyl group from adducts 30 (see Scheme 4). On the other hand, the reactions of 2-methylpyridinium acylaminides (32) with the same reagent gave some unstable primary adducts, 3a-methyl-1,3a-dihydropyrazolo[1,5-a]pyridine derivatives (33), which were smoothly converted to 3-vinylpyridines 34 and/or 5-vinylpyridines 35.20 Similar rearrangement was also observed in the reaction of 2,6-dimethylpyridinium vinylaminides such as 39 with ethyl propiolate.21 The proposed mechanism for this rearrangement was first N−N bond fission of adducts 33, the 1,2- and 1,4-shifts of the aza-allyl anion moiety onto the aza-arenium ring to give 37 and 38 respectively, and the 1,4-shift (σ2s + π4s) of a hydrogen at the 3- or 5-position of the pyridine ring to the nitrogen anion atom. Since this cationic 1,2-shift (ω0s + σ2s) is a symmetry allowed process in ground state (GS) but the 1,4-shift (π2s + σ2s) is not so, the latter process should be three sequences of such 1,2-shifts. However, the driving force and the preferred 3- and 5-positions on the pyridine ring in this rearrangement could not be well realized at that time. The molecular calculation (MOPAC-PM3) using model 40 made the following stability order (38>37>>41⋍42>>36) clear, that is, the contributors such as 36, 41, and 42 in which the positive charge is localized on the electronegative hetero atom (nitrogen) are unstabilized.22
Photochemical 1,3-dipolar cyclization of pyridinium ylides were first found by Streith et al.23 They reported the formation of 2-(2,2-dicyanovinyl)pyrrole (45) in low yield from the irradiation of parent pyridinium dicyanomethylide (4) in benzene and proposed a reaction sequence in which the photochemical disrotatory cyclization (π4s) of 4, the disrotatory ring enlargement (π4s + s2s) of the resulting 1-azanorcaradiene 43 in the ground state, and the ring contraction-rearrangement of 2,2-dicyano-2H-azepine (44) was involved.
J. Streith et al. extended this photolysis to some pyridinium acylaminides (13) and found their simpler and more effective transformation to 1(1H),2-diazepine derivatives.24 By the ensuing research of Sasaki25 and Snieckus groups26 the wide scope of this photolysis was established and various reactions of 1(1H),2-diazepines obtained thus with iron pentacabonyl,24 tetracyanoethylene,25 ketenes,27 nitrile oxides,28 and diazoisopropane29 to give compounds 48−52 were also investigated (Scheme 6).
Interestingly, Tsuchiya et al. reported the formation of N-unsubstituted 1(1H),2-benzodiazepine derivatives 56 by the irradiation of quinolinium (unsubstituted)aminides (54) generated in situ from the dimer 5330 (Scheme 7).
The 1,3-dipoles conjugated with an unsaturated double or triple bond are defined as 1,5-dipoles.31 The 1,5-dipolar cyclization of such molecules are useful methods for synthesizing various five-membered heterocycles because of the smooth and symmetry allowed process (π6s) in ground state. Theoretically there are two types of pyridinium ylides which can act as a 1,5-dipole: a) pyridinium ylides IV having a double bond conjugated with the ylidic carbanion atom and b) pyridinium ylides V or V’ having a double or triple bond at the 2-position on the pyridine ring (Figure 3).
Both types of pyridinium ylides have been synthesized and their reactivity has also been investigated. The first example for the 1,5-dipolar cyclization of type IV pyridinium ylides was reported by Kröhnke et al.32 in 1966. They examined the reactions of pyridinium phenacylides (3) with picryl chloride in the presence of a base and demonstrated the formation of benz[a]indolizine derivatives 59 by way of the cyclization of 57 in this mode and the subsequent elimination of nitrous acid from the tricycloadducts 58 (Scheme 8).
Pyridinium (2,3-disubstituted) allylides (60), readily available from the alkaline treatment of the corresponding pyridinium salts 2 (R1=vinyl group), underwent the expected 1,5-cyclization, followed by the dehydrogenation of primary 1,8a-dihydroadducts 61 at comparative low temperatures to yield the corresponding 3-unsubstituted indolizine derivatives 62 (See Scheme 9).33 Stabilized pyridinium (1,2,3- or 1,3,3-trisubstituted)allylides (63) also underwent such cyclization under more severe reaction conditions.6e In contrast, pyridinium (3-substituted)- and (3,3-disubstituted)allylides (65) did not form the intramolecular mode of adducts, but they intermolecularly reacted with the carbon-carbon double bond in another molecule or with other dipolarophiles to afford 3-vinylindolizine derivatives.33c,34
Similar 1,5-dipolar cyclizations for pyridinium vinylaminides have been reported by some investigators. For example, Tamura et al. examined the reactions of pyridinium (1-oxo-2-cyclohexen-3-yl)aminides (66) in toluene at the reflux temperature and found the formation of the expected pyrido[1,2-b]indazoles (Scheme 10).6a,33a
Sasaki et al. also studied the 1,5-dipolar cyclization of the Z-isomers 69 of pyridinium [1,2-bis(methoxycarbonyl)vinyl)]aminides in chloroform at room temperature and obtained the primary adducts, 3,3a-dihydropyrazolo[1,5-a]pyridines (cis-71) which were very unstable and readily oxidized to pyrazolo[1,5-a]pyridines 26 upon exposure to air (Scheme 11).6b From the facts that the precursors of pyrazolo[1,5-a]pyridines 26 were cis-71 but not trans-71 and the mode in a 1,5-dipolar cyclization in the ground state is disrotatory (dis), they presumed the reaction mechanisms to be as follows: 1) the cis-trans isomerization of the vinyl group of the aminides 69, 2) the smooth cyclization of the resulting E-isomers 70, and 3) the dehydrogenation of the cycloadducts cis-71 to aromatic pyrazolo[1,5-a]pyridines 26.
We examined the thermolyses of pyridinium (N-acylimidoyl)aminides (15) in xylene at the reflux conditions to obtain 1,2,4-triazolo[1,5-a]pyridines (28) which were derived from their 1,5-dipolar cyclization, though other products, pyrido[6,1-f]-as-triazinium-4-olates 73, were also given (Scheme 12).7
On the other hand, a 1,5-dipolar cyclization of type V pyridinium ylides was reported first by Mörler and Kröhnke.35 They investigated the alkaline treatment of 1-(acylmethyl)pyridinium salts 74 having a 4-piperidino-1,3-butadien-1-yl group at the 2-position to obtain 3-acyl-2-vinylindolizine derivatives 78 via the generation of pyridinium methylides 75, their 1,5-dipolar cyclization to 2,3-dihydroindolizines 76, the 1,3-shift of the 2-proton onto the vinyl group, and the 1,4-elimination of a molecule of piperidine from 77. (See Scheme 13) Similarly, Kobayashi et al. reported the syntheses of 2-methylthio-3-acylindolizines or imidazo[1,5-a]pyridines 82 by way of the 1,5-dipolar cyclization-aromatization route of pyridinium methylides 80, which were generated in situ from the alkaline treatment of pyridinium salts 79 possessing a 2,2-bis(methylthio)vinyl or bis(methylthio)methyleneamino group at the 2-position.36
Tsuchiya et al. examined a cyclization of type V’ pyridinium ylides and found the smooth transformations of 2-alkynylpyridinium methylides or aminides 84, generated in situ from the alkaline treatment of the corresponding pyridinium salts 83, to 1-unsubstituted indolizines or 3-unsubstituted pyrazolo[1,5-a]pyridines such as 86 via the 1,5-dipolar cyclization-proton shift (Scheme 14).37
An electrocyclic reaction of more extended dipoles has also been known. Marx and Eberbach described a thermal and exclusive 1,7-dipolar cyclization (π8a) of pyridinium 1,3-pentadien-1-yl-5-ides (88) to provide the corresponding 5,5a-dihydropyrido[1,2-a]azepines (89) (Scheme 15).38 This procedure is very interesting from the standpoint of the synthesis of medium-sized nitrogen-containing heterocycles, since, in systems in which the different types of reactions by the other contributors are also possible, the 1,5-dipolar cyclization (5-exo-trig) of contributors 88’ is usually more favorable than the 1,7-dipolar one (7-endo-trig) as shown in the cyclization from 75 to 76 in Scheme 13. The cation-anion interaction may work effectively in this 1,7-dipole system.
3.2. REACTIONS AS NUCLEOPHILES
It has been known that the anionic atom of pyridinium ylides acts as a nucleophilc center. One of the title compounds, pyridinium salts, can be readily obtained by this method. For example, 1,3-dipoles 91 was alkylated by various alkyl halides and dialkyl sulfates to give the corresponding salts 92, while similar alkylations of 1,5-dipoles 91’ afforded pyridinium salts 93 having a vinyl or methyleneamino group at the 1-position (See Scheme 16). However, attempts for the O-alkylations in the 1,5-dipole forms of pyridinium 1,1-diacylmethylides or acylaminides by the same reagents were usually unsuccessful because of the presence of its faster dealkylation process.39
Beside their dipolar cycloadditions and cyclizations, the nucleophilic reactions between pyridinium methylides or aminides and electron poor alkenes, which afford the alkyl- and amino-substituted alkenes, cyclopropanes, enamines, and aziridines with the elimination of pyridine, have also been described. These are of practical and preparative importance, since usual synthetic routes for such compounds consist of the addition of elctrophilic species such as alkyl halides, carbenes, and nitrenes to electron-rich alkenes and their species can not usually react to electron-poor alkenes and alkynes. In 1961 Zecher and Kröhnke developed a new preparative method of tri- and tetra-substituted pyridine derivatives 96 from the reactions of pyridinium phenacylides 3 with certain α,β-unsaturated ketones in the presence of ammonium aetate40 (See Scheme 17). They suggested the reaction mechanisms which proceeded by way of first Michael addition of the carbanion of methylide 3 to the β-position of the α,β-unsaturated ketone, the 1,2-hydride shift of the resulting adduct 94 with the elimination of pyridine and the regeneration of the double bond, and final condensation between enedione 95 and ammonium acetate with the loss of water and acetic acid. The formation of 2-aryl-1-azaazulenes 98 from similar condensations of 3 with tropones and ammonium acetate was also reported.41 Some of the initially formed monoalkylated products such as 95 in these reactions have been isolated.41,42
On the other hand, the use of (benzylidene)benzoylacetates in place of α,β-unsaturated ketone in this reaction afforded different type of products. Chuang and Tsai reported an effective and stereoselective synthesis of 2,3-dihydrofuran derivatives 100, which were obtained from the reactions of pyridinium methylides 3 with ethyl (benzylidene)benzoylacetates via the formation of the primary adducts 99, followed by the intramolecular nucleophilic addition of the anionic oxygen atom to the carbon atom having the pyridinio group in the same molecule 99’ with the loss of pyridine (Scheme 18).43
Though an enamine synthesis from the nucleophilic reactions of pyridinium aminides and electron-poor olefins did not usually afford good results, it was indicated that the activation of such olefins by an acidic catalyst promoted the formation. For example, Sasaki et al. reported facile formation of the corresponding enamine derivatives 102, 103, 105, 106 from the reactions of pyridinium (ethoxycarbonyl)aminide (13) with maleic anhydride, N-phenylmaleimide, p-benzoquinone, and 1,4-naphthoquinone in the presence of silicic acid (silica gel) at room temperature (Scheme 19).44 Similar reactions of 13 with dimethyl maleate or fumarate under more severe conditions gave a mixture of dimethyl 2-(ethoxycarbonylamino)fumarate carbamate (107) and dimethyl 2-aminofumarate (108), while that with tropone yielded only 2-aminotropone 110. The primary amino compound 108 and 110 must be formed by the hydrolysis of the initially prepared carbamates 107 and 109 respectively under the reaction conditions employed here.
