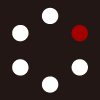
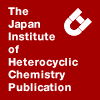
HETEROCYCLES
An International Journal for Reviews and Communications in Heterocyclic ChemistryWeb Edition ISSN: 1881-0942
Published online by The Japan Institute of Heterocyclic Chemistry
e-Journal
Full Text HTML
Received, 17th December, 2012, Accepted, 15th February, 2013, Published online, 21st February, 2013.
DOI: 10.3987/REV-12-762
■ Recent Progress in Organocatalytic Asymmetric Halocyclization
Kenichi Murai and Hiromichi Fujioka*
Graduate School of Pharmaceutical Science, Osaka University, 1-6 Yamadaoka, Suita, Osaka 560-0871, Japan
Abstract
In this review, recent progress made in the development of organocatalytic asymmetric halocyclization reactions that form heterocyclic compounds is described. New reactions and their mechanistic features are discussed in the context of an outline based on catalyst types, including biscinchona alkaloids, amino ureas, amino thioureas, amino thiocarbamates, chiral phosphoric acids, and trisimidazolines.CONTENTS
1. Introduction - - - - - - - - - - - - - - - - - - - - - - - - - - - - - - - - - - - - 1
2. Backgrounds - - - - - - - - - - - - - - - - - - - - - - - - - - - - - - - - - - - 5
3. Biscinchona alkaloid catalysts - - - - - - - - - - - - - - - - - - - - - - 8
4. Amino urea catalysts - - - - - - - - - - - - - - - - - - - - - - - - - - - - -15
5. Amino thiocarbamate catalysts - - - - - - - - - - - - - - - - - - - - - 19
6. Phosphoric acid catalysts - - - - - - - - - - - - - - - - - - - - - - - - - 23
7. Miscellaneous catalysts - - - - - - - - - - - - - - - - - - - - - - - - - - -28
8. Trisimidazoline catalyst - - - - - - - - - - - - - - - - - - - - - - - - - - - 32
9. Conclusion - - - - - - - - - - - - - - - - - - - - - - - - - - - - - - - - - - - - 39
References - - - - - - - - - - - - - - - - - - - - - - - - - - - - - - - - - - - - - - 40
1. INTRODUCTION
Halocyclization reactions of olefinic substrates, containing a pendant nucleophilic groups, serve as powerful methods in the synthesis of organic compounds.1 In particular, these processes can be employed to synthesize various simple and even complex heterocyclic compounds, including lactones, cyclic ethers, and cyclic amines. As well as their applicability to constructing heterocyclic ring systems, halocyclization reactions introduce halogen atoms into products, which is important because many halogen containing biologically active compounds are found in nature.2 In addition, from the viewpoint of synthetic utility, the halogen containing compounds generated in this manner are highly desirable as well-functionalized building blocks that can be utilized in further transformation. Another advantageous feature of halocyclization reactions is that they generally generate products with high degrees of diastereoselectively and in a highly predictable manner. Therefore, these processes have been widely used in routes for the synthesis of various natural products. In contrast, the development of enantioselective versions of halocyclization reactions has been taken place more slowly with advances being made only very recently.3
The mechanistic pathway for halocyclization reactions generally consists of an initial electrophilic addition of the halogen to olefin, forming a halonium ion intermediate, and subsequent anti-selective intramolecular cyclization through substitution by an internal nucleophile on carbon of the halonium ion. Based on this pathway, two approaches to the design of asymmetric halocyclization reactions have been considered. One involves the use of a halogen source that enables creation of a chiral environment for formation of the halonium ion intermediate from the olefin (Figure 1, (i)). In the development of this strategy, the most challenging and, in most cases, prohibiting task is to devise a method to generate the chiral halogenating agent in a catalytic manner. In addition, as recognized independently by Brown4 and Denmark,5 in the case of bromonium and iodonium ions, the propensity for degenerate halogen exchange reactions with olefins leads to undesirable racemization of the chiral halonium ion intermediates. The intervention of this process limits the degree of asymmetric induction in the halocyclization reaction.
