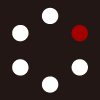
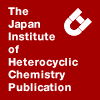
HETEROCYCLES
An International Journal for Reviews and Communications in Heterocyclic ChemistryWeb Edition ISSN: 1881-0942
Published online by The Japan Institute of Heterocyclic Chemistry
e-Journal
Full Text HTML
Received, 29th February, 2012, Accepted, 30th March, 2012, Published online, 2nd April, 2012.
DOI: 10.3987/REV-12-737
■ Synthesis of 2,2’-Bipyridines by Transition Metal-Catalyzed Alkyne/Nitrile [2 + 2 + 2] Cycloaddition Reactions
Sentaro Okamoto*
Department of Material and Life Chemistry, Faculty of Engineering, Kanagawa University, 3-27-1 Rokkakubashi, Kanagawa-ku, Yokohama 221-8686, Japan
Abstract
2,2’-Bipyridines are of great importance in the fields of catalysis, coordination chemistry, supramolecular chemistry, macromolecular chemistry and photochemistry. Recently, the metal-catalyzed [2 + 2 + 2]-type cycloaddition reaction of alkynes and nitriles has attracted considerable interest as a straightforward, atom-economical route to substituted pyridines and methods have been extensively applied to bipyridine synthesis. Developments in the field of 2,2’-bipyridine synthesis through alkyne/nitrile [2 + 2 + 2] cycloaddition reactions catalyzed by transition metals are reviewed in this article involving two types of fully intramolecular reaction, five types of partially intramolecular reaction and fully intermolecular reactions.CONTENTS
1. Introduction
2. Fully Intramolecular Reactions
3. Partially Intramolecular Reactions
3-1. Starting from Substrates Bearing a Pyridine Unit
3-1-1. 2-Cyanopyridines
3-1-2. Alkynes Bearing a 2-Pyridyl Group
3-2. Double Cycloaddition Reactions
3-3. Terpyridine and Quarterpyridine Formation
4. Fully Intermolecular Reactions
5. Mechanism and Regioselectivity
6. Appendix
7. Conclusion
1. INTRODUCTION
2,2’-Bipyridines are of great importance in the fields of catalysis, coordination chemistry, supramolecular chemistry, macromolecular chemistry, and photochemistry. They form a part of the substructure or main component in biologically active natural and artificial compounds, and in particular are versatile as a ligand capable of attaching to various metal atoms. Their metal complexes exhibit unique characteristics allowing them to fulfill a variety of synthetically useful roles such as a catalyst and its precursor, the substructure of polymers or self-assembling molecules, photosensitizer, and optical device materials.1 Therefore, many synthetic methods consisting of non-catalyzed heterocycle syntheses2 and metal-catalyzed reactions have been developed. In modern organic synthesis, bipyridines have been prepared by transition metal-catalyzed homocoupling (Ullmann-type reactions) of 2-halopyridine or cross-coupling between 2-halo and 2-metalated pyridines.3 However, in general, these processes are not necessarily applicable and are limited by the availability and reactivity of the halo- and/or metalated pyridine substrates. Recently, the metal-catalyzed [2 + 2 + 2]-type cycloaddition reaction of alkynes and nitriles has attracted considerable interest as a straightforward, atom-economical route to substituted pyridines,4 and the methods have been extensively applied to bipyridine synthesis. Developments in the field of 2,2’-bipyridine synthesis through alkyne/nitrile [2 + 2 + 2] cycloaddition reactions catalyzed by transition metals will be reviewed in this article.
Substituted pyridines can be synthesized from alkyne and nitrile substrates through three types of [2 + 2 + 2] cycloaddition reaction (Scheme 1); i.e., a fully intramolecular reaction (Equation 1), two partially intramolecular reactions (Equations 2 and 3), and a fully intermolecular reaction (Equation 4).
Based on these three types of pyridine formation as shown in equations 1-4, the examples of 2,2’-bipyridine synthesis reported so far can be classified into two types of fully intramolecular reaction (Scheme 2), five types of partially intramolecular reaction (Scheme 3) and fully intermolecular reactions (Scheme 4).
Synthesis of 2,2’-bipyridines via fully intramolecular reactions involves the reaction of diyne—nitrile substrates bearing 2-pyridyl moiety at the terminal position (Equation 5) and the double cycloaddition reaction of α,ω-dicyanotetraynes (Equation 6), both of which have no chemo- or regiochemical problem when having appropriate tether lengths.
