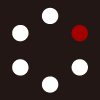
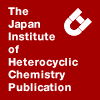
HETEROCYCLES
An International Journal for Reviews and Communications in Heterocyclic ChemistryWeb Edition ISSN: 1881-0942
Published online by The Japan Institute of Heterocyclic Chemistry
e-Journal
Full Text HTML
Received, 7th May, 2012, Accepted, 27th June, 2012, Published online, 2nd July, 2012.
DOI: 10.3987/REV-12-742
■ Synthetic Studies on Natural Pterin Glycosides
Tadashi Hanaya* and Hiroshi Yamamoto
Department of Chemistry, Faculty of Science, Okayama University, 3-1-1 Tsushima-naka, Okayama 700-8530, Japan
Abstract
Some pterins having various kind of sugars attached to the hydroxyalkyl side-chain at C-6 are known to occur in certain prokaryotes as exemplified by 2'-O-(α-D-glucopyranosyl)biopterin isolated from various kinds of cyanobacteria. A synthetic exploration of various types of glycosides of biopterin and related pterins has been undertaken owing to a marked interest in their physiological functions and biological activities as well as the structural proof of those natural products. This review summarizes our synthetic studies on natural pterin glycosides by employing the appropriately protected N2-(N,N-dimethylaminomethylene)-3-[2-(4-nitrophenyl)ethyl]pterin derivatives as glycosyl accepters.CONTENTS
1. Introduction
2. Synthesis of biopterin glycosides
2-1. Synthesis of 2'-O-(β-D-ribofuranosyl)- and 2'-O-(β-D-glucopyranosyl)biopterin (1c, 1e)
2-2. Synthesis of limipterin (1d)
2-3. Synthesis of 2'-O-(α-D-glucopyranosyl)biopterin (1b)
3. Synthesis of ciliapterin, neopterin, and 6-hydroxymethylpterin glycosides
3-1. Synthesis of 2'-O-(α-D-mannopyranosyl)ciliapterin (2b) and tepidopterin (2c)
3-2. Synthesis of 3'-O-(α-D-glucopyranosyluronic acid)neopterin (3b)
3-3. Synthesis of 6-hydroxymethylpterin α- and β-D-glucopyranosides (4b)
3-4. Synthesis of asperopterin-A (5b)
4. Conclusion
5. References
1. INTRODUCTION
Various pterin derivatives have been isolated from many living organisms: e.g., microorganisms, algae, insects, fish, amphibian, and mammals.1 Among them, some pterins having a hydroxyalkyl side-chain at C-6, such as biopterin (1a), ciliapterin (2a), and neopterin (3a), have been found as glycosidic forms in certain prokaryotes (Figure 1). As examples of glycosides of biopterin (1a), 2’-O-(α-D-gluco- pyranosyl)biopterin (1b)2-5 and its β-D-ribofuranosyl analog (1c)6 were isolated from cyanobacteria and limipterin [2’-O-(2-acetamido-2-deoxy-β-D-glucopyranosyl)biopterin] (1d)7 was isolated from a green sulfur photosynthetic bacterium. Glycosides (2b,c, 3b,c, 4b,c) of other pterins such as ciliapterin (2a),8,9 neopterin (3a),10,11 and 6-hydroxymethylpterin (4a)12,13 were also isolated from cyanobacteria, anaerobic photosynthetic bacteria, and chemoautotrophic archaebacteria. On the other hand, asperopterin-A (5b)14 isolated from Aspergillus oryzae is a unique glycoside of 5a having an isoxanthopterin (7-xanthopterin) structure as a parent ring. Various other glycosides consisting of different pterins and sugar moieties have also been found in nature, although some of them
have remained unclear concerning the position and the anomeric structure of the glycosidic linkage.8,12
The physiological function of the parent pterins has been studied in detail: e.g., 1a exhibits enzyme cofactor activity in aromatic amino acid hydroxylation15 and nitric oxide synthesis16 as the form of its tetrahydro derivative, while neopterin (3a) has been shown to be a marker for the activation of cellular immunity or an inducer of apoptosis.17 By contrast, the functional roles of pterin glycosides have remained obscure, although some inhibitory activities against tyrosinase18 and photostabilization of photosynthetic pigments19 were reported for 1b. Despite a considerable interest from the viewpoint of their biological activities and functions as well as structural proof of hitherto reported natural products, there had been no report for preparation of pterin glycosides before our synthetic studies on them. For example, although biopterin α-D-glucoside (1b) is the most noteworthy among these pterin glycosides because of its abundant occurrence in various kinds of cyanobacteria, Anacystis nidulans,2 Oscillatoria sp.,3 Synechococcus sp.,4 and Spirulina platensis,5 no attempts at synthesis of 1b had been made since its first discovery in 1958. In this review, we summarize our synthetic studies on natural pterin glycosides, taking up glycosides of biopterin in the first half and those of other pterins in the latter half.