A second approach involves creation of chiral environment for the nucleophilic substitution step in the pathway (Figure 1, (ii)). The guiding principle here is that a chiral nucleophile is expected to react selectively with one enantiomer of the halonium ion intermediate. The prospect of controlling the stereochemistry of the cyclization process by using chiral nucleophiles is supported by many successful examples of chiral auxiliary induced halocyclizations. Several examples of this phenomenon are found in halolactonization reactions using chiral amides (Scheme 1, (1)),6 as well as haloetherification reactions of chiral acetals (Scheme 1, (2)).7 Consequently, a great effort has been given recently to the development of the proper methods to bring about catalytic asymmetric nucleophile activation in asymmetric halocyclization reactions. In addition, dual activation approaches that include methods to activate both the halogen source and nucleophile are promising (Figure 1, (iii)), and some organocatalysts developed for promoting enantioselective halocyclization reactions are believed to behave in this manner.
In this review, recent investigations aimed at the development of organocatalytic asymmetric halocyclization reactions are described. In these efforts, various types of organocatalysts, including biscinchona alkaloids, amino ureas, amino thiocarbamates, S-alkyl thiocarbamates, chiral phosphoric acids, bifunctional and a trisimidazoline catalyst, have been shown to induce highly enantioselective processes (Figure 2). These processes along with their mechanistic features are discussed using a format, which classifies methods by catalyst type. Except for trisimidazoline catalysts, which have been developed recently in our laboratory, background information and characteristics of the organocatalysts are not presented because discussions of these topics can be found in earlier reviews.3 Electrophilic fluorination reactions of olefins likely are mechanistically different from other halogenations from the perspective of the intermediacy of halonium ion intermediates.8 Despite this potential difference, asymmetric versions of fluorocyclization reactions are covered in this review.
2. BACKGROUND
Before describing recent discoveries made in the field of catalytic asymmetric halocyclization reactions, we felt that it would be appropriate to summarize the results of important earlier work in this field. In 1992, Taguchi’s group described some appropriate bidentate ligands for carrying out asymmetric halocyclizations (Scheme 2, (i)).9 These workers found that in the presence of I2 and the chiral titanium complex generated from TADDOL derivative 5 and Ti(OiPr)4 diallyl-2-hydroxyacetic acid (6) as well as 2-hydroxymethylpent-4-en-1-ol (8) undergo respective desymmetrization type iodolactonization and iodoetherification reactions with moderate enantioselectivities (65% and 36% ee, respectively). Although a stoichiometric amount of the chiral ligand was necessary for these processes, the results of subsequent studies showed that only a catalytic amount of Ti(TADDOLate)2 (10) promotes iodocarbocyclization reaction of the 4-alkenylmalonate derivative 11 with excellent enantioselectivity (>95% ee) (Scheme 1, (ii)).10 This process was applied to a concise synthesis of the natural product, boschnialactone.
The observations summarized above show that a chiral environment for the nucleophile does indeed promote enantioselective halocyclization reactions. Although this technique is applicable to substrates that contain select types of functional groups like malonates, it is challenging to design catalysts that create chiral environments in halocyclization reactions of substrates containing more simple functional groups such as carboxylic acids and alcohols. For example, Gao’s group examined iodolactonization reactions of 5-aryl-4-pentanoic acids 13 using cinchona alkaloids as catalysts. These workers anticipated that chiral ion pairs would form between the carboxylic acid and catalyst and that the pairing would lead to stereochemical control. Although treatment of 13 with a stoichiometric amount of the catalyst led to formation of the cyclized products 14 and 15, the levels of enantioselectivity were not high (<35% ee).11 A two phase enantioselective iodolactonization reaction, using the chiral quaternary ammonium salts 16 derived from cinchonidine, was also described by the same group (Scheme 3).12 In this process, a chiral ammonium carboxylate ion pair with phase transfer capability might be formed in this reaction system. Although the first example of asymmetric iodolactonization using a catalytic amount (30 mol%) of a chiral catalyst was uncovered in this effort, the level of enantioselectivity control was not high (up to 42% ee).