The partially intramolecular reactions illustrated in Scheme 3 consist of three types of reaction using substrates bearing a 2-pyridyl group (Equations 7-9) and two types of double cycloaddition reaction (Equations 10 and 11). The addition of α,ω-diynes with 2-cyanopyridine can infallibly provide 2,2’-pyridines, but two regioisomeric products may be formed (Equation 7). Meanwhile, in the additions of diynes bearing a terminal 2-pyridyl moiety with nitriles and of α,ω-cyanoalkynes with 2-pyridylacetylenes, 2,2’- and 2,3’-isomers can be produced competitively (Equations 8 and 9). The regiochemical control is also important for the selective formation of 2,2’-bipyridienes in the double cycloadditions of tetraynes with nitriles and of α,ω-cyanoalkynes with 1,3-diynes (Equations 10 and 11).
Selective formation of 2,2’-bipyridenes by a fully intermolecular reaction is restricted to a few examples of the reaction of two molecules of alkynes and 2-cycanopyridine because of intrinsic difficulties in the selective cross-addition of two alkynes and a nitrile over the formation of benzenes.
As an additional caution, the generated 2,2’-bipyridine can affect the catalysis by its tendency to coordinate to the metal species. In fact, a few catalysts did not work well; therefore, inactivation by trapping of the metal catalyst by the bipyridine product is rationalized.
2. FULLY INTRAMOLECULAR REACTIONS
Roglans and co-workers reported the formation of bis-annulated 2,2’-bipyridine 2 from diyne—nitrile 1 with a nitrogen tether, which was effectively cycloadded intramolecularly in the presence of a Rh-catalyst (Wilkinson catalyst) under microwave irradiation conditions (a reaction of the same type as Equation 5).5 In the study, synthesis of bipyridine 4 by the double intramolecular cycloaddition reaction of 1,22-dicyano-5,10,12,17-tetrayne 3 under identical reaction conditions was also described (a reaction of the same type as Equation 6).
3. PARTIALLY INTRAMOLECULAR REACTIONS
3-1. Starting from Substrates Bearing a Pyridine Unit
3-1-1. 2-Cyanopyridines
The [2 + 2 + 2] cycloaddition reactions of α,ω-diynes with 2-cycnopyridine derivatives are a reliable approach to 2,2’-bipyridines without regiochemical concerns, as first demonstrated by Saá et al. in 2003 (Scheme 6, a reaction of the same type as Equation 7).6 In the presence of 10 mol% of a cationic Ru-catalyst [Cp*Ru(MeCN)3]PF6 (Cp* = η5-pentamethylcyclopentadienyl)], α,α-dipropargyl malonate 5a reacted with 2,6-dicyanopyridine (6a) selectively to produce 2,2’-bipyridine 7aa in a good yield. Note that under these conditions, neither 2-cyanopyridine nor 7aa reacted with 5a, and therefore, 7aa was selectively formed from 5a and 6a without further reaction of the remaining cyano moiety.
Table 1 summarizes the results of the cycloaddition of 2-cyanopyridine (6b) with various 1,6-diynes 5 reported by our group, in which the reaction was catalyzed by a dppe/CoCl2-6H2O/Zn reagent (dppe = 1,2-bis(diphenylphosphino)ethane) (a reaction of the same type as Equation 7).7-9 Symmetrical diynes 5b, 5c, and 5k were converted to the corresponding 2,2’-bipyridines in moderate-to-good yield.