2. SYNTHESIS OF BIOPTERIN GLYCOSIDES
2-1. Synthesis of 2'-O-(β-D-ribofuranosyl)- and 2'-O-(β-D-glucopyranosyl)biopterin (1c and 1e)
In our initial studies on glycosylation of a side-chain hydroxy group, we had to devise suitable protecting and at the same time solubilizing groups for the pyrimidine ring and the side-chain hydroxy groups of the starting material biopterin (1a). Because of the effectively stabilized intramolecular hydrogen bondings in the solid state,20 many pterin derivatives including 1a are little soluble in nonpolar aprotic solvents in which glycosylation reactions smoothly proceed. To overcome this problem, we employed dimethylaminomethylene group for protection of 2-amino and 2-(4-nitrophenyl)ethyl (NPE) group for N(3) of the pteridine ring.21 Thus, 1a was converted in a four-step-procedure via the di-O-acetyl intermediate (6) into the sufficiently solubilized and versatile N2-(N,N-dimethylaminomethylene)- 3-NPE-biopterin (7) in 87% overall yield (Scheme 1). Introduction of NPE group was achieved under Mitsunobu's conditions [NPE alcohol, triphenylphosphine, and diethyl azodicarboxylate (DEAD)].
For the purpose of further raising the solubility of compound 7 in dichloromethane, 7 was temporarily silylated with 1,1,1,3,3,3-hexamethyldisilazane (HMDS) in the presence of ammonium sulfate, yielding the 1’,2’-di-O-trimethylsilyl derivative (8) quantitatively. Glycosylation of 8 with 2,3,4,6-tetra-O-benzoyl-α-D-glucopyranosyl bromide (9)22 in the presence of tin(IV) chloride resulted in the formation of a mixture of 2’-O-(β-D-glucopyranosyl) 10a (41%), 1’-O-glycosyl isomer 10b (15%) and the 1’,2’-di-O- glycoside 10c (14%). Removal of the protecting groups of the 2’-O-(β-D-glucopyranosyl) derivative (10a) was performed according to the following three-step-procedure: 10a was treated with sodium methoxide in methanol to cleave benzoyl groups, and then treated with aqueous ammonia-methanol to remove the N,N-dimethylaminomethylene group. The NPE group was then removed by the action of DBU in DMF, thus affording 2’-O-(β-D-glucopyranosyl)biopterin (1e)23 as the first synthetic example of pterin glycosides despite of the anomeric isomer of natural biopterin glycoside 1b.