In addition to employing chiral nucleophiles, chiral halogenating agents have been utilized to induce enantioselective halocyclization reactions. Stoichiometric amounts of various chiral amines have been used to prepare the chiral amine-halogen complexes. For example, O-benzoyl dihydroquinidine derivatives 17, 2-menthylpyridine (18), and N-methylephedrine (19) were employed to generate (amine)2X+Y- species (X = Br, I; Y = SbF6, BF4), and primary amines, such as 1-aminotetralin (20), were employed for preparation of ICl⋅amine complexes (Figure 3).13-15 Unfortunately, many studies aimed at using these chiral amine-halogen complexes for the halolactonization or haloetherification reactions failed to uncover acceptable levels of enantioselectivity. In only one case reported by Wirth’s group, involving iodolactonization reaction promoted by the chiral primary amine 20, was moderate selectivity achieved (up to 49% ee) (Scheme 4).15 These observations demonstrate the difficulty associated with the design of a proper chiral halogenation agent that brings about asymmetric halocyclization reactions.
In contrast, more success has been achieved with using metal catalysts to induce formation of chiral halogen species in enantioselective halocyclization reactions. For example, the first highly enantioselective iodoetherification reaction of γ-hydroxy-cis-alkenes 23 was developed by Kang’s groups in 2003.17 The process, promoted by using I2 in the presence of an unprecedented catalytic system generated from salen-Co(II) complex and N-chlorosuccinimide (NCS), produces 2-monosubstituted tetrahydrofurans 24 with high enantioselectivities (up to 90% ee). Although the first reactions explored required a relatively high loading of the salen-Co(II) complex 25a (30 mol%), improved conditions were found in which a lower loading of the salen-Cr(III)Cl complex 25b (7 mol%) promoted efficient and highly enantioselective reactions (Scheme 5). In 2009, Gao’s group demonstrated the utility of the reaction system comprised of salen-Co(II) 25a, NCS and I2 by its application to asymmetric iodolactonization reactions of various 4-pentenoic acid derivatives 26 that generate cyclized products 27 with high enantioselectivities (up to 83% ee) (Scheme 6).18
Along with metal catalysts, since 2007 organocatalysts have been used to generate chiral halogenating species and to induce high levels of enantioselectivity in halocyclization reactions. One example comes from studies by Ishihara’s group, which uncovered an enantioselective halopolycyclization of polyprenoid 28 (Scheme 7).19 In this process, the nucleophilic chiral phosphoramidite 30 and N-iodosuccinimide (NIS) are believed to form a tight ion pair. Unfortunately, a stoichiometric amount of 30 as the chiral promoter is required in this reaction system because the use of a catalytic amount (20 mol%) gave a poor enantioselectivity.
3. BISCINCHONA ALKALOID CATALYSTS
Beginning in 2010, intense research efforts led to the development of enantioselective halocyclization reactions that rely on the use of organocatalysts. Although earlier studies using cinchona alkaloid derivatives did not lead to the discovery of asymmetric halocyclization reactions that take place with sufficiently high levels of enentioselectivity, many efforts demonstrated that these alkaloids serve as privileged catalysts for a number of processes. The high efficiencies of reactions promoted by biscinchona alkaloids, such as (DHQD)2PHAL (31), (DHQ)2PHAL (32), (DHQD)2PYR (33), (DHQD)2AQN (34) and (DHQD)2PYDZ (35) (Figure 4), in contrast to those induced by their monomeric counterparts, has been shown in many investigations. The quinuclidine amine moiety in these catalysts is considered to play a significant role in governing catalytic activity. Because the DHQD and DHQ groups in the catalysts have a pseudo-enantiomeric relationship, reactions producing both enantiomers of products are possible, as shown (although not discussed) below.
