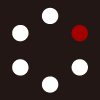
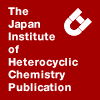
HETEROCYCLES
An International Journal for Reviews and Communications in Heterocyclic ChemistryWeb Edition ISSN: 1881-0942
Published online by The Japan Institute of Heterocyclic Chemistry
e-Journal
Full Text HTML
Received, 11th July, 2012, Accepted, 17th August, 2012, Published online, 23rd August, 2012.
DOI: 10.3987/COM-12-S(N)97
■ IMPROVEMENT OF HELIX-FORMING ABILITY OF MANNOSIDE-LINKED ETHYNYLPYRIDINE OLIGOMERS CONSTRUCTED BY CONVERGENT SYNTHESIS
Hajime Abe,* Hiroki Makida, and Masahiko Inouye*
Graduate School of Pharmaceutical Sciences, University of Toyama, Sugitani 2630, Toyama, 930-0194, Japan
Abstract
The improvement of helix-forming ability of α-D-mannoside-linked 2,6-pyridylene ethynylene "ethynylpyridine" oligomers was made by modification of the linker between the ethynylpyridine and mannoside moieties in the oligomers. The linker involves a triazole ring because the preparation utilizes Huisgen reaction, and the proper distance between the triazole ring and the ethynylpyridine moiety was found to be important to show strong Cotton effects.In the nature, helicity appears in the higher-order structures of biopolymers such as nucleic acids, peptides, and polysaccharides. The sophisticated functions of those biopolymers are based on their helical structures. Therefore in the field of biomimetic chemistry, it is one of the important issues to construct helices with synthetic materials at will.1
In this decade, our group has developed synthetic host compounds oligo- and poly(2,6-pyridylene ethynylene) 1, "ethynylpyridine oligomer/polymer", in which numbers of pyridine rings are tethered with acetylene bonds at their 2- and 6-positions (Figure 1).2,3,4 When these oligomers and polymers meet with a saccharide guest, they form helical complexes by multipoint hydrogen bonding. During the course of our study, we have designed saccharide-linked ethynylpyridine oligomers 2 that have a saccharide template at the one end. It was expected that they would form helices efficiently by intramolecular interaction between the template and the pyridine rings (Figure 2).2 As a result, the helix formation was well achieved, showing strong circular dichroism (CD), while it remained as a problem that the synthetic scheme was sequential and laborious, and lacked generality.
Therefore, we decided to develop convergent synthetic procedures to prepare a wide range of saccharide-linked ethynylpyridine oligomers more readily. Since our substrates are compatible with acetylene groups, Huisgen reaction was applied to attach saccharide azides to ethynylpyridine oligomers. In the previous report,3 oligomer 4 was developed (Figure 3), in which an acetylene group for Huisgen reaction was attached to an ethynylpyridine moiety with a hexynylene linker to avoid steric hindrance. Although 4 could be prepared straightforwardly from 3, the observed CD activity was rather weaker than that in the case of 2. It was supposed that the chiral effect of the saccharide template would be discounted by the distance of the linker.
Thus, we decided to develop a new type of saccharide-linked oligomers in which the oligomer and triazole moieties were directly tethered without a linker. Herein we report the helix-forming ability of saccharide-linked oligomers 6 as shown in Figure 4. The triazole ring in 6 might work as a member of supramolecular structures as reported by Hecht and coworkers.5
We prepared six kinds of α-D-mannoside-linked ethynylpyridine oligomers 6a, 6b, 6c, 11, 12, and 13 (Scheme 1), in which the triazole and the ethynylpyridine moieties are tethered directly as mentioned above (Figure 4). In 13 the α-mannosyl and the triazole moieties are also tethered directly, and in the other oligomers those two moieties via an ethylene group. The ethynylpyridine moieties of 6a, 11, 12, and 13 are 6-meric, and of 6b and 6c are 9-meric and 12-meric, respectively. The difference among the three 6-mers 6a, 11, and 12 is the kind of the substituent at the opposite end to the mannoside moiety. Diol 14 was prepared as a control oligomer without an α-mannoside moiety. Transition metal-assisted synthetic protocols such as Sonogashira coupling and Huisgen reaction were inevitable throughout the synthetic route to the target compounds.