Meanwhile, similar treatment of 8 with 1-O-acetyl-2,3,4,6-tetra-O-benzoyl-β-D-ribofuranose (11) afforded 2’-O-(β-D-ribofuranosyl) compound 12a (60%) along with a minor portion of the 1’,2’-di-O-glycoside 12c (18%). Deprotection of 12a by the same treatment described above provided natural product 2’-O-(β-D-ribofuranosyl)biopterin (1c).6
2-2. Synthesis of limipterin (1d)
The results shown in Scheme 1 prompted us to pursue an effective preparation of a suitably 1’-O-protected biopterin derivative in order to achieve complete 2’-O-glycosylation. Taking into consideration the available combination of protecting groups employed for the synthetic pathways, we chose the biopterin derivative (13) whose 1'-hydroxy group was protected with p-methoxybenzyl (PMB) group, as a key glycosyl acceptor. As shown in a retrosynthetic analysis for 13 outlined in Scheme 2, the pteridine ring formation of 13 would be achieved by the condensation of 2,5,6-triaminopyrimid-4(3H)-one (14) with the 3-O-protected pentos-2-ulose (15), which would be obtainable from the 3-O-protected 5-deoxy-L-arabinose (16). Compound 16 would be derived by two different synthetic routes, starting with L-rhamnose involving C-1 cleavage, or starting with D-xylose involving C-4 inversion and C-5 deoxygenation. Thus the efficient total synthesis of limipterin [2’-O-(2-acetamido-2-deoxy-β-D-glucopyranosyl)biopterin] (1d) was attempted by selective glycosylation of the appropriately protected biopterin derivative (13) as follows.24,25
A five-step synthetic conversion from L-rhamnose provided 4-O-PMB-6-deoxy-L-rhamnose (19) via the allyl (17)26 and prop-1-enyl glycosides (18) as shown in Scheme 3. Treatment of 19 with ethanethiol in the presence of p-toluenesulfonic acid in acetic acid gave the dithioacetal (20), which was then oxidized with m-chloroperbenzoic acid (mCPBA) to the corresponding sulfone (21). Degradation of 21 with dilute aqueous ammonia27 afforded 5-deoxy-3-O-PMB-L-arbinofuranose (16).24
Meanwhile, D-xylose also served as the starting material for preparation of 16: the 5-deoxy- 4-enofuranose derivative (23)28 (prepared from D-xylose in four steps) was stereoselectively hydrogenated to afford the 5-deoxy-L-arabinose derivative (24), which was then converted into 16. The selective oxidation of 2-hydroxy group of 16 with cupric acetate29 provided the 3-O-PMB-L-erythro-pentos-2-ulose (15).25
Condensation of 15 with sulfate of 14 in an aqueous sodium bicarbonate solution afforded a 78:22 mixture of the biopterin derivative 25a and its 7-substituted isomer 25b. Successive treatment of the mixture with N,N-dimethylformamide dimethyl acetal in DMF, with acetic anhydride in pyridine, and then with NPE alcohol (Mitsunobu reaction), gave the versatile biopterin derivative 26a (53% overall yield from 15) and the 7-substituted derivative 26b (15%). Methanolysis of 26a in the presence of sodium methoxide quantitatively provided the 1’-O-PMB derivative 13, an ideal precursor for 2’-O-monoglycosylation.
The structural assignment of 26a and 26b was achieved primarily on the basis of their 1H and 13C-NMR spectral data. The signals of C-6 and C-7 of 6-alkylpteridines generally appear at a similar field, whereas C-7 signals of 7-alkyl derivatives shifts to a lower field (ca. 20 ppm) from those of C-6.30 Therefore, the close values of 26a (C-6: δ 150.71, C-7: δ 149.88) and the distant values of 26b (C-6: δ 140.92, C-7: δ 159.98) indicate the 6-substituted pterin for the former and the 7-substituted pterin for the latter. These assignments are supported by the fact that H-7 signal (δ 8.96) of 26a appears at a lower field than that of H-6 of 26b (δ 8.78) because of the lower electron density of C-7 than that of C-6 due to the conjugation with the 4-oxo group.31