Borhan’s group reported the first examples of catalytic enantioselective halolactonization reactions that take place with synthetically useful levels of enantioselectivity.20 For example, (DHQD)2PHAL (31) was shown to catalyze asymmetric chlorolactonization reactions of 4-substituted-4-pentenoic acids (36) that produce γ-lactones 37 (Scheme 8). The system in which 1,3-dichloro-5,5-diphenylhydantoin (DCDPH) is employed as the chlorine source and benzoic acid as a co-additive was found to promote the most efficient reactions using this catalyst. In addition, DCDPH, instead of the more common 1,3-dichloro-5,5-dimethylhydantoin (DCDMH), was utilized in this process because better results were obtained in scale-up reactions. Finally, a facile method for the preparation of DCDPH had been developed earlier by the same group.
Borhan and his coworkers utilized 1H NMR studies to elucidate the role played by two possible complexes formed between (DHQD)2PHAL (31) and DCDPH in governing asymmetric delivery of chlorine to the olefin in this reaction. The authors proposed that two types of association complexes can be formed in this process (Figure 5), one in which hydrogen bonding mediates association between the protonated catalyst and DCDPH, and another in which a tight ion pair is generated between the chlorinated catalyst and the anion of hydantoin. These complexes are suggested not only to control enantioselectivity but also to enhance the rate of the reaction.
The effect of the combinations of (DHQD)2PHAL (31) and chiral N-chlorohydantoins, such as the R and S enantiomers of 5-isopropyl-1,3-dichlorohydantoin (R-38 and S-38), was also probed in a subsequent study by Borhan’s group (Scheme 9).21 Interestingly, a clear matched/mismatched behavior was
observed in the chlorolactonization of 36c with (DHQD)2PHAL, where lactone 37c is produced in 78% yield with 83% ee when (R)-38 is employed while (S)-38 promotes a lower yielding and less enantioselective (44%, 69% ee) reaction. These results provide experimental support for the role played by a complex between the catalyst and the chlorine source.
The same group also reported that use of a combination of (DHQD)2PHAL (31) and DCDPH also promotes efficient, asymmetric chlorocyclization reactions of unsaturated amides 39 and 41.22 The respective oxazolines 40 and dihydrooxazines 42 are produced selectively in these processes in a manner that depends on the substitution pattern in the olefin. For example, 1,1-disubstituted olefin substrates 39 react to produce oxazolines 40 (Scheme 10, (1)) whereas trans-disubstituted and trisubstituted olefins 41 react to form dihydrooxazines 42 (Scheme 10, (2)). Olefins containing both aromatic and aliphatic olefin substituents participate in highly enantioselective reactions, whose products can be transformed to chiral 1,2- and 1,3-amino alcohols by utilizing simple procedures. It is interesting that, rather than the less polar CHCl3 and MeCN/CCl4, both polar and protic 2,2,2-trifluoroethanol is an optimal solvent for this process.