The formation of cyclopropane derivatives from the reactions of S-ylides with Michael acceptors have been well known.45 Useful and enantioselective cyclopropanation reactions of pyridinium methylides (3) and electron-poor alkenes have also been observed by some investigators.46 Two examples of them are shown in Scheme 20.
In general, it is well known that aziridine is considerably unstable and easily undergoes the ring opening reaction or the decomposition under thermal and acidic reaction conditions. Hence, the formation of aziridine derivatives from the nucleophilic reactions between pyridinium aminides and electron-poor
alkenes was scarcely observed. However, the reactions in which the intermediacy of the 2-haloaziridine or 2H-azirine was strongly suggested were found by our laboratory.47 The reactions were performed by employing two moles of pyridinium (unsubstituted)aminides 12 and one mole of ethyl 2-chlorocinnamate or methyl 2-bromocrotonate to provide 1,9a-dihydro-2H-pyrido[1,2-b]-as-triazines (119) (Scheme 21). We thought that this reaction proceeded via the first nucleophilic addition of the aminide nitrogen atom of 12 onto the β-carbon of the α-haloacrylate, the formation of 2-haloaziridine 115 from the resultingzwitterion 114 with the elimination of pyridine, the nucleophilic replacement of 115 with an another molecule of 12, the ring opening of the resulting pyridinium (2-aziridinyl)aminide 117, and the
1,6-cyclization of zwitterion 118 to the final products 119. The intermediate 117 may also be formed by the nucleophilic addition of 12 to 2H-azirine 116 which is generated by the dehydrohalogenation of 115 under the alkaline conditions employed here. According to this mechanistic assumption we examined the reactions of various pyridinium aminides 12 with 2H-azirines 120, which were isolated from the thermolysis of α-azidostyrene or generated in situ by modified Neber reaction of alkyl aryl ketone N,N-dimethylhydrazone methiodides, to develop a novel preparative method for 1,9a-dihydro-2H-pyrido[1,2-b]-as-triazine derivatives 122 (Scheme 22).48
The reactions of pyridinium ylides with diphenylcyclopropenone are one of the most interesting themes in this field. Diphenylcyclopropenone is a very reactive molecule owing to the severe ring strain and behaves as a strong dienophile or dipolarophile. However, Eicher and Hansen first investigated the reactions of pyridinium acylmethylide 3 with diphenylcyclopropenone in 1969 to find the formation of 3,4-diphenyl-2-pyrone derivatives 125 in place of the expected 1,3-dipolar adducts49 (See Scheme 23). Furthermore, Sasaki et al. reported the syntheses of 2-pyrone 125 and 1,3-oxazin-6-one derivatives 126 from the reactions between pyridinium ylides 3 and 13 with the same reagent.50 Although this reaction mechanism is still not clear because of the high reactivity of the ketone carbonyl group in diphenylcyclopropenone, the reliable route at present may be one started by the first nucleophilic attack of the ylidic anion atom of ylides 3 or 13 onto the carbon-carbon double bond of diphenylcyclopropenone. The extension of this type of reaction to 1,2-diphenylcyclopropene-3-thione, and 1,2-diphenyl-3- methylenecyclopropene were also documented.51
It can be easily realized that the development of any ring formation reaction between a 1,5-dipole IVc (6π) and a 2π component leads to a useful route to unsaturated seven-membered heterocycles (VIIa or VIIb) which are not readily available by other methods (See Figure 4). However, the geometrically favorable mode (π6s + π2s) of a 1,7-dipolar cycloaddition is forbidden in the ground state, and the concerted process (π6s + π2a or π6a + π2s) is unfavorable owing to severe geometrical restrictions in the transition state. On the other hand, both the nucleophilic addition (ω2s + π2a) of a 1,5-dipole IVc to a double or triple bond and the 1,7-cyclization (ω0s + ω2s) of the resulting zwitterion VIb or VIIIb are symmetry allowed processes though the rotation or inversion of the anion moiety in the initially generated zwitterion VIa or VIIIa is required.
After some research for pyridinium ylides which can act as a 1,5-dipole we found first formation reactions of various pyrido[1,2-d]-1,4-thiazepine derivatives.52 For example, the reactions of pyridinium (2-alkylthio-1-benzoyl-2-thioxo)- (R1=COPh) and pyridinium (2-alkylthio-1-cyano-2-thioxo)ethanides 6 (R1=CN) with DMAD in CHCl3 at room temperature afforded the corresponding dimethyl 10aH-5-benzoyl- and 10aH-5-cyano-4-(alkylthio)pyrido[1,2-d]-1,4-thiazepine-1,2-dicarboxylates (127) in moderate yields (See Scheme 24). Similarly, those of 3-(1-pyridinio)thiophene-2-thiolates (8) and isoquinolinium 2-(thioxo)ethanides (129) with the same reagent provided the tricyclic 1,4-thiazepine derivatives, pyrido[1’,2’-d]thieno[2,3-b]-1,4-thiazepines (128) and 1,4-thiazepino[5,4-a]isoquinolines (130), respectively.
Interestingly, the reactions of pyridinium (2-alkylthio-1-ethoxycarbonyl-2-thioxo)ethanides 6’ with DMAD under the same reaction conditions did not provide the initially expected 10aH-pyrido[1,2-d]-1,4-thiazepines 127’, but, instead of them, gave their intramolecular Diels-Alder adducts, ethyl 5,6-dimethyl 4-thia-1-azatetracyclo[5.4.0.05,11.06,8]undeca-2,9-diene-5,6-tricarboxylates
(131)53 (See Scheme 25). Similar types of adducts 131 and 132 were also obtained by heating 10aH-pyrido[1,2-d]-1,4-thiazepines 127 and 128 or the reactions of pyridinium methylides 6 (R1=COPh and CN) and 3-(1-pyridinio)thiophene-2-thiolates (8) with DMAD in refluxing CHCl3.53
That this reaction proceeded via the nucleophilic addition―1,7-cyclization route could be proven by the reactions of pyridinium (2-alkylthio-1-cyano)ethanides (6) with DMAD in the presence of an alcohol, in which dimethyl 2-alkoxy-4-cycano-6-thia-3-azatricyclo[5.3.1.03,8]undeca-4,9-diene-7,11-dicarboxylates (136) were formed by way of intramolecular Diels-Alder reaction of the adducts 135’ derived from the key intermediates 126 and alcohols (Scheme 26).54
Recently we could isolate the unexpected products, thiino[3’,4’:4,5]oxazolo[1,3-b]-1,3-oxazepine derivatives 137, from the reactions of pyridinium (1-alkylthio-1-benzoyl-2-thioxo)ethanides 6 with DMAD in tetrahydrofuran involving its peroxide (THF peroxide) at the reflux temperature.55 Though this reaction mechanism is still unclear (See Scheme 27), the high participation between the intermediate 140, which was generated from the addition of pyridinium betaine 6” to DMAD, followed by the successive cyclizations of zwitterion 138, and the oxidant was suspected.
We have also reported some interesting reactions which proceeded through 10aH-pyrido[1,2-d]-1,4-thiazepines 127 and 128 and 12bH-1,4-thiazepino[5,4-a]isoquinolines 130 obtained or generated thus (See Scheme 28). For example, the thermolyses of the intramolecular Diels-Alder adducts 131 and 132 obtained from the corresponding 1,4-thiazepines 127 and 128 lead to the smooth formation of thiazole 142 and thieno[3,2-d]thiazole derivatives 143 by way of the retro Diels-Alder reaction and the fragmentation of the resulting benzo[f]-1,4-thiazepines such as 141.53b,56 On the other hand, the thermolyses of 1,4-thiazepino[5,4-a]isoquinolines 130, which could not convert to the corresponding intramolecular Diels-Alder adducts due to the fused benzene ring, in xylene at the reflux temperature afforded 1-(1,2-dihydrothiophen-2-yl)isoquinolines 144 via the ring contraction- rearrangement route, while those in the presence of base provided the ring contraction-elimination products, pyrrolo[2,1-a]isoquinolines 145.57
Furthermore, we found two different types of rearrangements in reactions of one thiophene-fused pyridothiazepine derivative.58 The reaction of 9,10-dimethyl 2-ethyl 7-methyl-3-phenyl-8aH- pyrido[1,2-d]thieno[2’,3’-b]-1,4-thiazepine-2,9,10-tricarboxylate (128) in THF at the reflux temperature yielded 6,8-dithia-2-azatetracyclo[7.3.2.02,10.03,7]tetradeca-Δ3,7,4,11,13-tetraene 147, while that in CHCl3 10H-pyrrolo[1,2-d]thieno[2’,3’-b]-1,4-thiazocine 148. Product 147 can be formed from the cyclization between the 5- and 9-positions in 128, followed by the cationic 1,2-shift (ω0s+σ2s) of a carbon-nitrogen bond of the resulting zwitterion 146 with the regeneration of a carbon-carbon double bond. On the other hand, the formation mechanism for 1,4-thiazocine 148 is unclear, because a route starting from compound 147 in which a 2,5-dihydropyrrole skeleton is present was refused. The thermal conversion from the latter product 148 to dimethyl (Z)- and (E)-2-[thieno[2’,3’:2,3][1,4]thiazino[4,5-a]pyrrol-8-ylidene]succinates 151 was shown (Scheme 29).