An efficient glycosylation was exemplified by the condensation of 13 with 3,4,6-tri-O-acetyl-2-deoxy-2-phthalimido-β-D-glucopyranosyl bromide (27)32 in the presence of silver triflate (2.2 mol equiv.) and tetramethylurea (TMU) (1.0 mol equiv.) in dichloromethane,33 affording 2’-O-β-D-glucopyranosyl derivative (28) as a sole product in 82% yield (Scheme 4). Removal of the protecting groups of 28 was carried out by successive treatment of DDQ (to cleave PMB group) and then methylamine (to remove the phthaloyl, N,N-dimethylaminomethylene, and acetyl groups), followed by the action of and acetic anhydride, to give the fully-acetylated derivative 29. Treatment of 29 with aqueous ammonia and then with DBU furnished limipterin (1d).25
2-3. Synthesis of 2'-O-(α-D-glucopyranosyl)biopterin (1b)
The stereoselective formation of the β-glycosides (10a–c, 12a–c, 28) from 8 and 13 (in Scheme 1,4) was mainly caused by participation of the 2-O-benzoyl and 2-N-phthaloyl groups of the glycosyl donor (9,11,27) through the formation of an acyloxonium ion intermediate.34 Accordingly, in order to avoid such a neighboring group participation in synthesis of biopterin α-D-glucoside (1b), we sought to introduce an ether substituent for protection of 2-OH of a glycosyl donor; thus PMB and acetyl groups were respectively chosen for protection of 2,3-OH and 4,6-OH of the glycosyl moiety. According to a synthetic route shown in Scheme 5, such an appropriately protected novel glycosyl donor (34) was prepared starting with penta-O-acetyl-β-D-glucopyranose (30) via 1-thio-β-D-glucopyranoside derivatives (31–33).35
Then, glycosylation of 13 was found to give the best result when it was treated with 4.0 mol equiv. of the glycosyl bromide (34) in dichloromethane in the presence of silver triflate (2.0 mol equiv.) and TMU (1.0 mol equiv.), affording an inseparable anomeric mixture (85:15) of the 2’-O-(α-D-glucopyranosyl)biopterin derivative (35a) and its β-anomer (35b) in 66% yield, along with the recovery of 13 (24%) (Scheme 6). Separation of these isomers was achieved by removal of PMB groups and the subsequent acetylation, affording the α-D-glucopyranoside (36a) in 51% (total yield from 13) and its β-anomer (36b) in 9%. The α-anomeric structure of 36a was derived from its J1,2 value (3.9 Hz) of 1H-NMR, while the larger J1,2 value (8.1 Hz) confirmed the β-form of 36b. Removal of the protecting groups of 36a was accomplished as usual, furnishing the desired 2’-O-(α-D-glucopyranosyl)biopterin (1b).35
We thus achieved the first synthesis of biopterin α-D-glucoside (1b) by use of the key intermediate 1’-O-PMB-biopterin derivative (13) and the novel glycosyl donor (34) to preferentially provide an α-glycoside.
3. SYNTHESIS OF CILIAPTERIN, NEOPTERIN, AND 6-HYDROXYPTERIN GLYCOSIDES
3-1. Synthesis of 2'-O-(α-D-mannopyranosyl)ciliapterin (2b) and tepidopterin (2c)
On the basis of the successful strategy for regioselective synthesis of biopterin 2’-O-glycosides (1b,d) by use of the 1'-O-protected precursor (13) in Schemes 4 and 6, preparation of ciliapterin 2’-O-glycosides (2b,c) was also attempted.25,36 The 1'-O-protected ciliapterin derivative (43), corresponding to the 1'-epimer of 13, was prepared by two synthetic routes starting with D-xylose or L-xylose (Schemes 7 and 8).
5-Deoxy-1,2-O-isopropylidene-α-D-xylofuranose (37)37 derived from D-xylose was converted into the enol acetate (38) (Scheme 7). Catalytic hydrogenation of 38 afforded the 3-O-acetyl-L-lyxofuranose derivative, which was subjected to deacetylation, p-methoxybenzylation, and acetal cleavage, providing 5-deoxy-3-O-PMB-L-lyxose (39). The selective oxidation of 39 with cupric acetate afforded L-threo-pentose-2-ulose derivative (40). Meanwhile, 5-deoxy-1,2-O-isopropylidene-α-L-xylofuranose (41)38 derived from L-xylose provided 5-deoxy-3-O-PMB-L-xylose (42), which was then oxidized to the 2-ulose 40.