References
1. (a) M. D. Dowle and D. I. Davies, Chem. Soc. Rev., 1979, 8, 171; CrossRef (b) S. Ranganathan, K. M. Muraleedharan, N. K. Vaish, and N. Jayaraman, Tetrahedron, 2004, 60, 5273. CrossRef
2. For selected reviews, see: (a) C. Paul and G. Pohnert, Nat. Prod. Rep., 2011, 28, 186; CrossRef (b) C. Wagner, M. E. Omari, and G. M. König, J. Nat. Prod., 2009, 72, 540. CrossRef
3. For reviews, see: (a) G. Chen and S. Ma, Angew. Chem. Int. Ed., 2010, 49, 8306; CrossRef (b) A. Castellanos and S. P. Fletcher, Chem. Eur. J., 2011, 17, 5766; CrossRef (c) C. K. Tan, L. Zhou, and Y.-Y. Yeung, Synlett, 2011, 1335; CrossRef (d) S. A. Snyder, D. S. Treitler, and A. P. Brucks, Aldrichimica Acta, 2011, 44, 27; (e) U. Hennecke, Chem. Asian J., 2012, 7, 456; CrossRef (f) S. E. Denmark, W. E. Kuester, and M. T. Burk, Angew. Chem. Int. Ed., 2012, 51, 10938. CrossRef
4. (a) R. S. Brown, Acc. Chem. Res., 1997, 30, 131; CrossRef (b) A. A. Neverov and R. S. Brown, J. Org. Chem., 1996, 61, 962. CrossRef
5. S. E. Denmark, M. T. Burk, and A. J. Hoover, J. Am. Chem. Soc., 2010, 132, 1232. CrossRef
6. (a) T. Yokomatsu, H. Iwasawa, and S. Shibuya, J. Chem. Soc., Chem. Commun., 1992, 728; CrossRef (b) T. Yokomatsu, H. Iwasawa, and S. Shibuya, Tetrahedron Lett., 1992, 33, 6999. CrossRef
7. (a) H. Fujioka and Y. Kita, J. Synth. Org. Chem. Jpn., 2009, 67, 606; CrossRef (b) H. Fujioka, Synlett, 2012, 825. CrossRef
8. S. C. Wilkinson, R. Salmon, and V. Gouverneur, Future, Med. Chem., 2009, 1, 847. CrossRef
9. O. Kitagawa, T. Hanano, K. Tanabe, M. Shiro, and T. Taguchi, J. Chem. Soc., Chem. Commun., 1992, 1005. CrossRef
10. (a) T. Inoue, O. Kitagawa, A. Saito, and T. Taguchi, J. Org. Chem., 1997, 62, 7384; CrossRef (b) O. Kitagawa and T. Taguchi, Synlett, 1999, 1191. CrossRef
11. M. Wang, L. X. Gao, W. Yue, and W. P. Mai, Synth. Commun., 2004, 34, 1023. CrossRef
12. M. Wang, L. X. Gao, W. P. Mai, A. X. Xia, F. Wang, and S. B. Zhang, J. Org. Chem., 2004, 69, 2874. CrossRef
13. R. B. Grossman and R. J. Trupp, Can. J. Chem., 1998, 76, 1233. CrossRef
14. X.-L. Cui and R. S. Brown, J. Org. Chem., 2000, 65, 5653. CrossRef
15. (a) J. Haas, S. Piguel, and T. Wirth, Org. Lett., 2002, 4, 297; CrossRef (b) J. Haas, S. Bissmire, and T. Wirth, Chem. Eur. J., 2005, 11, 5777. CrossRef
16. J. M. Garnier, S. Robin, and G. Rousseau, Eur. J. Org. Chem., 2007, 3281. CrossRef
17. (a) S. H. Kang, S. B. Lee, and C. M. Park, J. Am. Chem. Soc., 2003, 125, 15748; CrossRef (b) S. H. Kang, S. Y. Kang, C. M. Park, H. Y. Kwon, and M. Kim, Pure Appl. Chem., 2005, 77, 1269; CrossRef (c) H. Y. Kwon, C. M. Park, S. B. Lee, J.-H. Youn, and S. H. Kang, Chem. Eur. J., 2008, 14, 1023. CrossRef