Unsymmetrical diynes 5d—j also reacted smoothly with 6b, and it is noteworthy that the reactions provided the corresponding 2,2’-bipyridine with complete regioselectivity (runs 3—9). In the reactions of alkyl or silyl mono-substitute diynes 5d—f (R2 = H), the substituent R1 was located at the 3-position of the generated pyridine ring (runs 3—5). Meanwhile, the reaction of 5g gave the product 2,2’-bipyridine 8gb exclusively, with a phenyl group at the 2-position of the resulting pyridine ring (run 6). In addition, unsymmetrical diynes with two internal alkynes 5h—j also reacted with 6b in a completely regioselective fashion. Note that silyl-substituted internal diynes such as 5i and 5j exclusively yielded 3-silyl-2,2’-bipyridines 7, independent of the substituent R2. It was suggested that the regiochemistry was controlled by the electronic nature of the diynes.8
Deiters and co-workers have reported the synthesis of 3-(2-pyridyl)-2-aza-anthracene 9, as a new fluorescent molecule, via its dihydro derivative 7mb prepared by microwave-assisted CpCo(CO)2-catalyzed cycloaddition of 1,2-dipropargylbenzene 5m and 2-cyanopyridine (6b) (Scheme 7).10
Malacria, Aubert, Gandon, and co-workers reported synthesis of 2,2’-bipyridiene 10 having a 1,2,3,4-tetrahydroquinoline structure by a CpCo(CO)(dmfu)-catalyzed reaction of nitrogen-tethered 1,7-diyne 5n with 6b (Cp = η5-cyclopentadienyl, dmfu = dimethyl fumarate) (Scheme 7, a reaction of the same type as Equation 7).11 The authors suggested that the moderate yield (50%) may be due to the chelating nature of the bipyridine scaffold that is capable of trapping the catalyst. The regioselectivity was exclusive, but the sole generation of 2-silyl substituted pyridine differed from the results of runs 8 and 9 in Table 1. The production of 10 may be rationalized by steric constraints.
3-1-2. Alkynes Bearing a 2-Pyridyl Group
The bipyridine synthesis starting from alkynes bearing a 2-pyridyl moiety shown in Equations 8 and 9 in Scheme 3 has been most extensively explored. It is necessary to consider the regiochemical selectivity because these reactions have the potential to provide both 2,2’- and 2,3’-bipyridines.
The cycloaddition of 6-(2-pyridyl)-1,6-diynes 11 with nitriles may yield the 2,2’-bipyridides 13 and its 2,3’-isomer (Equation 8). We have demonstrated exclusive production of 2,2’-bipyridine from the pyridyl—diynes 11 and nitrile 12 with use of a dppe/CoCl2-6H2O/Zn catalyst (Table 2).7 2-Pyridyl-diynes 11 bearing an internal or terminal alkyne moiety were converted to the 2,2’-bipyridines 13 as a single isomer in moderate-to-good yield.
Recently, Nissen and Detert have elegantly applied a Cp*RuCl(cod)-catalyzed [2 + 2 + 2] cycloaddition to the total synthesis of lavendamycin, a bacterial-derived antitumor antibiotic, as one of the key reactions (cod = 1,4-cyclooctadiene) (Scheme 8, a reaction of the same type as Equation 8).12 Thus, the Ru-catalyzed reaction of highly functionalized 2-pyridyl-diyne 14a with methyl cyanoformate 12e furnished the desired 2,2’-bipiridine derivative 15 exclusively in excellent yield. The origin of the excellent regioselectivity of Ru-catalyzed reaction has been rationalized by considering the [2 + 2] addition of the electron-deficient nitrile to metallacyclic intermediate A. In the [2 + 2] adduct C, the sterically more demanding substituent Ar will come close to the bulky Cp* ligand. In contrast, the formation of B will avoid this steric repulsion and lead to the formation of 2,2’-isomer 15. It was reported that the Rh-catalyzed reactions of 14a and 12e gave the 2,3’-isomer predominantly, the ratio of which was varied by the reaction temperature.
Interestingly, Cp*RuCl(cod)-catalyzed reactions of similar but more simple substrate 14b did not produce the corresponding 2,2’-bipyridine derivative, which was explained by assuming that the bipyridyl moiety in the product inhibits the catalytic cycle by complexation to the catalyst (Scheme 8). On the other hand, the reaction 3-pyridyl derivative 14c underwent cycloaddition with an exclusive regioselectivity to give the corresponding β-carboline in 70% yield.13
The pioneering work by Castedo and Saá in the field of bipyridine synthesis via [2 + 2 + 2] cycloaddition involves the reactions of 5-hexynenitrile (17a) or 6-heptynenitrile (17b) with 2-pyridylacetylene derivatives 18 providing 2,2’- and 2,3’-bipyridines (Scheme 9, a reaction of the same type as Equation 9),14 which were catalyzed by CpCo(CO)2 under photo-irradiation conditions. Saá’s group also reported the selective formation of bis-annulated 2,2’-bipyridine 22 but in a low yield with recovery of 40% of 21 (Scheme 10).15
In 2010, Siegel and co-workers reported an elegant application of the cycloaddition of 6-heptynenitrile and 2-pyridylacetylene derivatives to the total synthesis of (+)-complanadine A,16 which was identified in a screen of compounds from the club moss Lycopodium complanatum and induces secretion of neurotrophic factors from 1321N1 cells, promoting the differentiation of PC-12 cells (Scheme 11, a
reaction of the same type as Equation 9). Under CpCo(CO)2-catalyzed reaction conditions, the 6-heptynenitrile derivative 23 could undergo cycloaddition with the 2-(2-trimethylsilylethyneyl)pyridine derivative 24 to produce a mixture of 2,2’- and 2,3’-bipyridenes 25 and 26, the latter of which is suitable for the synthesis of complanadine A. Interestingly, the authors found that the regioselectivity could be changed by the addition of PPh3. The ability of PPh3 to change the regioselectivity warrants further study.