The condensation of 40 with sulfate of 14 in an aqueous sodium bicarbonate solution gave a 75:25 mixture of ciliapterin derivative (41) and its 7-substituted isomer (Scheme 8). These products were, as in the cases of 26a,b from 25a,b (Scheme 3), converted into the corresponding 2’-O-acetyl-N2-(N,N-dimethylaminomethylene)-3-NPE-1’-O-PMB derivative (42) (52% overall yield from 40) and the 7-substituted derivative (17%).
Methanolysis of 42 with sodium methoxide provided the 1’-O-PMB derivative (43), a versatile precursor for the 2’-O-monoglycosylation. Efficient glycosylation of 43 was attained by the D-mannopyranosyl bromide (44)39 or the 2-deoxy-2-phthalimido-D-glucopyranosyl bromide (27) in the presence of silver triflate and TMU, affording the 2’-O-(α-D-mannopyranosyl)- (45) and 2’-O-(β-D-glucopyranosyl)- ciliapterin derivative (46), respectively. Deprotection of 45 and 46 by the similar treatment described in the preceding sections led to the first syntheses of ciliapterin α-D-mannopyranoside (2b) and tepidopterin (2c), respectively.
3-2. Synthesis of 3'-O-(α-D-glucopyranosyluronic acid)neopterin (3b)
As the first synthetic example of a natural neopterin glycoside, 3'-O-(α-D-glucopyranosyluronic acid)neopterin (3b) was prepared starting with neopterin (3a)40 (available from D-arabinose), as illustrated in Scheme 9.41 Namely, the key precursor (48), whose pyrimidine moiety and 1’,2’-hydroxy groups of the side chain were protected, was prepared from 3a via 47 in five steps. Treatment of 48 with the methyl D-glucopyranosyluronate bromide (49)42 in the presence of silver triflate and TMU afforded 3’-O-(β-D-glucopyranosyluronate)neopterin derivative (50) in 64% yield, along with the recovery of 48 (26%).
For removal of the protecting groups of 50, attempted hydrolysis of all ester groups by use of aqueous sodium hydroxide resulted in the formation of an inseparable mixture of unidentified products. However, selective cleavage of methyl ester of 50 was achieved by use of lithium iodide in pyridine, followed by the action of sodium methoxide, providing the 3’-O-(β-D-glucopyranosyluronic acid)neopterin derivative (51). Treatment of 51 with aqueous ammonia and then with DBU, followed by acidification using an ion-exchange resin, furnished the target neopterin glycoside (3b).
3-3. Synthesis of 6-hydroxymethylpterin α- and β-D-glucopyranosides (4b)
As shown in Figure 1, anomeric structures of some pterin glycosides are α-type and those of others are β-type depending on the combination of the pterin and sugar moieties. Therefore we undertook the exploration of an efficient protocol for selective α- and β-glycosilation of pterin derivatives by employing 6-hydroxymethylpterin (4a) as a model pterin substrate, leading to the selective synthesis of each of 6-hydroxymethylpterin α- and β-D-glucopyranoside (4b).43
6-Hydoxymethylpterin (4a),44 prepared from the pyrimidine derivative (14) and dihydroxyacetone, was converted into the N2-(N,N-dimethylaminomethylene)-3-NPE derivative (53) via 52 in four steps. (Scheme 10). Glycosylation of 53 with two glycosyl donors (9 and 34) in chloroform was then extensively investigated under various conditions in the presence of activators. Remarkable results are shown in Scheme 10.
Glycosylation of 53 with 9 in refluxing chloroform in the presence of silver triflate (2.0 mol equiv.) and TMU (1.0 mol equiv.) provided 6-[(β-D-glucopyranosyloxy)methyl]¬pterin derivative (54) in 63% yield, whereas the same reaction in the presence of tetraethylammonium bromide (2.0 mol equiv.) and N-ethyldiisopropylamine (2.0 mol equiv.) afforded α-D-gluco¬pyranose-1,2-orthobenzoate derivative (55) (in 93% yield) instead of the pterin glycoside.