18. Z. Ning, R. Jin, J. Ding, and L. Gao, Synlett, 2009, 2291. CrossRef
19. A. Sakakura, A. Ukai, and K. Ishihara, Nature, 2007, 445, 900. CrossRef
20. D. C. Whitehead, R. Yousefi, A. Jaganathan, and B. Borhan, J. Am. Chem. Soc., 2010, 132, 3298. CrossRef
21. R. Yousefi, D. C. Whitehead, J. M. Mueller, R. J. Staples, and B. Borhan, Org. Lett., 2011, 13, 608. CrossRef
22. A. Jaganathan, A. Garzan, D. C. Whitehead, R. J. Staples, and B. Borhan, Angew. Chem. Int. Ed., 2011, 50, 2593. CrossRef
23. O. Lazano, G. Blessley, T. Martinez del Campo, A. L. Thompson, G. T. Giuffredi, M. Bettati, M. Walker, R. Borman, and V. Gouverneur, Angew. Chem. Int. Ed., 2011, 50, 8105. CrossRef
24. T. Ishimaru, N. Shibata, T. Horikawa, N. Yasuda, S. Nakamura, T. Toru, and M. Shiro, Angew. Chem. Int. Ed., 2008, 47, 4157. CrossRef
25. C. Baudequin, J.-F. Loubassou, J.-C. Plaquevent, and D. Cahard, J. Fluorine Chem., 2003, 122, 189. CrossRef
26. (a) Z.-M. Chen, Q.-W. Zhang, Z.-H. Chen, H. Li, Y.-Q. Tu, F.-M. Zhang, and J.-M. Tian, J. Am. Chem. Soc., 2011, 133, 8818; CrossRef (b) H. Li, F.-M. Zhang, Y.-Q. Tu, Q.-W. Zhang, Z.-M. Chen, Z.-M. Chen, and J. Li, Chem. Sci., 2011, 2, 1839. CrossRef
27. C. H. Müller, M. Wilking, A. Rühlmann, B. Wibbeling, and U. Hennecke, Synlett, 2011, 2043. CrossRef
28. M. R. Monaco and M. Bella, Angew. Chem. Int. Ed., 2011, 50, 11044. CrossRef
29. K. C. Nicolaou, N. L. Simmons, Y. Ying, P. M. Heretsch, and J. S. Chen, J. Am. Chem. Soc., 2011, 133, 8134. CrossRef
30. K. Ikeuchi, S. Ido, S. Yoshimura, T. Asakawa, M. Inai, Y. Hamashima, and T. Kan, Org. Lett., 2012, 14, 6016. CrossRef
31. M. Inai, T. Goto, T. Furuta, T. Wakimoto, and T. Kan, Tetrahedron: Asymmetry, 2008, 19, 2771. CrossRef
32. (a) W. Zhang, S. Zheng, N. Liu, J. B. Werness, I. A. Guzei, and W. Tang, J. Am. Chem. Soc., 2010, 132, 3664; CrossRef (b) W. Zhang, N. Liu, C. M. Schienebeck, K. Decloux, S. Zheng, J. B. Werness, and W. Tang, Chem. Eur. J., 2012, 18, 7296. CrossRef
33. W. Zhang, H. Xu, H. Xu, and W. Tang, J. Am. Chem. Soc., 2009, 131, 3832. CrossRef
34. G. E. Veitch and E. N. Jacobsen, Angew. Chem. Int. Ed., 2010, 49, 7332. CrossRef
35. A. G. Doyle and E. N. Jacobsen, Chem. Rev., 2007, 107, 5713. CrossRef
36. V. K. Chaikovskii, A. A. Funk, V. D. Filimonov, T. V. Petrenko, and T. S. Kets, Russ. J. Org. Chem., 2008, 44, 935. CrossRef
37. J. E. Tungen, J. M. J. Nolsøe, and T. V. Hansen, Org. Lett., 2012, 14, 5884. CrossRef