The reactions of pyridinium and 4-methylpyridinium (arylthiocarbonyl)aminides (16) with DMAD in dry CHCl3 at 50−60 ºC did not give any 5aH-pyrido[1,2-d]-1,3,4-thiadiazepine derivatives 153 and their intramolecular Diels-Alder adducts 154, but, instead of them, provided the rearranged products, 4-aryl-5-thia-2,3-diazatricyclo[4.3.2.02,7]undeca-3,8,10-trienes (156), in low yields via similar reaction mechanisms proposed for the transformation from 128 to 147 in Scheme 2959 (See Scheme 30). On the other hand, the reactions of 3-methylpyridinium (arylthiocarbonyl)aminides (16, R3=H, R4=Me) with DMAD formed the expected dimethyl 2-aryl-6-methyl-5aH-pyrido[1,2-d]-1,3,4-thiadiazepine-4,5- dicarboxylates (153’), together with dimethyl 4-aryl-8-methyl-5-thia-2,3-diazatricyclo[4.3.2.02,7]undeca- 3,8,10-triene-6,11-dicarboxylates (156). Products 156 were derived from the 8-methyl-5aH-pyrido- [1,2-d]-1,3,4-thiadiazepines (153, R3=H, R4=Me). This fact strongly suggested the large effect of the 6-methyl group on the stabilization of pyridothiadiazepine structure. Similarly, 5a-methyl-1,3,4- thiadiazepino[4,5-a]quinolines (158) were isolated in moderate yields from the reactions of 2-methylquinolinium (arylthiocarbonyl)aminides (157) with DMAD.
The reason why 5aH-pyrido[1,2-d]-1,3,4-thiadiazepine derivatives 153 did not afford the corresponding intramolecular Diels-Alder adducts but, instead of them, gave the rearranged products, 5-thia-2,3-diazatricyclo[4.3.2.02,7]undeca-3,8,10-trienes 155, is unclear. However, the introduction of shorter carbon-nitrogen and nitrogen-nitrogen bonds than the carbon-carbon one should cause the larger ring strain in the heterocyclic Diels-Alder adducts 154 possessing a cyclopropane ring than the rearranged tricyclic products 156.
3.3. REACTIONS AS ELECTROPHILES
The attack of external nucleophiles onto the 2- or 4-position of the pyridinium ring of pyridinium ylides has been scarcely known. However, a few intramolecular versions of this type of reaction were reported. In 1975 Kato and Masuda described the formation of 3-acetylpyrazolo[1,5-a]pyridin-2-ols (161) by treating pyridinium (acetoacetyl)aminides (13, R2=CH2COMe) with a strong base60 (See Scheme 31). Products 161 were formed by way of the deprotonation from the active methylene group of 13, the intramolecular cyclization between the resulting anion terminal and the positive 2-position of 159, and the elimination of a hydride and the keto-enol isomerization of 160. Similarly, we investigated the thermolyses of pyridinium (thiocyanatoacetyl)aminides (13, R2=CH(R3)SCN) in the presence of a strong base to obtain 1,3,4-thiadiazolo[3,2-a]pyridinium 2-(N-acetyl)imides (166), though their yields were low.10b By considering the possible reaction mechanisms and examining the independent synthesis of key intermediates 164 starting from 1-amino-2-chloropyridinium salt (11) we could develop a novel and more effective synthetic method for this type of compound 166.
3.4. YLIDIC BOND FISSION
The cleavage of the ylidic bond is a phenomenon observed well in various thermal and photochemical reactions of pyridinium ylides. Since pyridinium ylides are not explosive, ylidic bond fission is a useful method for generating reactive carbene and nitrene species safely. The carbene and nitrene formed thus are electrophilic and react with electron-rich substrates to provide cyclopropane and aziridine derivatives or intramolecularly cyclize to give some heterocycles. For example, the photolysis of pyridinium dicyanomethylide (4) or pyridinium (ethoxycarbonyl)aminide (13) in benzene afforded 7,7-dicyanobicyclo[4.1.0]hepta-2,4-diene (170)23 or ethyl N-phenylcarbamate (172)25b as a by-product together with each original product described earlier. It can be thought that compound 172 was formed by the isomerization of initially formed N-ethoxycarbonyl-7-azabicyclo[4.1.0]hepta-2,4-diene (171). On the other hand, the irradiation of pyridinium [(3-oxo-1-butenyl)]aminides (14)6d and pyridinium [(N-acyl)benzimidoyl]aminides (15)7b gave the corresponding 4-acyl-5-methylisoxazoles (174) and 5-acyl-3-phenyl-1,2,4-oxadiazoles (175) in good yields with the loss of pyridine respectively (Scheme 32). The thermolysis of ylide 15 (R3=Ph) in xylene at the reflux temperature also provided the same compound 175 (R3=Ph).7b
We investigated the substituent effect which influenced ylidic bond fission and found a large promotion by the 2,6-dimethyl groups on the pyridine ring (Scheme 33).61 The thermolyses of 2,6-dimethyl- pyridinium (2-alkoxycarbonyl-2-phenylvinyl)aminides (14) smoothly afforded indole-3-carboxylates (179), phenylcyanoacetates (181), phenylacetates (183), and 4-phenyloxazoles (186), which were derived from the vinyl nitrenes 176 or the related 2-phenyl-2H-azirine-2-carboxylates (177), and their photolysis
gave 2H-azirine 177, isonitriles 184, and 8-methyl-3-phenylpyrido[1,2-b]pyridazinium-4-olate (187). The last compound 187 was formed by way of the first photochemical cis-trans isomerization of the vinyl group of ylides 14, followed by the intramolecular cyclization-elimination of the resulting 14’. On the other hand, the thermolysis and photolysis of parent pyridinium ylide 14” did not provide any distinct product.
3.5. OTHER REACTIONS
Two reactions for pyridinium ylides except those described above have been sometimes observed. The first is a smooth hydrogen shift from the 2-methyl group to the terminal anion atom of the dipole. For example, zwitterion 188 generated from the hydrogen shift of 2-methylpyridinium 1,3,3-tris(ethoxycarbonyl)allylide (5) undergoes the intramolecular Michael addition to form 1H-2,3-dihydroindolizine intermediates 189, which in turn proceeds to ethyl indolizine-3-carboxylate (191) via its aromatization with the elimination of diethyl malonate33 (See Scheme 34). Similarly, that of 2-methylpyridinium [N-(ethoxycarbonyl)benzimidoyl]aminide (15) proceeded via the hydrogen shift to the 3-nitrogen atom, the nucleophilic cyclization of 192 to 193, and the final aromatization to 2-phenylpyrazolo[1,5-a]pyridine (194).7
The second is the 1,6-cyclization reaction of 1,5-dipoles having an alkoxycarbonyl group at the anion terminal, and it affords the corresponding 6-membered mesoionic compound (see Scheme 12 and 33 for two examples). This reaction has been known to some extent in the field of azolium ylides and the formation of the mesoionic compounds was elucidated by the increased nucleophilicity at the 2-position of the azole ring caused by the back donation.62 However, the large contribution of such a back donation as seen in 195’ is not able to expect so much to the π-deficient pyridine ring, and hence an alternative route such as the 1,6-cyclization (π6s) of the hetero-triene system 195” may be considered (Scheme 35).
4. REACTIONS OF PYRIDINIUM SALTS
4.1. ELECTROPHLIC REACTIONS OF PYRIDIUM RING
It has been reported that 1-(disubstituted)aminopyridinium salt 197, readily available from the alkylation of pyridinium acetylaminide 13 (R2=Me) with alkyl halides, reacted with a cyanide ion to provide 4- (200) and 2-cyanopyridines (201) in good yields via the aromatization of the primary adducts, 4-cyano-1,4-dihydropyridine 198 and 2-cyano-1,2-dihydropyridine 199, with the loss of an amide (Scheme 36).63 However, the synthetic value of this type of reaction is not too high, because similar reactions can be performed by using more readily available materials such as pyridine N-oxides.64
The ring opening in the reactions of 1-vinylpyridinium salts 202 and some nucleophiles under alkaline conditions have been observed. For example, Aklbrecht and Kröhnke reported the formation of 3-aza-1,3,5,7-octatetraenes 204 from the reactions of salts 202 and amines such as piperidine and cyclohexylamine65 and Kobayashi et al. described those of N-vinyl-1,3,5-hexatrienylamines 207 from their reactions with active methylene compounds (Scheme 37).66 These products 204 and 207 should be obtained via the electrocyclic ring opening (π4s + σ2s) of the first adducts 203 or 205 between salts 202 and amine or the carbanion generated from the deprotonation of active methylene compounds, followed by the cis-trans isomerization of 204’ or a hydrogen shift of 206.
Kato reported the synthesis of 3-acetyl-1-methyl-1,2-dihydropyrazol[1,5-a]pyridine-2-ones (211) from the alkaline treatment of 1-[acetoacetyl(methyl)amino]pyridinium iodide (208), which was obtained from the reaction of pyridinium (acetoacetyl)aminide (13) with iodomethane60 (See Scheme 38). Compound 211 can be formed via the dehydriodination of salt 208, the 1,5-cyclization of the resulting zwitterion 209, and the dehydrogenation of cycloadduct 210. Later, our groups also described a more common synthesis of 1-alkyl-1,2-dihydropyrazol[1,5-a]pyridin-2-one derivatives 212.10a
As described in Section 2, pyridinium ylides 6, 7, 16 and 17 having a thiocarbonyl group on the ylidic atom always undergo the alkylation at the sulfur atom. When alkyl halides such as bromoacetonitrile, α-haloesters, α-haloketones and benzyl halides having an electron-poor group are employed as an alkylating agent in these reactions, the resulting pyridinium salts 213 and 214 have an active methylene group in the 1-substituent and their treatment with an appropriate base can generate new zwitterions 215 and 216 (Scheme 39).
We developed a quite novel synthetic method for indolizines 20 and pyrazolo[1,5-a]pyridines 26 through the investigation of the generation and the reaction of zwitterions 215 and 216 (Scheme 40).67 In these reactions the generated zwitterions 215 and 216 undergo 1,6-cyclization to afford the corresponding 1,9a-dihydropyrido[2,1-c]-1,4-thiazine 217 or 1,9a-dihydropyrido[1,2-d]-1,3,4-thiadiazine intermediates 218 which can be isolated or detected by NMR spectral inspection. The treatment of 217 and 218 with a dehydrogenating agent at low temperature (0 oC) generates pyrido[2,1-c]-1,4-thiazines 219 or pyrido[1,2-d]-1,3,4-thiadiazines 220 and they are spontaneously transformed by way of the ring contraction-desulfurization (R3=CN, CO2R5, and Ar) or ring contraction-rearrangement (R3=COAr) to the corresponding indolizines 20 and pyrazolo[1,5-a]pyridines 26.