Meanwhile glycosylation of 53 with 34 in refluxing chloroform in the presence of silver triflate and TMU resulted in the formation of decomposed compounds, but the same reaction in the presence of tetraethylammonium bromide and N-ethyldiisopropyl¬amine resulted in the formation of 6-[(α-D-glucopyranosyloxy)¬methyl]pterin derivative (56) as a sole product (69% yield).
Removal of the protecting groups of β- (54) and α-D-glucoside (56) was performed as usual, furnishing 6-hydroxymethylpterin β- (β-4b) and α-D-glucopyranoside (α-4b), respectively.
3-4. Synthesis of asperopterin-A (5b)
Synthetic methodology for pterin glycosides described so far was applied to preparation of asperopterin-A (5b) known as the sole example of a natural isoxanthopterin glycoside, as illustrated in Scheme 11.45
The 2-oxopropionate derivative (58) was prepared from ethyl acrylate in three steps. The pteridine ring formation of the pyrimidine derivative (57) with 58 and the subsequent introduction of N,N-dimethylaminomethylene group afforded the isoxanthopterin derivative (59) in 48% yield. Protection of 59 with NPE group and the following removal of TBS group provided 6-hydroxymethyl compound (60), which was then temporarily silylated to give the trimethylsilyl derivative (61).
Glycosylation of 61 with the glycosyl donor (11) was attempted in the presence of tin(IV) chloride (2.0 mol equiv.) to afford the D-ribofuranosyl derivative (62) in 43% yield, along with the recovery of 60 (45%). The β-anomeric configuration of the D-ribofuranoside (62) was assigned by its J1,2 value (0 Hz). Removal of the protecting groups of 62 was performed by the successive three-step-procedure, furnishing the target asperopterin-A (5b) in 81% overall yield.
4. CONCLUSION
We have developed a novel, effective way for selective preparation of both pterin 2’-O-β- and 2’-O-α-glycosides. As the representative examples of this work, the first synthesis of biopterin α-D-glucoside (1b) was achieved by use of the key intermediate 1’-O-PMB-biopterin derivative (13) and the novel glycosyl donor (34), while the biopterin β-D-glucoside (limipterin) (1d) was selectively prepared from 13 and the glycosyl donor (27). This synthetic strategy using N2-(N,N-dimethylaminomethylene)-3-NPE-protected pterin derivatives has proved a useful method applicable to preparation of various natural pterin glycosides having diverse pterin and sugar moieties.
The spectral data of the synthetic pterin glycosides were found to be essentially identical with those reported for the natural products and thus the validity of their proposed structures was confirmed. Although some ambiguous NMR parameters were included in the previous reports for natural compounds, their complete assignments have been established by this work.
References
1. (a) W. Pfleiderer, Angew. Chem., Int. Ed. Engl., 1964, 3, 114; CrossRef (b) H. Rembold and W. L. Gyure, Angew. Chem., Int. Ed. Engl., 1972, 11, 1061; CrossRef (c) T. Hama and T. Obika, Nature, 1960, 187, 326; CrossRef (d) H. S. Forrest and C. Van Baalen, Ann. Rev. Microbiol., 1970, 24, 91; CrossRef (e) I. Ziegler and R. Harmsen, Advan. Insect. Physiol., 1969, 6, 139.
2. H. S. Forrest, C. Van Baalen, and J. Myers, Arch. Biochem. Biophys., 1958, 78, 95. CrossRef
3. T. Matsunaga, J. G. Burgess, N. Yamada, K. Komatsu, S. Yoshida, and Y. Wachi, Appl. Microbiol. Biotechnol., 1993, 39, 250.