38. L. Zhou, C. K. Tan, X. Jiang, F. Chen, and Y.-Y. Yeung, J. Am. Chem. Soc., 2010, 132, 15474. CrossRef
39. (a) S. E. Denmark and W. R. Collins, Org. Lett., 2007, 9, 3801; CrossRef (b) S. E. Denmark and M. T. Burk, Proc. Natl, Sci. U.S.A., 2010, 107, 20655. CrossRef
40. (a) C. K. Tan, L. Zhou, and Y.-Y. Yeung, Org. Lett., 2011, 13, 2738; CrossRef (b) J. Chen, L. Zhou, C. K. Tan, and Y.-Y. Yeung, J. Org. Chem., 2012, 77, 999. CrossRef
41. C. K. Tan, C. Le, and Y.-Y. Yeung, Chem. Comm., 2012, 48, 5793. CrossRef
42. L. Zhou, J. Chen, C. K. Tan, and Y.-Y. Yeung, J. Am. Chem. Soc., 2011, 133, 9164. CrossRef
43. J. Chen, L. Zhou, and Y.-Y. Yeung, Org. Biomol. Chem., 2012, 10, 3808. CrossRef
44. X. Jiang, C. K. Tan, L. Zhou, and Y-Y. Yeung, Angew. Chem. Int. Ed., 2012, 51, 7771. CrossRef
45. U. Hennecke, C. H. Müller, and R. Fröhlich, Org. Lett., 2011, 13, 860. CrossRef
46. D. Huang, H. Wang, F. Xue, H. Guan, L. Li, X. Peng, and Y. Shi, Org. Lett., 2011, 13, 6350. CrossRef
47. S. E. Denmark and M. T. Burk, Org. Lett., 2012, 14, 256. CrossRef
48. V. Rauniyar, A. D. Lackner, G. L. Hamilton, and F. D. Toste, Science, 2011, 334, 1681. CrossRef
49. Y.-M. Wang, J. Wu, C. Hoong, V. Rauniyar, and F. D. Toste, J. Am. Chem. Soc., 2012, 134, 12928. CrossRef
50. G. Li, Q. Fu, X. Zhang, J. Jiang, and Z. Tang, Tetrahedron: Asymmetry, 2012, 23, 245. CrossRef
51. M. C. Dobish and J. N. Johnston, J. Am. Chem. Soc., 2012, 134, 6068. CrossRef
52. D. H. Paull, C. Fang, J. R. Donald, A. D. Pansick, and S. F. Martin, J. Am. Chem. Soc., 2012, 134, 11128. CrossRef
53. (a) H. Fujioka, K. Murai, Y. Ohba, A. Hiramatsu, and Y. Kita, Tetrahedron Lett., 2005, 46, 2197; CrossRef (b) H. Fujioka, K. Murai, O. Kubo, Y. Ohba, and Y. Kita, Tetrahedron, 2007, 63, 638; CrossRef (c) K. Murai, N. Takaichi, Y. Takahara, S. Fukushima, and H. Fujioka, Synthesis, 2010, 520; CrossRef (d) K. Murai, M. Morishita, R. Nakatani, H. Fujioka, and Y. Kita, Chem. Commun., 2008, 4498; CrossRef (e) H. Fujioka, K. Murai, Y. Ohba, H. Hirose, and Y. Kita, Chem. Commun., 2006, 832; CrossRef (f) K. Murai, M. Morishita, R. Nakatani, O. Kubo, H. Fujioka, and Y. Kita, J. Org. Chem., 2007, 72, 8947; CrossRef (g) K. Murai, S. Fukushima, S. Hayashi, Y. Takahara, and H. Fujioka, Org. Lett., 2010, 12, 964. CrossRef
54. (a) S. B. Tsogoeva, G. Dürner, M. Bolte, and M. W. Göbel, Eur. J. Org. Chem., 2003, 1661; CrossRef (b) D. Akalay, G. Dürner, J. W. Bats, M. Bolte, and M. W. Göbel, J. Org. Chem., 2007, 72, 5618. CrossRef
55. J. Xu, Y. Guan, S. Yang, Y. Ng, G. Peh, and C. H. Tan, Chem. Asian J., 2006, 1, 724. CrossRef