The smooth desulfurization of the related species, 1,3,4-thiadiazinyl anion, is described, but the rearrangement of an arylcarbonyl group onto the sulfur atom has no precedent.68 This method is superior to traditional ones in the introduction of a wide range of the 2-substituent such as alkyl, aryl, alkylthio, alkoxy, and alkylamino groups. Although most reactions proceeded via the ring contraction- rearrangement route when R3=COAr, an exceptional ring contraction-desulfurization one was also observed in the reactions with 1-[2-alkylthio-2-aroylmethylthio-1-(ethoxycarbonyl)vinyl]-3,5- dimethypyridinium salts 213’ (Scheme 41).69
These bicyclic key intermediates 219 and 220 have an unstable anti-aromatic 12π system and therefore could not be isolated and detected at all. However, pyrido[2,1-c]thieno[3,2-e]-1,4-thiazines (224) fused with an aromatic thiophene ring were very stable under the reaction conditions employed here, though only the 6,8-dimethyl derivatives were slowly converted to the corresponding thieno[2,3-b]indolizines 225 (Scheme 42).70
Indolizines obtained thus are useful precursors for synthesizing tricyclic analogs fused with a hetero-ring. For example, thieno[3,2-a]indolizine 226 and thieno[2,3-b]indolizine derivatives 228 were prepared by the alkaline treatment of 2-(ethoxycarbonylmethylthio)indolizines 227 bearing a cyano or ethoxycarbonyl group at the 1- or 3-position,71 and 1,4-dihydropyrido[2,3-b]indolizin-4-ones 231 were formed via the N-formylation of 3-acetyl-2-(alkylamino)indolizines 229 with triethyl orthoformate-acetic anhydride in the presence of a catalyst and the dehydration of the resulting compounds 230 under strong alkaline conditions (Scheme 43).72
Since the introduction of the 2-substituent involving an active methylene group by this method was considerably restricted, however, we developed a novel procedure for synthesizing polyfunctionalized
indolizines 236 and pyrazolo[1,5-a]pyridines 237 having various acylmethylthio groups at the 2-position.
The retro-Michael addition of compounds 232 and 233 bearing a 2-cyanoethylthio or 2-(ethoxycarbonyl)ethylthio group by the treatment with a strong base (t-BuOK) in DMF and then the S-alkylation of the resulting indolizine-2-thiolates 234 and pyrazolo[1,5-a]pyridine-2-thiolates 235 with α-haloacetates and α-haloketones afforded the corresponding 2-(acylmethylthio)indolizines 236 and 2-(acylmethylthio)pyrazolo[1,5-a]pyridines 237.73
The reactions of thiolates 234 and 235 with isocyanates and isothiocyanates afforded 1,3-thiazino[6,5-b]indolizines 238,74 and those with DMAD, diethyl acetylenedicarboxylate (DEAD), ethyl propiolate (EP), yielded the Michael adducts 239 and 241 and/or thiazino[2,3-b]indolizin-4-ones 240 depending upon the reaction conditions used.75 Similarly, the S-alkylation of 1,3-bis(ethoxycarbonyl)indolizine-2-thiolates (234) with ethyl 4-bromocrotonate and subsequent alkaline treatment of the resulting indolizine derivatives gave thiepino[2,3-b]- 242 and thiepino[3,2-a]indolizine 243.76 The functionalized indolizines 236 were transformed in the presence of a base to the corresponding thieno[2,3-b]indolizines 244 or thieno[3,2-a]indolizines 245 depending upon the substituents at the 1- and 3-positions, and 2-(acylmethylthio)pyrazolo[1,5-a]pyridine-3-carbonitriles 237 were similarly converted to thieno[2’,3’:2,3]pyrazolo[1,5-a]pyridines 246.77 These results are summarized in Scheme 44.
In the attempts to synthesize 2-benzylthio-3-(2-ethoxycarbonylvinyl)indolizine-1-carbonitrile (248) from the EZ-mixtures of 1-(1-benzylthio-1-cyanomethylthio-4-ethoxycarbonyl-1,3-butadien-2-yl)pyridinium bromides (247) we noticed the formation of the byproducts, ethyl 3-benzylthio-1-cyanothieno- [3,4-b]indolizine-9-carboxylates (249), in low yields together with the target compounds.78 We assumed that compounds 249 were formed by way of the Michael addition (MA) of the carbanion atom of 250 generated by the dehydrobromination of the Z-isomers of salts 247, the aromatization of dihydrothiophenes 251, the 1,5-cyclization of zwitterions 252, and the dehydrogenation of 8a,9-dihydrothieno[2,3-b]indolizine intermediates 253 (Scheme 45).
To confirm this assumption we planned two independent syntheses of thieno[2,3-b]indolizines such as 256 (Scheme 46). The first route (method A) involved the generation of the common key intermediates 252’ by the dehydrobromination and the dehydration of 1-[2-alkylthio-2-arylcarbonylmethylthio-1- (ethoxycarbonylacetyl)vinyl]pyridinium bromides (254) in the presence of a base,79 and the second (method B) can also form the same intermediates 252’ by the dehydrohalogenation of 1-[2-alkylthio-5-arylcarbonyl-4-(ethoxycarbonylmethyl)thiophen-3-yl]pyridinium halides (255), which were readily available from the S-alkylation of 3-(1-pyridinio)thiophene-2-thiolates (9) with various alkylating agents.80 The experimental results made the high effectiveness of method B clear. The thieno[2,3-b]indolizine molecules 256 having an arylmethylthio, allylthio, propargylthio, or acylmethylthio group at the 3-position exhibited an interesting arene-arene, arene-π, or arene-cation interaction in their gauche conformation in the relation of the sulfide linkage.81
Similarly, we found that the alkaline treatment of 1-[2-alkylthio-4-amino-5-(arylcarbonyl)thiophen- 3-yl]pyridinium halides (257), readily available from 4-amino-3-(1-pyridinio)thiophene-2-thiolates (10) and alkyl halides, smoothly generated the corresponding 3-(1-pyridinio)thiophen-4-imides 258 and then their 1,5-cyclization and the aromatization afforded thieno[3’,4’:4,5]imidazo[1,2-a]pyridine derivatives 259 (Scheme 47).82
The smooth deprotonation from an aromatic primary amino group described above encouraged us to extend this type of reaction to pyridinium salts bearing a carbamoyl group in the 1-substituent. As expected, the treatment of 1-[1-carbamoyl-2,2-bis(methylthio)vinyl]pyridinium iodides (260), prepared from the reactions of pyridinium methylides 6 (R1=CONH2) with iodomethane, with a strong base afforded 3-[bis(methylthio)methylene-2(3H)-imidazo[1,2-a]pyridines (262) with the loss of a molecule of hydrogen83 (See Scheme 48). Compounds 262 reacted with several active methylene compounds such as diethyl malonate, ethyl acetoacetate, and ethyl cyanoacetate in the presence of a base to provide 3-vinylimidazo[1,2-a]pyridin-2-ols 263, and the products, in turn, on heating at reduced pressure were transformed to the corresponding pyrano[2’,3’-b]imidazo[1,2-a]pyridin-2-ones 264.83 Furthermore, compounds 262 reacted with ethyl 4-bromoacetoacetate to give directly spiro[2-cyclopentene-1,3-imidazo[1,2-a]pyridine] derivatives 266 via the dehydrobromination of the initially formed 3-vinylimidazo[1,2-a]pyridin-2-ols 265. The smooth replacement of the 2-methylthio group of 266 by primary and secondary amines was also reported. The proton signals of compounds 260 and their 2-amino derivatives 267 showed an interesting concentration-dependency in their 1H-NMR spectra in CDCl3.84
4.2. REACTIONS OF 2- OR 4-METHYL OR AMINO GROUP ON PYRIDIUM RING
The increased acidity of the methyl and amino group at the 2- and 4-positions of the pyridinium salts is well realized by seeking their resonance structures (see Figure 1) and, in particular, various reactions of the activated 2-methyl, 2-methylene, and 2-amino groups have been investigated. For example, Tschitschibabin reaction is a representative method for obtaining 3-unsubstituted indolizines 27385 (See Scheme 49). This reaction affords compounds 273 via the intramolecular nucleophilic addition of the methanide ion 270 (X=CR3), generated from the alkaline treatment of 1-acylmethyl-2-methyl- or 2-(substituted methyl)pyridinium halides 268, to the ketone carbonyl group, the proton shift of the resulting 271 (X=CR3), and the aromatization with the loss of water from 272 (X=CR3). Indolizines 273 obtained thus may be useful precursors for cycl[3.2.2]azines 27586 and 3-vinylindolizines 276.87 Similarly, the use of 2-aminopyridinium salts 269 in this reaction gave the corresponding 3-unsubstituted imidazo[1,2-a]pyridines 274.88
The alkaline treatment of 1-ethoxycarbonylmethyl-2-(substituted methyl)pyridinium salts 277 and 2-amino-1-(ethoxycarbonylmethyl)pyridinium halides 278 afforded the corresponding 2-indolizinols 28089 and imidazo[1,2-a]pyridin-2-ols 28290 with the elimination of a hydrogen halide and an alcohol. The keto forms such as 281 and 283 in these reactions were not confirmed (Scheme 50).
When the alkaline treatment of 1-(ethoxycarbonylmethyl)pyridinium salts (284) or 1-[2,2-bis(alkylthio)-1-ethoxycarbonylvinyl]pyridinium salts (289) having an alkyl or benzyl group at the 2-position was examined, we found the formation of the corresponding 2(3H)-indolizinones (285) or 3-[bis(alkylthio)methylene]-2(3H)-indolizinones (290) but 2-indolizinols 286 which were enol forms of 285 could not be detected at all.91 The reactions of 2(3H)-indolizinones 285 with various alkyl halide in the presence of a base afforded the expected 3,3-dialkyl-2(3H)-indolizinones (287) but those with dialkyl sulfates reacted in the enol forms 286 to yield 2-alkoxyindolizines (288).91c On the other hand, bifunctionalized 3-methylene-2(3H)-indolizinones 290 reacted with some acetates activated with an electron-withdrawing or aryl group in the presence of a base to provide the corresponding pyrano[2,3-b]indolizin-2-one derivatives 291.91a,b These results are summarized in Scheme 51.