Recently, Louie and co-workers have developed the first homogeneous iron complex-catalyzed [2 + 2 + 2] cycloaddition reaction of 5-hexynenitrile or 6-heptynenitrile derivatives with internal alkynes, in which the production of a mixture of 2,2’- and 2,3’-bipyridines from the 5-hexynenitrile derivative 27 and 2-alkynylpyridine 28 was reported (Scheme 12, a reaction of the same type as Equation 9).17 Based on the results of the reaction of 27 with other unsymmetrical alkynes, it can be assumed that the regioselectivity may be controlled electronically as well as sterically.
3-2. Double Cycloaddition Reactions
The bipyridine preparation by a double (domino) [2 + 2 + 2] cycloaddition depicted in Equations 10 and 11 in Scheme 3 involves the reaction of 1,6,8,13-tetraynes 32 with nitriles (Scheme 13 and Table 3) and that of 5-hexynenitrile (17a) with 1,3-diynes (34) (Table 4), both of which have an intrinsic regiochemical issue; i.e., the possibility of the production of three different regioisomers, 2,2’-, 2,3’-, and 3,3’-bipyridines.
In 2001, Itoh and co-workers reported the exclusive production of 2,2’-bipyridines from tetrayne 32a and nitriles 12b or 12f, which was effectively catalyzed by Cp*RuCl(cod). For successful cycloaddition, the nitrile substrate requires a coordinative group such as CN or Cl at the α position.18,19
Table 3 summarizes the results of the cobalt-catalyzed reaction of various tetraynes 32 and nitriles 12, reported by Kotora et al.,20 and our group7,8 in 2008 (Table 3). Both of the catalyst systems, (i.e., dppe/CoCl2-6H2O/Zn (Method A, runs 1—5) and CpCo(CO)2/microwave (Method B, runs 6—14)), could furnish the corresponding bipyridines 33 with exclusive 2,2’-selectivity.
Some of the products have been oxidized to the corresponding axially chiral bis-N-oxide compounds, which were resolved to their optically active form, and were applied to asymmetric allylation reactions as a chiral Lewis base-catalyst (Scheme 14).20
Another type of double cyclization has been reported by Saá’s group in 1998,15,21 which cycloadded two molecules of 5-hexynenitrile (17a) to 1,3-diynes 34 (Table 4, a reaction of the same type as Equation 11).
Despite the possibility of producing three regioisomers, the reaction actually yielded only a mixture of 2,2’- and 2,3’-bipyridines 35 and 36. It was realized that employing 1,3-diynes bearing coordinative oxygen or nitrogen functionalities at the propargylic positions, such as 34c and 34h, resulted in a complete regiocontrol giving 2,2’-isomer (runs 3 and 8). In the reaction of the sterically demanding 1,3-diyne 34a, one of the triple bonds of the diyne could be associated to the cycloaddition (run 1).
3-3. Terpyridine and Quarterpyridine Formation
Based on the bipyridine formation processes mentioned above, several applications in the production of terpyridines and quarterpyridines have been demonstrated.