4. Y. K. Choi, Y. K. Hwang, Y. H. Kang, and Y. S. Park, Pteridines, 2001, 12, 121.
5. Y. Noguchi, A. Ishii, A. Matsushima, D. Haishi, K. Yasumuro, T. Moriguchi, T. Wada, Y. Kodera, M. Hiroto, H. Nishihara, M. Sekine, and Y. Inada, Mar. Biotechnol., 1999, 1, 207. CrossRef
6. T. Hanaya, K. Torigoe, K. Soranaka, H. Fujita, H. Yamamoto, and W. Pfleiderer, Pteridines, 2008, 19, 72.
7. K. W. Cha, W. Pfleiderer, and J. J. Yim, Helv. Chim. Acta, 1995, 78, 600. CrossRef
8. M. Ikawa, J. J. Sasner, J. F. Haney, and T. L. Foxall, Phytochemistry, 1995, 38, 1229. CrossRef
9. S.-H. Cho, J.-U. Na, H. Youn, C.-S. Hwang, C.-H. Lee, and S.-O. Kang, Biochim. Biophys. Acta, 1998, 1379, 53. CrossRef
10. (a) R. Suzuki and M. Goto, J. Biochem., 1968, 63, 798; (b) K. Kobayashi and H. S. Forrest, Comp. Biochem. Physiol., 1970, 33, 201. CrossRef
11. X. Lin and R. H. White, J. Bacteriol., 1988, 170, 1396.
12. D. L. Hatfield, C. van Baalen, and H. S. Forrest, Plant Physiol., 1961, 36, 240. CrossRef
13. H. W. Lee, C. H. Oh, A. Geyer, W. Pfleiderer, and Y. S. Park, Biochim. Biophys. Acta, 1999, 1410, 61. CrossRef
14. (a) Y. Kaneko and M. Sanada, J. Ferment. Technol., 1969, 47, 8; (b) S. Matsuura, M. Yamamoto, and Y. Kaneko, Bull. Chem. Soc. Jpn., 1972, 45, 492. CrossRef
15. (a) S. Kaufman and E. E. Kaufman, ‘Folates and Pterins,’ ed. by R. Blakley and S. J. Benkovic, J. Wiley & Sons, New York, 1985, Vol. 2, pp. 251–352; (b) S. Kaufman and D. B. Fisher, ‘Molecular Mechanisms of Oxygen Activation,’ ed. by O. Hayaishi, Academic Press, New York, 1974, pp. 285–369; (c) P. F. Fitzpatrick, Annu. Rev. Biochem., 1999, 68, 355. CrossRef
16. (a) N. S. Kwon, C. F. Nathan, and D. J. Stuehr, J. Biol. Chem., 1989, 264, 20496; (b) M. A. Marletta, Cell, 1994, 78, 927; CrossRef (c) B. R. Crane, A. S. Arvai, D. K. Ghosh, C. Q. Wu, E. D. Getzoff, D. J. Stuehr, and J. A. Tainer, Science, 1998, 5359, 2121. CrossRef
17. (a) H. Wachter, E. R. Werner, G. Reibnegger, D. Fuchs, and A. Hausen, Pteridines, 1989, 1, 3; (b) A. Hausen, G. Reibnegger, B. Speck, and H. Wachter, Transplantation, 1984, 38, 497; CrossRef (c) G. Hoffman, S. Kenn, B. Wirleitner, C. Deetjen, S. Frede, M. Smolny, J. Rieder, D. Fuchs, G. Baier-Bitterlich, and W. Schobersberger, Immunobiology, 1998, 199, 63. CrossRef
18. Y. Wachi, S. Yoshida, K. Komatsu, and T. Matsunaga, Jpn. Patent, 05,286,989, 1993 (Chem. Abstr., 1994, 120, 161782t).
19. T. Saito, H. Ishikawa, Y. Hada, K. Fukui, Y. Kodera, A. Matsushima, and Y. Inada, Dyes Pigments, 2003, 56, 203. CrossRef
20. W. Pfleiderer, ‘Physical Methods in Heterocyclic Chemistry’, Vol. 1, ed. by A. R. Katritzky, Academic Press, New York, 1963, pp. 177–188.