We further examined this reaction to find a novel synthetic method for functionalized 3-vinylindolizines 293 by employing a combination of the vinylation of 2(3H)-indolizinone intermediates 285 and alkylation or acylation of the resulting 3-vinyl-2-indolizinols 292.91d These functionalized 3-vinylindolizines 293 have been proven to be important precursors or intermediates for forming 3-acylpyrano[2,3-b]indolizin-2-ones 294,92 2-(arylcarbonyl)furo[2,3-b]indolizines 297,93 and 4-acyl-2-arylcarbonyl-2,3-dihydrooxepino[2,3-b]indolizin-3-ols 298 (Scheme 52).94 Compounds 297 and 298 were formed via the 5-exo-trig cyclization (Michael addition) of the major carbanions 295 generated by the deprotonation of 2-arylcarbonylmethoxy-3-vinylindolizines 293 onto the 2-vinyl group and then aromatization of the resulting 296 with the elimination of an active methylene compound, and the 7-exo-trig cyclization (nucleophilic addition) of the minor carbanions 295’ to the ketone carbonyl group on the 3-vinyl group, respectively. Interestingly, the acid-catalyzed dehydration of 4-acetyl-2-arylcarbonyl-3-methyl-2,3-dihydrooxepino[2,3-b]indolizin-3-ols 298 (R5=Ac, R7=Me) did not afford the initially expected full-conjugated oxepino[2,3-b]indolizines, but, instead of them, only 3-methylene derivatives 299 were obtained. On the other hand, similar treatment of 4-ethoxycarbonyl-3-phenyl-2,3-dihydrooxepino[2,3-b]indolizin-3-ols 298 (R5=CO2Et, R7=Ph) gave the corresponding full-conjugated products 300. These full-conjugated oxepino[2,3-b]indolizines 300 were considerably unstable and, on heating in ethanol, underwent the smooth rearrangement to 2-arylcarbonyl-2-phenylpyrido[1,2-a]indol-1-ones 301. From these facts we could realize a trend to evidently avoid the formation of compounds with an unfavorable 4nπ-electron system, though the oxepine ring of the full-conjugated oxepino[2,3-b]indolizines 300 was not planar.
Some 3-(mercapto)methylene-2(3H)-indolizinones 302, readily available from the reactions of pyridinium salts 284 and carbon disulfide and alkylating agents or dithioesters in the presence of a base, are also useful materials for obtaining new nitrogen-bridged heterocycles such as 1,3-oxazino[6,5-b]indolizines 305,95 4(1H)-8,8a-dihydro-1,4-thiazino[3,4,5-cd]indolizinones 30896 and cycl[3.2.2]azin-3-ols 311,97 and 3-(1,3-oxathiol-2-ylidene)-2(3H)-indolizinones 31398 (See Scheme 53). An equilibrium is present between thiol compounds 303 and enol ones 302, and they reacted at the sulfur atom of the thiol group with soft alkylating agents and at the oxygen atom of the hydroxyl group with hard electrophiles. For example, when compounds 302 were allowed to react with some isothiocyanates in the presence of a base, 2H-3,4-dihydro-1,3-oxazino[6,5-b]indolizine-2,4-diones (305) were formed via the intramolecular addition of the imide atom onto the carbon atom of the thiocarbonyl group of the initially generated adducts 304 with a loss of methanethiolate ion. On the other hand, the reactions of 303 with α-bromoacetonitrile, ethyl bromoacetate, and phenacyl bromides in the presence of a base proceeded via the S-alkylation followed by the deprotonation of the resulting 306 to provide products 308 or 313 depending upon the nature of the substituents R5 and R7. Furthermore, the dehydrogenation of compounds 308 with 2,3-dichloro-5,6-dicyano-p-benzoquinone (DDQ) under the heating conditions caused to the ring contraction-desulfurization to yield cycl[3.2.2]azin-3-ols 311 by way of the dehydrogenated derivatives 309 or 310.
In 1968 Severin and Böhme reported the first synthesis of 2-allylidene-1,2-dihydropyridines 317 and 4-allylidene-1,4-dihydropyridines 320 from the reactions of some 1,2- 314 (R1=Me) and 1,4-dimethylpyridinium salts 318 (R1=Me) with 1-dimethylamino-2-nitro-2-phenylethylene in the presence of a base99 (See Scheme 54). We100 and other researchers101 described the preparation of various allylidenedihydropyridine analogs 317 and 320 by using more common vinylating agents such as ethoxymethylene and ketene dithioacetal compounds. Similar compounds 323 were also synthesized by the reactions of 2-[2,2-bis(methylthio)vinyl]-1-methylpyridinium salts (321 (R1=Me)) with malondinitrile in the presence of a base.102 These products 317 and 320 were formed via the Michael addition of zwitterions 315’ and 319’, which are the resonance contributors of 2-methylene-1,2- 315 and 4-methylene-1,4-dihydropyridines 319 and generated by the dehydrohalogenation of salts 314 and 318, onto a vinylating agent and the elimination of a HZ molecule. On the other hand, the formation of 323 proceeded by way of the nucleophilic addition of dicyanomethanide ion generated from the deprotonation of malondinitrile onto the positive carbon atom of ketene dithioacetal 321, followed by the loss of a methanethiol from adduct 322.
As shown in Figure 5, a remarkable structural feature of 2-allylidene-1,2-dihydropyridines 317 is the presence of the nucleophilic centers at the 2(1)- and 2(3)-position. Actually, the structural data of 2-(3-cyano-3-ethoxycarbonylallylidene)-1-methyl-1,2-dihydropyridine supported a pyridinium ring form rather than a 1,2-dihydropyridine one and the high double bonded character of the C(2(1))─C(2(2)) and C(2(2))─C(2(3)) bonds, and hence exhibited a larger contribution of the allyl anion structures 317b and 317c than the allylidene one 317a. It can be readily realized that 2-allylidene-1,2-dihydropyridines bearing an electron-poor reaction site in the R1 substituent should be useful precursors for some heterocycles.
In 1977 we first reported the synthesis of functionalized 3-vinylpyrazolo[1,5-a]pyridine derivatives 327 from the thermolysis of 2-[3-cyano-3-(ethoxycarbonyl)allylidene]-1-(disubstituted methyleneamino)-1,2- dihydropyridines (325), which could be readily synthesized via the N-alkylation of pyridinium 1-[(N-ethoxycarbonyl)imidoyl]aminides 15 with iodomethane and subsequent vinylation of the resulting pyridinium salts with ethoxymethylene compounds, in xylene at the reflux temperature103 (See Scheme 55). Similarly, we could obtain some functionalized 1-acyloxy-1-vinylindolizines 331 by the reactions of 2-allylidene-1-ethoxycarbonylmethyl-1,2-dihydropyridines 328 and anhydride.104 Products 327 and intermediates 330 were formed by the electrocyclic cyclization (π6s) of 325 and 329 and subsequent aromatization with the elimination of ethyl N-methylcarbamate and ethanol respectively. Compounds 331 were formed by the acetylation of 2-indolizinol 330 with acetic anhydride.
We could realize the high possibility of the transformation from a divinylamine or its aza-analogue system 332 to a pyrrole or pyrazole skeleton 334 through the mechanistic consideration of these reactions (Figure 6).
So we synthesized 2-methylpyridinium salts 335 and 336 having a vinyl or methyleneamino group at the 1-position from the alkylation of 2-methylpyridinium (2-thioxo)ethanides 6 or (thiocarbonyl)aminides 16, and examined their reactions with ethoxymethylene compounds. As expected, we could obtain various 1-vinylindolizines 339 or 3-vinylpyrazolo[1,5-a]pyridines 340 bearing an alkylthio105 and alkylamino group106 at the 2-position (See Scheme 56). The 2-alkylamino compounds 339 and 340 could be converted to 1-alkyl-1,2-dihydropyrido[3,2-a]indolizin-2-ones 341 and their 10-aza-analogs 342 in acetic acid under the reflux conditions or to the corresponding 2-imine derivatives 343 and 344 by the treatment of potassium t-butoxide.106 The treatment of 2-(methylthio)indolizine derivatives 339 (X=CCO2Et) with conc. hydrochloric acid afforded 2H-thiino[3,2-a]indolizin-2-imine hydrochlorides 345, which were then converted to carbonyl derivatives 346 on heating in acetic acid.107 On the other hand, the reactions of 345 and phenacyl bromides in the presence of a strong base provided thieno[3,2-a]indolizines 351 via the reaction sequence shown below.108
We also found a one-pot synthesis of 2H-pyrano[3,2-a]indolizin-2-ones 355 which was performed by refluxing 2-[3-cyano-3-(ethoxycarbonyl)allylidene]-1-ethoxycarbonylmethyl-1,2-dihydropyridines (352) in acetic acid (Scheme 57).
Another reaction is a 1,6-hydrogen shift from the N-methylene group of 2-allylidene-1,2-dihydropyridine derivatives, and it generates in situ reactive 1,5-dipoles and the 1,5-dipolar cyclization gives indolizines. In 1977 Kobayashi et al. described a one-pot synthesis of the corresponding 2-[3-cyano-2-(methylthio)allylidene]-1-ethoxycarbonylmethyl-1,2-dihydropyridines 357 and ethyl 2-(disubstituted methyl)indolizine-3-carboxylates 358 in the reactions of 2-methylpyridinium salts 356 and 3,3-bis(methylthio)propenenitriles in the presence of a base in DMF at 100 oC101b (See Scheme 58). They reported that indolizines 358 were formed by way of 2-allylidene-1,2-dihydropyridines 357, though the mechanisms were not described until 1981.109 On the other hand, we found the formation of ethyl indolizine-3-carboxylate 361 in the thermolysis of allylidene-1,2-dihydropyridine 359 in 1978, and showed the possible reaction routes via the 1,5-dipolar cyclization of the intermediates 360 and 360’ generated from the hydrogen-shift of 359 and subsequent aromatization to final product 361.104
Some condensation reactions of 2-alkyl- or 2-aminopyridinium salts with various bifunctional reagents such as α-diketones and α-ketoesters have been well documented. For example, quinolizinium salts 367 were prepared by the stepwise dehydrations between 1-acylmethyl-2-alkylpyridinium salts 362 and 1,2-dicarbonyl compounds in the presence of a base110 (See Scheme 59). Similar treatment of 2-alkyl-1-amino- 363 and 1,2-diaminopyridinium salts 364 gave the corresponding pyrido[1,2-b]pyridazinium salts 368111 and pyrido[1,2-b]-as-triazinium salts 369 respectively.112
5. CONCLUSION
In this review I briefly described some of more common and practical reactions of pyridinium ylides and
their closely related pyridinium salts. The chemistry is now changing from the traditional syntheses of bicyclic heterocycles such as indolizine and pyrazolo[1,5-a]pyridine to those of more complex and functionalized compounds. Although many novel methods for preparing such compounds have been developed, it is important to notice that no method is almighty and the scope of the application is severely restricted. To expand further this chemistry of pyridinium ylides and related pyridinium salts I sincerely hope the development of novel methodologies in this field continue.