In 1997, Castedo and Saá reported pioneering work on the formation of terpyridines by CpCo(CO)2-catalysis with 6-hexynenitrile (17a) and 2,6-dialkynylpyridiene 41 via double cycloaddition (Scheme 15). The reaction resulted in the production of a mixture of 2,2’:6’,2”-, 2,2’:6’,3”-, and 3,2’:6’,3’-isomers, 42, 43, 44, with the co-production of the mono-cyclized product 45.14
The highly selective preparation of 2,2’:6’,2”-terpyridine 46 has been demonstrated by us, where a diyne bearing a 2-pyridyl substituent cycloadded with 2-cyanopyridine (6b) in the presence of a dppe/CoCl2-6H2O/Zn catalyst (Scheme 16).7 We have also attempted the synthesis of quarterpyridine 47 by the double cycloaddition reaction of tetrayne 32d with two molecules of 2-cyanopyridine (6b), but the reaction yielded only 5% of quarterpyridine 47 (a single regioisomer) with co-production of the mono- cyclized product 48 (Scheme 17). The low reaction rate of the second cycloaddition reaction is presumably because of a steric factor. The separated 48 was converted into 2,2’:6’,2”-terpyridine 49 by reaction with another nitrile by the same catalyst system, in which complete regioselectivity was again observed.
4. FULLY INTERMOLECULAR REACTIONS
Pyridine synthesis by fully intermolecular alkyne/nitrile [2 + 2 + 2] cycloaddition is not easy because it requires high levels of chemo- and regioselectivities. To date, the reported synthesis of 2,2’-bipyridines in this method (a reaction of the same type as Equation 12) has been restricted to the reaction of two molecules of an alkyne with a nitrile.
In 1994, Chelucci et al. reported that CpCo(cod) catalyzed cocyclotrimerization of 2,6-dicyanopyridine (6a) with acetylene to give 2,2'-bipyridine-6-carbonitrile (50) in 70% yield (Scheme 18).22 The method has been applied to the synthesis of optically active bipyridine-phosphine compound 53 (Scheme 19).23 A chiral cyanopyridine 51 reacted with acetylene in the presence of CpCo(cod) to furnish optically active bipyridine 52 in 75% yield, which was converted to 53.
In addition, a report of the cobalt- or palladium-catalyzed synthesis of 3,4,5,6-tetraphenyl-2,2’-bipyridine (55) by co-cycloaddition of tolan (54) and 2-cyanopyridine (6b) by Uhm and An can be found in a journal online search (Scheme 20), although the details are unknown.24
5. MECHANISM AND REGIOSELECTIVITY
In the several reports mentioned above, the origin of the regioselectivity was discussed by consideration of the electronic and/or steric factors. As illustrated in Scheme 8, Cp*RuCl(cod)-catalyzed reactions might be controlled mainly by the steric factor in the reacting intermediate(s).
As shown in Scheme 21, the CpCoI-catalyzed reactions of hexynenitrile 17a with (2-pyridyl)alkynes 18 generally afford a mixture of 2,2’- and 2,3’-isomers (Scheme 9, 11 and 15), which may proceed via both intermediates D and E. For the reaction of 17a with 1,3-diynes 34 (Table 4), based on the B3LYP/LANL2DZ ab initio calculations (R = SiH3 or H), Saá et al. suggested that the cobaltacycle F is more stable than its isomer G. In fact, when R was a sterically demanding SiMe3, the reaction yielded a mono-cycloadded product 37 as a sole isomer (run 1 in Table 4). The intermediate F gives the compound of type 18, which is further reacted with 17a. When diyne 34 has coordinative substituents (R) such as CH2OH and CH2NMe2, the second cycloaddition proceeds predominantly through an intermediate D’, which can be stabilized by the coordination of a directing group at the 3-position of the first-generated pyridyl moiety to the cobalt, to yield 2,2’-isomer 35.
Regarding a dppe/CoCl2·6H2O/Zn catalyst developed by us, we postulate that the pyridine formation may proceed through a (dppe)CoICl complex generated by the reduction of (dppe)CoCl2 with zinc, which might react with diynes 5 or 11 to provide cobaltacyclopentadiene intermediates H or J, respectively (Scheme 22). The regioselectivity can be systematically rationalized by considering differences in the
electronic nature between the diynes’ two alkynes. Thus, a relatively electron-rich alkyne moiety in diynes 5 or 11 was bonded to the electron-poor position (carbon atom) of the cyano group in nitriles. It is plausible that an intermediate cobaltacycle of the type H or J derived from the corresponding 5 or 11 might have a similar electronic nature influenced by the substituents R1 and R2 in the reaction proceeding through [4 + 2] cycloaddition and/or insertion pathways. Thus, the regioselectivity can be predicted accurately by ordering the electronic nature of alkynes as Me3Si-, alkyl-, H-, Ph-, C≡C-, and 2-pyridyl-substituted alkynes from electron rich to electron poor. The double cycloaddition reactions of tetraynes 32 with nitriles are the case: the first cycloaddition may proceed similarly to the reaction of 5 through an intermediate K and the resulting adduct further reacts with a nitrile like the reaction of 11. Overall, the regioselectivity may be altered mainly by electronic effects. In conclusion, the consideration of the regiochemical trend lends credence to our method’s synthetic potential, although confirmation of the reaction mechanism involving the conversion of metallacycle intermediates H, J or K to pyridines will require further studies.