21. T. Hanaya, K. Torigoe, K. Soranaka, H. Yamamoto, Q. Yao, and W. Pfleiderer, Pteridines, 1995, 6, 1.
22. R. K. Ness, H. G. Fletcher, Jr., and C. S. Hudson, J. Am. Chem. Soc., 1950, 72, 2200. CrossRef
23. T. Hanaya, K. Soranaka, K. Harada, H. Yamaguchi, R. Suzuki, Y. Endo, H. Yamamoto, and W. Pfleiderer, Heterocycles, 2006, 67, 299. CrossRef
24. T. Hanaya, H. Toyota, and H. Yamamoto, Synlett, 2006, 2075. CrossRef
25. T. Hanaya, H. Baba, H. Toyota, and H. Yamamoto, Tetrahedron, 2008, 64, 2090. CrossRef
26. R. Gigg, S. Payne, and R. Conant, J. Carbohydr. Chem., 1983, 2, 207. CrossRef
27. L. Hough and T. J. Taylor, J. Chem. Soc., 1955, 3544. CrossRef
28. J. Kiss, R. D'Souza, and P. Taschner, Helv. Chim. Acta, 1975, 58, 311. CrossRef
29. (a) J. Weinstock, U.S. Patent, 3,505,329, 1970 (Chem. Abstr., 1970, 72, 132787h); (b) E. C. Taylor and P. A. Jacobi, J. Am. Chem. Soc., 1976, 98, 2301. CrossRef
30. (a) J. P. Geerts, A. Nagel, and H. C. Van der Plas, Org. Magn. Reson., 1976, 8, 607; CrossRef (b) T. Hanaya, D. Takayama, and H. Yamamoto, Heterocycles, 2006, 70, 355. CrossRef
31. (a) U. Ewers, H. Günther, and L. Jaenicke, Chem. Ber., 1974, 107, 3275; CrossRef (b) S. Tobias, H. Günther, and W. Pfleiderer, Chem. Ber., 1985, 118, 354. CrossRef
32. J. Farkas, M. Ledvina, J. Brokes, J. Jezek, J. Zajicek, and M. Zaoral, Carbohydr. Res., 1987, 163, 63. CrossRef
33. (a) S. Hanessian and J. Banoub, Carbohydr. Res., 1977, 53, C13; CrossRef (b) S. Hanessian and J. Banoub, Methods Carbohydr. Chem., 1980, 8, 247.
34. G. Wulff and G. Röhle, Angew. Chem., Int. Ed. Engl., 1974, 13, 157, and references cited therein. CrossRef
35. T. Hanaya, H. Baba, H. Toyota, and H. Yamamoto, Tetrahedron, 2009, 65, 7989. CrossRef
36. T. Hanaya, H. Baba, M. Kanemoto, and H. Yamamoto, Heterocycles, 2008, 76, 635. CrossRef
37. B. Hildebrandt, Y. Nakamura, and S. Ogawa, Carbohydr. Res., 1991, 214, 87. CrossRef
38. N. A. Jones, S. A. Nepogodiev, C. J. MacDonald, D. L. Hughes, and R. A. Field, J. Org. Chem., 2005, 70, 8556. CrossRef
39. (a) R. K. Ness, H. G. Fletcher, and C. S. Hudson, J. Am. Chem. Soc., 1950, 72, 2200; CrossRef (b) M. Mach, U. Schlueter, F. Mathew, B. Fraser-Reid, and K. C. Hazen, Tetrahedron, 2002, 58, 7345. CrossRef
40. R. Soyka and W. Pfleiderer, Helv. Chim. Acta, 1990, 73, 808. CrossRef
41. T. Hanaya, T. Hattori, D. Takayama, and H. Yamamoto, Pteridines, 2010, 21, 79.
42. W. W. Zorbach and G. D. Valiaveedan, J. Org. Chem., 1964, 29, 2462. CrossRef
43. T. Hanaya, H. Baba, K. Ejiri, and H. Yamamoto, Heterocycles, 2010, 80, 1013. CrossRef
44. C. M. Baugh and E. Shaw, J. Org. Chem., 1964, 29, 3610. CrossRef
45. T. Hanaya, K. Ejiri, and H. Yamamoto, Heterocycles, 2012, 84, 801. CrossRef