References
1. F. Kröhnke, Chem. Ber, 1935, 68, 1177. CrossRef
2. a) H.-J. Timpe, Z. Chem., 1972, 7, 250; b) C. G. Stuckwisch, Synthesis, 1973, 469; CrossRef c) V. P. Litvinov, Rus. J. Org. Chem, 1993, 29, 1722; d) V. P. Litvinov, Rus. J. Org. Chem., 1994, 30, 1658; e) V. P. Litvinov, Zh. Org. Khim., 1995, 31, 1441; f) W. Sliwa, Heterocycles, 1996, 43, 2005; CrossRef g) A. Kakehi, Yuki Gosei Kagaku Kyokaishi, 2005, 63, 36; CrossRef h) J. Jacobs, E. V. Hende, S. Claessens, and N. D. Kimpe, Cur. Org. Chem., 2011, 15, 1340.. CrossRef
3. R. Gösl and A. Meuwen, Chem Ber., 1959, 92, 2521. CrossRef
4. a) L. A. Carpino, J. Chem. Soc. (C), 1960, 3133; b) Y. Tamura, J. Minamikawa, Y. Miki, S. Matsugashita, and M. Ikeda, Tetrahedron Lett., 1972, 13, 4133. CrossRef
5. T. Okamoto, M. Hirobe, C. Mizushima, and A. Osawa, J. Pharm. Soc. Jpn., 1963, 83, 308.
6. a) Y. Tamura, N. Tsujimoto, and M. Ikeda, Chem. Commun., 1971, 310; CrossRef b) T. Sasaki, K. Kanematsu, and A. Kakehi, J. Org. Chem., 1972, 37, 3106; CrossRef c) Y. Tamura, Y. Sumida, and M. Ikeda, J. Chem. Soc., Perkin Trans. 1, 1973, 2091; CrossRef d) Y. Tamura, Y. Miki, Y. Sumida, and M. Ikeda, J. Chem. Soc., Perkin Trans. 1, 1973, 2580; CrossRef e) Y. Tamura, Y. Sumida, and M. Ikeda, J. Chem. Soc., Perkin Trans. 1, 1975, 575; CrossRef f) Y. Tominaga, S. Hidaki, Y. Matsuda, and G. Kobayashi, J. Pharm. Soc. Jpn., 1984, 104, 440.
7. a) A. Kakehi, S. Ito, K. Uchiyama, and Y. Konno, Chem. Lett., 1976, 17, 413; CrossRef b) A. Kakehi, S. Ito, K. Uchiyama, Y. Konno, and K. Kondo, J. Org. Chem., 1977, 42, 443. CrossRef
8. a) F. Kröhnke and K. Gerlach, Chem. Ber., 1962, 95, 1108; CrossRef b) F. Kröhnke and W. Zecher, Chem. Ber., 1962, 95, 1128; CrossRef c) S. Suzue, M. Hirobe, and T. Okamoto, Chem. Pharm. Bull., 1973, 21, 2146; CrossRef d) R. Krischke, R. Grashey, R. Huisgen, Ann., 1977, 498; e) Y. Tominaga, Y. Miyake, H. Fujito, Y. Matsuda, and G. Kobayashi, J. Pharm. Soc. Jpn., 1977, 97, 927; f) E. Fischer, G. Remberz, and K. M. Wollin, Z. Chem., 1978, 18, 137.
9. a) Y. Tominaga, H. Fujito, H. Norisue, A. Ushirogochi, Y. Matsuda, and G. Kobayashi, J. Pharm. Soc. Jpn., 1979, 99, 1081; b) A. Kakehi, S. Ito, Y. Ohno, S. Schiba, and S. Kamata, Bull. Chem. Soc. Jpn., 1987, 60, 3713; CrossRef c) A. Kakehi, S. Ito, H. Suga, T. Miwa, T. Mori, T. Fujii, N. Tanaka, and T. Kobayashi, Chem. Pharm. Bull., 2003, 51, 75. CrossRef
10. a) A. Kakehi, S. Ito, Y. Konno, and T. Maeda, Bull. Chem. Soc. Jpn., 1978, 51, 251; CrossRef b) A. Kakehi, S. Ito, and Y. Hashimoto, Bull. Chem. Soc. Jpn., 1996, 70, 1769. CrossRef
11. W. J. Linn, O. W. Webster, and R. E. Benson, J. Am. Chem. Soc., 1965, 87, 3651. CrossRef
12. K. Hafner, D. Zinser, and K. L. Moritz, Tetrahedron Lett., 1964, 1733. CrossRef
13. K. Watanabe, H. Suga, and A. Kakehi, 39th Congress of Heterocyclic Chemistry, Kashima, Japan, 2009, 2P-45, 153.
14. a) A. Kakehi and S. Ito, Bull. Chem. Soc. Jpn., 1974, 47, 938; CrossRef b) O. Tsuge, S. Kanemasa, and S. Takenaka, Bull. Chem. Soc. Jpn., 1985, 58, 3137; CrossRef c) O. Tsuge, S. Kanemasa, and S. Takenaka, Bull. Chem. Soc. Jpn., 1985, 58, 3320; CrossRef d) Y. Tominaga, Y. Ichihara, and A. Hosomi, Heterocycles, 1988, 27, 1345; CrossRef e) Y. Tominaga, Y. Ichihara, and A. Hosomi, J. Heterocycl. Chem., 1988, 25, 1745; CrossRef f) Y. Tominaga, Y. Ichihara, T. Mori, C. Kamio, and A. Hosomi, J. Heterocycl. Chem., 1990, 27, 263; CrossRef g) X. Wei, Y. Hu, T. Li, and H. Hu, J. Chem. Soc., Perkin Trans. 1, 1993, 2487. CrossRef
15. a) V. Boekelheide and K. Fahrenholtz, J. Am. Chem. Soc., 1961, 83, 458; CrossRef b) K. Matsumoto, T. Uchida, T. Sugi, and Y. Yagi, Chem. Lett., 1982, 869; CrossRef c) J. Alvarez-Builla, M. G. Quintanilla, C. Abril, and M. T. Gandasegui, J. Chem Res. (S), 1984, 202; d) Y. Tominaga, Y. Shiroshita, Y. Matsuda, and A. Hosomi, Heterocycles, 1987, 26, 2073. CrossRef
16. a) J. E. Douglass and J. M. Wesolosky, J. Org. Chem., 1971, 36, 1165; CrossRef b) C. Leonte and I. Zugrãvescu, Tetrahedron Lett., 1972, 2029; CrossRef c) T. Kutsuma, K. Fujiyama, Y. Sekine, and Y. Kobayashi, Chem. Pharm. Bull., 1972, 20, 1558; CrossRef d) T. Kutsuma, K. Fujiyama, and Y. Kobayashi, Chem. Pharm. Bull., 1972, 20, 1809. CrossRef
17. a) K. Matsumoto, T. Uchida, Y. Ikemi, T. Tanaka, M. Asahi, T. Kato, and H. Konishi, Bull. Chem. Soc. Jpn., 1987, 60, 3645; CrossRef b) A. Diaz-Ortiz, E. Diez-Barra, A. de la Hoz, A. Loupy, A. Petit, and L. Sanchez, Heterocycles, 1994, 38, 785; CrossRef c) K. Matsumoto, R. Ohta, T. Uchida, H. Nishioka, M. Yashida, and A. Kakehi, J. Heterocycl. Chem., 1995, 32, 367. CrossRef
18. a) R. Huisgen, R. Grashey, and R. Krischke, Tetrahedron Lett., 1962, 3, 387; CrossRef b) V. Boekelheide and N. A. Fedoruk, J. Org. Chem., 1968, 33, 2062; CrossRef c) T. Sasaki, K. Kanematsu, and Y. Yukimoto, J. Chem. Soc.(C), 1970, 481; CrossRef d) Y. Tamura, Y. Sumida, Y. Miki, and M. Ikeda, J. Chem. Soc., Perkin Trans. 1, 1975, 406. CrossRef
19. a) T. Okamoto, M. Hirobe, Y. Tamai, and E. Yabe, Chem. Pharm. Bull., 1966, 14, 506; CrossRef b) T. Okamoto, M. Hirobe, and E. Yabe, Chem. Pharm. Bull., 1966, 14, 523. CrossRef
20. a) T. Sasaki, K. Kanematsu, and A. Kakehi, J. Org. Chem., 1971, 36, 2978; CrossRef b) T. Sasaki, K. Kanematsu, A. Kakehi, and G. Ito, Bull. Chem. Soc. Jpn., 1972, 45, 2050. CrossRef
21. T. Sasaki, K. Kanematsu, and A. Kakehi, Tetrahedron Lett., 1972, 13, 5245. CrossRef
22. Our unpublished data.
23. J. Streith and J.-M. Cassal, C. R. Acad. Sci. (C), 1967, 264, 1307.
24. a) J. Streith and J.-M. Cassal, Angew. Chem., 1968, 80, 117; CrossRef b) J. Streith, A. Blind, J.-M. Cassal, and Sigwalt, Bull. Soc. Chim. Fr., 1969, 948.