6. APPENDIX
This section adds several interesting topics, highly related to synthesis of 2,2’-bipyridines by a cycloaddition reaction.
Although the following two examples are not for formation of 2,2’-bipyridines, Co- and Rh-catalysts could effectively convert malononitrile derivatives 56, 57, and 60 to the unique spiro compounds, i.e., tetrahydro-7,7’-spirobi[cyclopenta[b]pyridine]s and tetrahydro-8,8’-spirobi[quinoline]s, by a [2 + 2 + 2] double cycloaddition reaction of nitrile and two alkynes (Scheme 23 and Scheme 24).
Saá et al. first demonstrated synthesis of 7,7’-spirobi[cyclopenta[b]pyridine]s 58 and 8,8’-spirobi[quinoline]s 59 from dialkylated malononitirles 56 and 57, respectively, by treatment with a CpCo(cod) or CpCo(CO)2 catalyst in a refluxed toluene (Scheme 23).25 For the reaction with acetylene (R1, R2 = H) as an alkyne counterpart, use of CpCo(cod) gave a better yield. The moderate or low yields of these reactions were explained by assuming that the gem-dinitrile unit of malononitriles 56 and 57 may reduce the donor character of the nitrile, disfavoring stabilization of the cobaltacycle intermediate. They also studied formation of coordination complexes with use of the resulting racemic 7,7’-spiropyridine 58 (R1 = R2 = H, n = 1). Thus, the tetra-coordinate complex 58-Cu was obtained as a diastereomeric mixture when [CuI(CH3CN)]PF6 was added to solution of (±)-58 in CH2Cl2 at room temperature.
Tanaka and co-workers have reported that a fully intramolecular double cycloaddition of dicyano-tetrayne
compounds 60 catalyzed by [Rh(cod)2]BF4 in the presence of a chiral diphosphine such as (R)-Segphos and (R)-H8-BINAP proceeded smoothly in CH2Cl2 at ambient temperature to provide the corresponding 7,7’-spirobi[cyclopenta[b]pyridine]s and 8,8’-spirobi[quinoline]s 61 in good-to-excellent yields as an optically active form (up to 71% enantiomeric excess) (Scheme 24).26 In contrast to the cobalt-catalyst systems mentioned in Scheme 23, the Rh-catalyst system could not cycloadd dicycano-diyne 56a to an internal alkyne intermolecularly, and the compound 56a was recovered even at elevated temperature.
In the report of selective pyridine formation from two different unsymmetrical alkynes and a nitrile developed by Takahashi’s group, an efficient chemoselective and regioselective synthesis of 2,2’-bipyridine 62 from 2-cyanopyridine (6b) and two different alkynes has been reported, albeit the process is not catalytic and requires a stoichiometric amount of zirconium reagent (Scheme 25).27
Xi and co-workers synthesized 4,5-disubstituted 2,2’-bipyridine 65 starting from 1-halo-1,3-diene 64 by lithiation and the following addition reaction with 2-cyanopyridine (6b) (Scheme 26).28 The 1-halo-1,3-diene 64 may be prepared from trimethylsilylalkynes by zirconium-mediated reaction, and therefore, the overall processes are a formal [2 + 2 + 2] cycloaddition of 2-cyanopyridine and alkynes, albeit it needs a stoichiometric amount of metallic reagents.
7. CONCLUSION
In recent years, the transition metal-catalyzed de novo synthesis of 2,2’-bipyridines by [2 + 2 + 2] cycloaddition of alkynes and nitriles has been evolving as a versatile method in synthetic chemistry. In general, the reactions proceed through the formation of metallacyclopentadiene intermediates and subsequent insertion or [4 + 2] cycloaddition of another unsaturated bond. In several reaction types, regioisomeric bipyridine products can be generated with a selectivity determined by the regiochemistry of the metallacyclopentadiene formation and/or the following reaction of another unsaturated bond. With the advances accounted here, an efficient, straightforward, and atom-economical construction of 2,2’-bipyridines as well as several high regioselectivities have been realized in fully and partially intramolecular reactions. Meanwhile, the fully intermolecular reaction is still not much explored. In particular, the regioselective catalytic assembly of two different unsymmetrical acetylenes and a nitrile, leading to a single pyridine product, still remains a challenge.