56. (a) C. Moberg, Angew. Chem. Int. Ed., 1998, 37, 248; CrossRef (b) C. Moberg, Angew. Chem. Int. Ed., 2006, 45, 4721; CrossRef (c) S. E. Gibson and M. P. Castaldi, Chem. Commun., 2006, 3045; CrossRef (d) S. E. Gibson and M. P. Castaldi, Angew. Chem. Int. Ed., 2006, 45, 4718. CrossRef
57. G. Desimoni, G. Faita, and K. A. Jørgensen, Chem. Rev., 2006, 106, 3561. CrossRef
58. K. Murai, S. Fukushima, S. Hayashi, Y. Takahara, and H. Fujioka, Org. Lett., 2010, 12, 964; CrossRef K. Murai, S. Fukushima, A. Nakamura, M. Shimura, and H. Fujioka, Tetrahedron, 2011, 67, 4862. CrossRef
59. (a) K. Murai, T. Matsushita, A. Nakamura, S. Fukushima, M. Shimura, and H. Fujioka, Angew. Chem. Int. Ed., 2010, 49, 9174; CrossRef (b) K. Murai, A. Nakamura, T. Matsushita, M. Shimura, and H. Fujioka, Chem. Eur. J., 2012, 18, 8448. CrossRef
60. (a) A. Kraft and R. Fröhlich, Chem. Commun., 1998, 1085; CrossRef (b) A. Kraft, A. Reichert, and R. Kleppinger, Chem. Commun., 2000, 1015. CrossRef
61. I. P. Singh, K. E. Milligan, and W. H. Gerwick, J. Nat. Prod., 1999, 62, 1333. CrossRef
62. For previous asymmetric total synthesis of tanikolide, see: (a) R. M. Kanada, T. Taniguchi, and K. Ogasawara, Synlett, 2000, 1019; CrossRef (b) H. Tanaka, Y. Kozuki, and K. Ogasawara, Tetrahedron Lett., 2002, 43, 4175; CrossRef (c) H. Mizutani, M. Watanabe, and T. Honda, Tetrahedron, 2002, 58, 8929; CrossRef (d) M. Carda, S. Rodriguez, E. Castillo, A. Bellido, S. D. Oltra, and J. A. Marco, Tetrahedron, 2003, 59, 857; CrossRef (e) A. E. Koumbis, K. M. Dieti, M. G. Vikentiou, and J. K. Gallos, Tetrahedron Lett., 2003, 44, 2513; CrossRef (f) J. M. Schomaker and B. Borhan, Org. Biomol. Chem., 2004, 2, 621; CrossRef (g) T. Ohgiya and S. Nishiyama, Tetrahedron Lett., 2004, 45, 8273; CrossRef (h) H. Arasaki, M. Iwata, M. Makida, and Y. Masaki, Chem. Pharm. Bull., 2004, 52, 848; CrossRef (i) Y. Kita, S. Matsuda, E. Fujii, M. Horai, K. Hata, and H. Fujioka, Angew. Chem. Int. Ed., 2005, 44, 5857; CrossRef (j) T. Ohgiya, K. Nakamura, and S. Nishiyama, Bull. Chem. Soc. Jpn., 2005, 78, 1549; CrossRef (k) F. Wu, R. Hong, J. Khan, X. Liu, and L. Deng, Angew. Chem. Int. Ed., 2006, 45, 4301; CrossRef (l) H. Fujioka, S. Matsuda, M. Horai, E. Fujii, M. Morishita, N. Nishiguchi, K. Hata, and Y. Kita, Chem. Eur. J., 2007, 13, 5238; CrossRef (m) P. Vichare and A. Chattopadhyay, Tetrahedron: Asymmetry, 2008, 19, 598; CrossRef (n) B. Gourdet and H. W. Lam, Angew. Chem. Int. Ed., 2010, 49, 8733; CrossRef (o) R. Doran, L. Duggan, S. Singh, C. D. Duffy, and P. J. Guiry, Eur. J. Org. Chem., 2011, 7097. CrossRef