25. a) T. Sasaki, K. Kanematsu, and A. Kakehi, J. Chem. Soc. (D), 1969, 432; CrossRef b) T. Sasaki, K. Kanematsu, A. Kakehi, I. Ichikawa, and K. Hayakawa, J. Org. Chem., 1970, 35, 426. CrossRef
26. A. Balasubramanian, J. M. McIntosh, and V. Snieckus, J. Org. Chem., 1970, 35, 433. CrossRef
27. J. P. Luttringer and J. Streith, Tetrahedron Lett., 1973, 14, 4163. CrossRef
28. J. Streith, G. Wolff, and H. Fritz, Tetrahedron, 1977, 33, 1349. CrossRef
29. J. R. Frost and J. Streith, J. Chem. Soc., Perkin Trans. 1, 1978, 1297. CrossRef
30. T. Tsuchiya, J. Kurita, and V. Snieckus, J. Org. Chem., 1977, 42, 1856. CrossRef
31. E. C. Taylor and I. G. Turchi, Chem. Rev., 1979, 79, 181. CrossRef
32. I. Mitteil, W. Augstein, and F. Kröhnke, Liebigs Ann. Chem., 1966, 697, 158. CrossRef
33. a) Y. Tamura, N. Tsujimoto, Y. Sumida, and M. Ikeda, Tetrahedron, 1972, 28, 21; CrossRef b) E. Pohjara, Tetrahedron Lett., 1972, 13, 2585; CrossRef c) T. Sasaki, K. Kanematsu, A. Kakehi, and G. Ito, Tetrahedron, 1972, 28, 4947; CrossRef d) T. Sasaki, K. Kanematsu, A. Kakehi, and G. Ito, J. Chem. Soc. Perkin Trans. 1, 1973, 2089. CrossRef
34. Y. Tamura, S. Sumida, and M. Ikeda, Chem. Pharm. Bull., 1972, 20, 1058. CrossRef
35. D. Mörler and F. Kröhnke, Liebigs Ann. Chem. 1971, 744, 65. CrossRef
36. a) C. Maseda, M. Sone, Y. Tominaga, R. Natsuki, Y. Matsuda, and G. Kobayashi, J. Pharm. Soc. Jpn., 1974, 94, 839; b) K. Kurata, H. Awaya, Y. Tominaga, Y. Matsuda, and G. Kobayashi, J. Pharm. Soc. Jpn., 1978, 98, 631.
37. a) H. Sashida and T. Tsuchiya, Chem. Pharm. Bull., 1984, 32, 4600; CrossRef b) T. Tsuchiya, M. Kato, and H. Sashida, Chem. Pharm. Bull., 1984, 32, 4666; CrossRef c) H. Sashida, M. Kato, and T. Tsuchiya, Chem. Pharm. Bull., 1988, 36, 3826. CrossRef
38. a) K. Marx and W. Eberbach, Tetrahedron, 1997, 53, 14687; CrossRef b) K. Marx and W. Eberbach, Eur. J. Org. Chem., 2000, 6, 2063.
39. a) A. Kakehi, S. Ito, K. Nagata, M. Kinoshita, and N. Kakinuma, Chem. Pharm. Bull., 1987, 35, 156; CrossRef b) A. Kakehi, S. Ito, N. Kinoshita, and Y. Abaka, Bull. Chem. Soc. Jpn., 1988, 61, 2055. CrossRef
40. a) W. Zecher and F. Kröhnke, Chem. Ber., 1961, 94, 690; CrossRef b) W. Zecher and F. Kröhnke, Chem. Ber., 1961, 94, 698; CrossRef c) W. Zecher and F. Kröhnke, Chem. Ber., 1961, 94, 707. CrossRef
41. Y. Sugimura, N. Soma, and Y. Kishida, Bull. Chem. Soc. Jpn., 1972, 45, 3174. CrossRef
42. a) M. F. Aldersley, F. M. Dean, and R. Nayyir-Mashir, J. Chem. Soc., Perkin Trans. 1, 1983, 1753; CrossRef b) M. F. Aldersley, F. M. Dean, and A. S. Hamzah, Tetrahedron Lett., 1986, 27, 255. CrossRef
43. C.–P. Chuang and A. Tsai, Synthesis, 2006, 675. CrossRef
44. T. Sasaki, K. Kanematsu, and A. Kakehi, Tetrahedron, 1972, 28, 1469. CrossRef
45. a) B. M. Trost and M. J. Bogdanowicz, J. Am. Chem. Soc., 1973, 95, 5307; CrossRef b) C. S. F. Tang and H. Rapoport, J. Org. Chem., 1973, 38, 2806; CrossRef c) J. P. Marino and T. Kaneko, Tetrahedron Lett., 1973, 14, 3975. CrossRef
46. a) Y. Yamashita, Y. Miyauchi, and M. Masumura, Chem. Lett., 1983, 12, 489; CrossRef b) A. M. Sheslopalov, Yu A.Sharanin, V. P. Litvinov, and O. M. Nefedov, Zh. Org. Khim., 1989, 25, 1111; c) S. Kojima, K. Fujitomo, Y. Shinohara, M. Shimizu, and K. Ohkata, Tetrahedron Lett., 2000, 41, 9847; CrossRef d) N. Kanomata, R. Sakaguchi, K. Sekine, S. Yamashita, and H. Tanaka, Adv. Synth. Cataly., 2010, 352, 2966. CrossRef
47. A. Kakehi and S. Ito, J. Org. Chem., 1974, 39, 1542. CrossRef
48. a) A. Kakehi, S. Ito, and T. Manabe, J. Org. Chem., 1975, 40, 544; CrossRef b) A. Kakehi, S. Ito, T. Manabe, H. Amano, and K. Shimaoka, J. Org. Chem., 1976, 41, 2739; CrossRef c) A. Kakehi, S. Ito, T. Manabe, T. Maeda, and K. Imai, J. Org. Chem., 1977, 42, 2514. CrossRef
49. T. Eicher and A. Hansen, Tetrahedron Lett., 1967, 8, 1169. CrossRef
50. T. Sasaki, K. Kanematsu, and A. Kakehi, J. Org. Chem., 1970, 35, 2451. CrossRef
51. a) J. W. Lown and K. Matsumoto, Can. J. Chem., 1972, 50, 584; CrossRef b) T. Eicher, von E. Angerer, and A. M. Hansen, Ann. Chem., 1971, 746, 102. CrossRef
52. a) A. Kakehi, S. Ito, and J. Hakui, Chem. Lett., 1992, 21, 777; CrossRef b) A. Kakehi, S. Ito, and J. Hakui, Bull. Chem. Soc. Jpn, 1993, 66, 3475. CrossRef
53. a) A. Kakehi and S. Ito, Heterocycles, 1993, 36, 1195; CrossRef b) A. Kakehi, S. Ito, M. Mitani, and M. Kanaoka, Bull. Chem. Soc. Jpn., 1994, 67, 1646. CrossRef
54. A. Kakehi, S. Ito, and S. Fujita, Bull. Chem. Soc. Jpn., 1995, 68, 1473. CrossRef
55. A. Kakehi, K. Itoh, H. Suga, H. Kobayashi, H. Muranaka, and M. Shiro, Heterocycles, 2011, 83, 1013. CrossRef
56. A. Kakehi, H. Suga, Y. Okumura, and T. Nishi, Heterocycles, 2010, 81, 175. CrossRef
57. A. Kakehi, S. Ito, and S. Nishizawa, Chem. Pharm. Bull., 1998, 46, 934. CrossRef
58. A. Kakehi, K. Itoh, H. Suga, Y. Ohkubo, A. Kobayashi, and T. Nishi, Heterocycles, 2012, 85, 305. CrossRef
59. a) A. Kakehi, S. Ito, F. Ishida, and Y. Tominaga, Heterocycles, 1995, 42, 2657; CrossRef b) A. Kakehi, S. Ito, F. Ishida, and Y. Tominaga, J. Org. Chem., 1997, 62, 7788. CrossRef
60. T. Kato and S. Masuda, Chem. Pharm. Bull., 1975, 23, 452. CrossRef
61. A. Kakehi, S. Ito, T. Funahashi, and Y. Ota, J. Org. Chem., 1976, 41, 1570. CrossRef
62. a) Y. Matsuda, H. Gotou, K. Katou, H. Matsumoto, M. Yamashita, K. Takahashi, and S. Ide, Heterocycles, 1990, 31, 977; CrossRef b) Y. Matsuda, H. Gotou, K. Katou, H. Matsumoto, M. Yamashita, K. Takahashi, S. Ide, K. Furuno, and K. Torisu, Heterocycles, 1991, 32, 2217; CrossRef c) Y. Matsuda, M. Yamashita, K. Takahashi, S. Ide, K. Torisu, and K. Furuno, Heterocycles, 1992, 33, 295; CrossRef d) Y. Matsuda, M. Yamashita, K. Takahashi, S. Ide, T. Itou, C. Motokawa, and Y. Chiyomaru, Chem. Pharm. Bull., 1994, 42, 454. CrossRef
63. T. Okamoto, M. Hirobe, C. Mizushima, and A. Ohsawa, Chem. Pharm. Bull., 1963, 22, 780. CrossRef
64. a) T. Okamoto and H. Tani, Chem. Pharm. Bull., 1959, 7, 130; CrossRef b) T. Okamoto and H. Tani, Chem. Pharm. Bull., 1959, 7, 925; CrossRef c) T. Okamoto and H. Tani, Chem. Pharm. Bull., 1959, 7, 930; CrossRef d) W. E. Feely and E. H. Beavers, J. Am. Chem. Soc., 1959, 81, 4008. CrossRef
65. H. Aklbrecht and F. Kröhnke, Ann. Chem., 1967, 701, 126. CrossRef
66. Y. Tominaga, H. Fujito, K. Mizuyama, Y. Matsuda, and G. Kobayashi, Chem. Pharm. Bull., 1977, 25, 1519. CrossRef
67. a) A. Kakehi, S. Ito, M. Ito, and T. Yotsuya, Heterocycles, 1984, 22, 2237; CrossRef b) A. Kakehi, S. Ito, M. Ito, S. Yonezu, K. Maruta, and K. Yuito, Heterocycles, 1985, 23, 33; CrossRef c) A. Kakehi, S. Ito, M. Ito, T. Yotsuya, and K. Nagata, Bull. Chem. Soc. Jpn., 1985, 58, 1432; CrossRef d) A. Kakehi, S. Ito, K. Nagata, N. Kinoshita, and N. Kakinuma, Chem. Pharm. Bull., 1987, 35, 156; CrossRef e) A. Kakehi, S. Ito, S. Yonezu, K. Maruta, K. Yuito, M. Shiohara, and K. Adachi, Bull. Chem. Soc. Jpn., 1987, 60, 1867; CrossRef f) A. Kakehi, S. Ito, N. Kinoshita, and Y. Abaka, Bull. Chem. Soc. Jpn., 1988, 61, 2055. CrossRef
68. R. Schmidt, Angew. Chem., Int. Ed. Engl., 1975, 14, 581. CrossRef