In addition, the results of the reports reviewed here may be a prompt to investigate bipyridine synthesis by other catalytic systems active for an alkyne/nitrile [2 + 2 + 2] cycloaddition reaction, such as N-heterocyclic carbene/Ni(cod)2,29 Xantphos/Ni(cod)2,30 and dppp/FeI2/Zn.31 The development of new catalytic reactions applicable to bipyridine synthesis in this fashion is also expected.
References
1. J. G. Cordaro, J. K. McCusker, and R. G. Bergman, Chem. Commun., 2002, 1496; CrossRef and references cited therein. R. W. J. Chubb, M. R. Bryce, and B. Tarbit, J. Chem. Soc., Perkin Trans. 1, 2001, 1853; CrossRef J. G. Cordaro, J. K. McCusker, and R. G. Bergman, Chem. Commun., 2002, 1496; CrossRef C. Kaes, A. Katz, and M. W. Hosseini, Chem. Rev., 2000, 100, 3553; CrossRef K. T. Potts, K. A. Gheysen Raiford, and M. Keshavarz-K, J. Am. Chem. Soc., 1993, 115, 2793; CrossRef E. Constable, Tetrahedron, 1992, 48, 10013; CrossRef V. Grosshenny, A. Harriman, and R. Ziessel, Angew. Chem., Int. Ed. Engl., 1995, 34, 2705; CrossRef P. Belser, R. Dux, M. Baak, L. De Cola, and V. Balzani, Angew. Chem., Int. Ed. Engl., 1995, 34, 595; CrossRef A. Livoreil, C. O. Dietrich-Buchecker, and J.-P. Sauvage, J. Am. Chem. Soc., 1994, 116, 9399; CrossRef F. Barigelletti, L. Flamigni, V. Balzani, J.-P. Sauvage, A. Sour, E. C. Constable, and A. M. W. Cargill Thompson, J. Am. Chem. Soc., 1994, 116, 7692; CrossRef C. Dhenaut, I. Ledoux, I. D. W. Samuel, J. Zyss, M. Bourgault, and H. Le Bozec, Nature, 1995, 374, 339; CrossRef Y. Eichen, J.-M. Lehn, M. Scherl, D. Haarer, J. Fischer, A. DeCian, A. Corval, and H. P. Trommsdorff, Angew. Chem., Int. Ed. Engl., 1995, 34, 2530; CrossRef D. Gust, Nature, 1994, 372, 133; CrossRef J. Zyss, Molecular Nonlinear Optics: Materials, Physics and Devices; Academic Press: Boston, 1993.
2. S. P. Stanforth, B. Tarbit, and M. D. Watson, Tetrahedron Lett., 2003, 44, 693; CrossRef T. Shintou, F. Ikeuchi, H. Kuwabara, K. Umihara, and I. Itoh, Chem. Lett., 2005, 34, 836 and cited therein. CrossRef
3. M. Hapke, L. Brandt, and A. Lützen, Chem. Soc. Rev., 2008, 37, 2782; CrossRef T. D. Nelson and R. D. Crouch, Organic Reactions, 2004, 63, 265; A. A. Fuller, H. R. Hester, E. V. Salo, and E. P. Stevens, Tetrahedron Lett., 2003, 44, 2935 and cited therein. CrossRef
4. Reviews for pyridine formation by the transition-metal-catalyzed [2 + 2 + 2] cycloaddition reactions: H. Bönnenmann, Angew. Chem., Int. Ed. Engl., 1985, 24, 248; CrossRef J. A. Varela and C. Saá, Chem. Rev., 2003, 103, 3787; CrossRef I. Nakamura and Y. Yamamoto, Chem. Rev., 2004, 104, 2127; CrossRef P. R. Chopade and J. Louie, Adv. Synth. Catal., 2006, 348, 2307; CrossRef B. Heller and M. Hapke, Chem. Soc. Rev., 2007, 36, 1085; CrossRef B. Heller and M. Hapke, Chem. Soc. Rev., 2007, 36, 1085. CrossRef