69. A. Kakehi, H. Suga, Y. Goto, and N. Yamaguchi, Heterocycles, 2007, 74, 521. CrossRef
70. a) A. Kakehi, S. Ito, Y. Ohno, S. Shiba, and S. Kamata, Bull. Chem. Soc. Jpn., 1987, 60, 3713; CrossRef b) A. Kakehi, S. Ito, J. Hakui, and H. Fukazawa, Bull. Chem. Soc. Jpn., 1992, 65, 1244. CrossRef
71. a) A. Kakehi, S. Ito, S. Matsumoto, and Y. Morimoto, Chem. Lett., 1987, 2043; CrossRef b) A. Kakehi, S. Ito, T. Fujii, Y. Morimoto, S. Matsumoto, and M. Shiohara, Bull. Chem. Soc. Jpn., 1989, 62, 119. CrossRef
72. A. Kakehi, S. Ito, S. Hayashi, and T. Fujii, Bull. Chem. Soc. Jpn., 1995, 69, 3573. CrossRef
73. a) A. Kakehi, S. Ito, N. Yamada, and K. Yamaguchi, Bull. Chem. Soc. Jpn., 1990, 63, 829; CrossRef b) A. Kakehi, S. Ito, H. Isawa, and T. Takashima, Chem. Pharm. Bull., 1990, 38, 2662. CrossRef
74. A. Kakehi, S. Ito, T. Sakurai, K. Urushido, H. Isawa, and M. Enomoto, Bull. Chem. Soc. Jpn., 1991, 64, 3289. CrossRef
75. a) A. Kakehi, S. Ito, H. Suga, H. Takahashi, and K. Dobashi, Heterocycles, 2000, 52, 215; CrossRef b) H. Isawa, A. Kakehi, and H. Suga, Heterocyclic Commun., 2008, 14, 141. CrossRef
76. H. Isawa, A. Kakehi, and H. Suga, Heterocycles, 2009, 78, 319. CrossRef
77. a) A. Kakehi, S. Ito, N. Yamada, and K. Yamaguchi, Chem. Pharm. Bull., 1990, 38, 1527; CrossRef b) A. Kakehi, S. Ito, T. Sakurai, K. Urushido, H. Isawa, and T. Takashima, Chem. Pharm. Bull., 1990, 38, 2667; CrossRef c) A. Kakehi, S. Ito, T. Fujii, T. Ueda, and T. Hirata, Chem. Pharm. Bull., 1992, 40, 2313. CrossRef
78. a) A. Kakehi, S. Ito, and H. Sa, Chem. Pharm. Bull., 1999, 47, 1607; CrossRef b) A. Kakehi, S. Ito, K. Hirata, and P. Zuo, Chem. Pharm. Bull., 2000, 48, 865. CrossRef
79. A. Kakehi, S. Ito, H. Suga, and K. Yasuraoka, Heterocycles, 2001, 54, 185. CrossRef
80. a) A. Kakehi, S. Ito, H. Suga, T. Miwa, T. Mori, and T. Kobayashi, Heterocycles, 2002, 57, 17; CrossRef b) A. Kakehi, S. Ito, H. Suga, T. Miwa, T. Mori, T. Fujii, N. Tanaka, and T. Kobayashi, Chem. Pharm. Bull., 2003, 51, 75. CrossRef
81. a) A. Kakehi, H. Suga, T. Kako, T. Fujii, N. Tanaka, and T. Kobayashi, Chem. Pharm. Bull., 2003, 51, 1246; CrossRef b) A. Kakehi, H. Suga, Y. Kaneko, T. Fujii, and N. Tanaka, Chem. Pharm. Bull., 2005, 53, 1430; CrossRef c) A. Kakehi, H. Suga, and H. Isogai, Chem. Pharm. Bull., 2007, 55, 95; CrossRef d) A. Kakehi, H. Suga, Y. Okumura, M. Shinohara, T. Kako, T. Sekiguchi, and M. Shiro, Chem. Pharm. Bull., 2009, 57, 1385. CrossRef
82. a) A. Kakehi, H. Suga, A. Izumita, and T. Abe, Heterocycles, 2008, 76, 391; CrossRef b) A. Kakehi, H. Suga, Y. Okumura, K. Itoh, Y. Aikawa, K. Kobayashi, and K. Misawa, Chem. Pharm. Bull., 2010, 58, 1502. CrossRef
83. T. Abe, Y. Okumura, H. Suga, and A. Kakehi, Heterocycles, 2010, 80, 439. CrossRef
84. T. Abe, A. Kakehi, H. Suga, Y. Okumura, and K. Itoh, Heterocycles, 2010, 81, 2075. CrossRef
85. A. E. Tschitschibabin, Ber., 1927, 60, 1607.
86. A. Galbraith, T. Small, R. A. Bernes, and V. Boekelheide, J. Am. Chem. Soc., 1961, 84, 453. CrossRef
87. Y. Yamashita, D. Suzuki, and M. Masumura, Heterocycles, 1981, 16, 1499. CrossRef
88. A. E. Tschitschibabin, Ber., 1925, 58, 1704.
89. D. R. Bragg and D. G. Wibberly, J. Chem. Soc., 1963, 3277. CrossRef
90. A. E. Tschitschibabin, Ber., 1924, 57, 2092.
91. a) A. Kakehi, S. Ito, K. Nakanishi, and M. Kitagawa, Chem. Lett., 1979, 7, 297; CrossRef b) A. Kakehi, S. Ito, K. Nakanishi, K. Watanabe, and M. Kitagawa, Bull. Chem. Soc. Jpn., 1980, 53, 1115; CrossRef c) A. Kakehi, S. Ito, K. Watanabe, M. Kitagawa, S. Takeuchi, and T. Hashimoto, J. Org. Chem., 1980, 45, 5100; CrossRef d) A. Kakehi, S. Ito, B. Wada, K. Watanabe, K. Nishimura, and A. Kumagai, Bull. Chem. Soc. Jpn., 1982, 55, 3590. CrossRef
92. A. Kakehi, S. Ito, S. Murakami, and H. Sano, Bull. Chem. Soc. Jpn., 1984, 57, 548. CrossRef
93. a) A. Kakehi, S. Ito, T. Ohizumi, and M. Ito, Bull. Chem. Soc. Jpn., 1983, 56, 1219; CrossRef b) A. Kakehi, S. Ito, H. Furuta, and K. Todoroki, J. Fac. Eng. Shinshu. Univ., 1985, 58, 1.
94. a) A. Kakehi, S. Ito, and H. Muranaka, J. Fac. Eng. Shinshu. Univ., 1994, 75, 31; b) A. Kakehi, S. Ito, and H. Muranaka, Bull. Chem. Soc. Jpn., 1994, 67, 2795. CrossRef
95. A. Kakehi, S. Ito, T. Sakurai, K. Urushido, S. Hatanaka, and K. Sugiura, Bull. Chem. Soc. Jpn., 1991, 64, 3296. CrossRef
96. a) A. Kakehi, S. Ito, and S. Hatanaka, Chem. Lett., 1989, 18, 2229; CrossRef b) A. Kakehi, S. Ito, T. Fujii, T. Sakurai, K. Urushido, S. Hatanaka, T. Mabuchi, and S. Matsushita, Bull. Chem. Soc. Jpn., 1990, 63, 3571. CrossRef
97. H. Muranaka, A. Kakehi, H. Suga, and K. Itoh, Heterocycles, 2011, 82, 1251. CrossRef
98. A. Kakehi, S. Ito, H. Suga, T. Kobayashi, and S. Hatanaka, Chem. Pharm. Bull., 1998, 46, 1866. CrossRef
99. T. Severin, H.- J. Böhme, Chem. Ber., 1968, 101, 2925. CrossRef
100. a) A. Kakehi, S. Ito, T. Funahashi, and N. Ogasawara, Chem. Lett., 1975, 4, 919; CrossRef b) A. Kakehi, S. Ito, T. Funahashi, and N. Ogasawara, Bull. Chem. Soc. Jpn., 1976, 49, 2250. CrossRef
101. a) J. Kuthan, D. Ilavsky, J. Krochl, and P. Trĕka, Tetrahedron Lett., 1976, 4763; CrossRef b) Y. Tominaga, Y. Miyake, H. Fujito, K. Kurata, H. Awaya, Y. Matsuda, and G. Kobayashi, J. Pharm. Soc. Jpn., 1977, 25, 1528.
102. K. Mizuyama, Y. Tominaga, Y. Matsuda, and G. Kobayashi, J. Pharm. Soc. Jpn., 1975, 95, 290.
103. a) A. Kakehi, S. Ito, K. Uchiyama, and K. Kondo, Chem. Lett., 1977, 6, 545; CrossRef b) A. Kakehi, S. Ito, K. Uchiyama, and K. Kondo, J. Org. Chem., 1978, 43, 2896. CrossRef
104. a) A. Kakehi, S. Ito, T. Maeda, R. Takeda, M. Nishimura, and T. Yamaguchi, Chem. Lett., 1978, 7, 59; CrossRef b) A. Kakehi, S. Ito, T. Maeda, R. Takeda, M. Nishimura, M. Tamashima, and T. Yamaguchi, J. Org. Chem., 1978, 43, 4837. CrossRef
105. a) A. Kakehi, S. Ito, and K. Watanabe, Bull. Chem. Soc. Jpn., 1980, 53, 1775; CrossRef b) A. Kakehi, S. Ito, T. Ohizumi, and T. Maeda, J. Org. Chem., 1982, 47, 369. CrossRef
106. a) A. Kakehi, S. Ito, K. Watanabe, T. Ono, and T. Miyajima, Chem. Lett., 1979, 8, 205; CrossRef b) A. Kakehi, S. Ito, K. Watanabe, T. Ono, and T. Miyajima, J. Chem. Res., (S), 1980, 18.
107. A. Kakehi, S. Ito, H. Suga, S. Takano, and K. Hirata, Chem. Pharm. Bull., 1998, 46, 1632. CrossRef
108. A. Kakehi, S. Ito, T. Ueda, and S. Takano, Chem. Pharm. Bull., 1993, 41, 1753. CrossRef
109. K. Kurata, H. Awaya, Y. Tominaga, Y. Matsuda, and G. Kobayashi, J. Pharm. Soc. Jpn., 1981, 101, 980.
110. a) O. Westphal, K. Jahn, and W. Heffe, Arch. Pharm., 1961, 294, 37; CrossRef b) F. Kröhnke, H. Schnegelberg, and W. Weis, Chem. Ber., 1964, 97, 3566; CrossRef c) J. Ezquerra and J. Alvarez-Builla, J. Heterocycl. Chem., 1986, 23, 1151. CrossRef
111. M. P. Matia, J. L. G. Navío, J. J. Vaquero, and J. Alvarez-Builla, J. Heterocycl. Chem., 1990, 27, 661. CrossRef
112. a) N. Baranova, A. K. Sheinkman, and A. N. Kost, Khim. Geterotsikl. Soed., 1973, 1266; b) Z. Juhász-Riedl, G. Hajás, G. Kollenz, and A. Messmer, Chem. Ber., 1989, 122, 1935. CrossRef