5. L. Gaarcia, A. Pla-Quintana, A. Rogalans, and T. Parella, Eur. J. Org. Chem., 2010, 3407. CrossRef
6. J. A. Varela, L. Castedo, and C. Saá, J. Org. Chem., 2003, 68, 8595. CrossRef
7. A. Goswami, K. Ohtaki, K. Kase, T. Ito, and S. Okamoto, Adv. Synth. Catal., 2008, 350, 143. CrossRef
8. Y. Sugiyama and S. Okamoto, Synthesis, 2011, 2247. Errata: 2011, 3928. CrossRef
9. J. Watanabe, Y. Sugiyama, A. Nomura, S. Azumatei, A. Goswami, N. Saino, and S Okamoto, Macromolecules, 2010, 43, 2213. CrossRef
10. Y. Zou, D. D. Young, A. Cruz-Montanez, and A. Deiters, Org. Lett., 2008, 10, 4661. CrossRef
11. P. Garcia, Y. Evanno, P. George, M. Sevrin, G. Ricci, M. Malacria, C. Aubert, and V. Gandon, Org. Lett., 2011, 13, 2030. CrossRef
12. F. Nissen and H. Detert, Eur. J. Org. Chem., 2011, 2845. CrossRef
13. F. Nissen, V. Richard, C. Alayrac, and B. Witulski, Chem. Commun., 2011, 47, 6656. CrossRef
14. J. A. Varela, L. Castedo, and C. Saá, J. Org. Chem., 1997, 62, 4189. CrossRef
15. J. A. Varela, L. Castedo, M. Maestro, J. Mahía, and C. Saá, Chem. Eur. J., 2001, 7, 5203. CrossRef
16. C. Yuan, C.-T. Chang, A. Axelrod, and D. Siegel, J. Am. Chem. Soc., 2010, 132, 5924. CrossRef
17. B. R. D’Souza, T. K. Lane, and J. Louie, Org. Lett., 2011, 13, 2936. CrossRef
18. Y. Yamamoto, R. Ogawa, and K. Itoh, J. Am. Chem. Soc., 2001, 123, 6189. CrossRef
19. Y. Yamamoto, K. Kinpara, R. Ogawa, H. Nishiyama, and K. Itoh, Chem. Eur. J., 2006, 12, 5618. CrossRef
20. R. Hrdina, M. Dracínsky, I. Valterová, J. Hodacová, I. Císarová, and M. Kotora, Adv. Synth. Catal., 2008, 350, 1449. CrossRef
21. J. A. Varela, L. Castedo, and C. Saá, J. Am. Chem. Soc., 1998, 120, 12147. CrossRef
22. G. Chelucci, M. A. Cabras, and A. Saba, J. Heterocycl. Chem., 1994, 31, 1289. CrossRef
23. G. Chelucci, M. A. Cabras, C. Botteghi, and M. Marchetti, Tetrahedron: Asymmetry, 1994, 5, 299; CrossRef G. Chelucci, Tetrahedron: Asymmetry, 1995, 6, 811. CrossRef
24. J.-K. Uhm and H. W. An, J. Korean Chem. Soc., 2001, 45, 268.
25. J. A. Varela, L. Castedo, and C. Saá, Org. Lett., 1999, 1, 2141. CrossRef
26. A. Wada, K. Noguchi, M. Hirano, and K. Tanaka, Org. Lett., 2007, 9, 1295. CrossRef
27. T. Takahashi, F.-Y. Tsai, Y. Li, H. Wang, Y. Kondo, M. Yamanaka, K. Nakajima, and M. Kotora, J. Am. Chem. Soc., 2002, 124, 5059. CrossRef
28. C. Wang, Z. Wang, L. Liu, C. Wang, G. Liu, and Z. Xi, J. Org. Chem., 2006, 71, 8565. CrossRef
29. M. M. McComick, H. A. Duong, G. Zuo, and J. Louie, J. Am. Chem. Soc., 2005, 127, 5030; CrossRef R. M. Stolley, M. T. Maczka, and J. Louie, Eur. J. Org. Chem., 2011, 3815. CrossRef
30. P. Kumar, S. Prescher, and J. Louie, Angew. Chem. Int. Ed., 2011, 50, 10694. CrossRef
31. C. Wang, X. Li, F. Wu, and B. Wan, Angew. Chem., 2011, 123, 7300. CrossRef