The mannoside-linked ethynylpyridine oligomers 6a−c and 11 were prepared by Huisgen reaction of 2-azidoethyl α-D-mannopyranoside α-Man-N36 with ethynylpyridine blocks 9a, 9b, 9c, and 10 bearing two ethynyl groups at the both ends. The one end is protected with 1-hydroxy-1-methylethyl group, while the other is free, and Huisgen reaction took place with α-Man-N3 at the free end.7 The oligomeric blocks 9a, 9b, and 9c were derivatized from 7a, 7b, and 7c, respectively, as shown in Scheme 1. The preparations of 6-meric oligomers 7a, 8a, and 9a, and longer oligomers 7b and 7c were reported previously.3 The monoiodides 7a−c were coupled with (tert-butyldimethylsilyl)acetylene (TBSA) to give diprotected diynes 8a−c. The tert-butyldimethylsilyl (TBS) groups of 8a−c were treated with tetra-n-butylammonium fluoride (TBAF) to yield 9a−9c by protiodesilylation. Besides, the 1-hydroxy-1-methylethyl group of 8a was deprotected with NaOH to liberate acetone to give mono-TBS-protected diyne 10. The Huisgen reactions of α-Man-N3 with 9a−c and 10 were carried out by the use of CuSO4 pre-treated with sodium ascorbate and tris((1-benzyl-1H-1,2,3-
triazol-4-yl)methyl)amine8 (TBTA) to yield target compounds 6a−6c and 11, respectively.7 The use of TBTA somewhat improved the yields, compared to the case without TBTA.3 Furthermore, the TBS group of 11 was removed by TBAF to give 12. Similarly, the substrates 13 and 14 for reference experiments were obtained by Huisgen reaction of 9a with α-D-mannosyl azide9 and 2-azidoethanol,10 respectively.
The UV-vis and CD spectra of the α-D-mannoside-linked oligomers of various lengths 6a−c and the control 14 were studied in CH2Cl2 solutions (Figures 5A and 5B). The unit concentration of the pyridine rings of the oligomers was set at 5.0 × 10-4 M. In CD measurements, a positive CD band was observed around 330 nm in every case. This CD band indicates the formation of a chiral helical higher-order structure for the ethynylpyridine moiety. Typically, in our studies so far, various kinds of chiral helical ethynylpyridine oligomers exhibit a CD band of a similar shape around 330 nm.3,4 Of course, 6-mer 14 which lacks a saccharide moiety showed no meaningful CD activity. Among 6a−c, the order of the observed CDmax value around 330 nm was 6a > 6b > 6c. Because the pyridine unit concentrations were equal, that order reflected the order of the average CD strength of the pyridine rings in each substrate. Accordingly, that order could be explained by the distance between the mannoside template and the pyridine units, and the farther pyridine units in the longer oligomers were less affected by the template.
When the CD spectrum of 6a (Figure 6, blue line) was compared with that of the previous mannoside-linked ethynylpyridine 6-mer 4 (sugar = α-D-mannoside, n = 6, black line) composed by Huisgen reaction with a longer linker,3 the strength of the CD band of 6a were found to be much enhanced as expected. On contrary, 6-mer 13 (green line), in which the mannoside and ethynylpyridine moieties are tethered with merely one triazole ring, showed much weaker CD than 6a. For 13, flexibility of conformation would extremely be limited, so the interaction between ethynylpyridine and mannoside moieties would not work well. It was also found that the strength of the CD band around 330 nm for 6a−c was comparable to that for 2 (sugar = α-D-mannoside, m = 3, n = 6, orange line) having a flexible alkylene linker without a triazole ring.
The effect of substituent at the other end of the oligomer was studied by using 6a, 11, and 12. The CD and UV-vis spectra were shown in Figure 7, and the order of the strength of the first Cotton effect was 6a > 12 > 11, that is, the helix stabilization effect of the substituents was in the order of 1-hydroxy-1-methylethyl > H > TBS. The 1-hydroxy-1-methylethyl group in 6a would form a hydrogen bond with a pyridine nitrogen atom or a saccharide oxygen atom to stabilize the helix. Such a stabilization effect is supported by the molecular models obtained by Monte-Carlo analyses using a methoxy analogue of 6a (Figure 8).
The additive effect of MeOH was studied to estimate the contribution of hydrogen bonding for the helix formation. CD and UV-vis spectra of 6a were measured in a mixed solvent CH2Cl2/MeOH = 3:1 and compared to those measured in CH2Cl2. As shown in Figure 9 (blue solid and broken lines), the addition of MeOH suppressed the Cotton effect because MeOH disturbed the helix formation by the inhibition of the intramolecular hydrogen bonding.
Among UV-vis spectra shown in Figures 5B, 7B, and 9B, the differences were small. When the unit concentration of pyridine rings were set same, spectrum of 6a appeared to become weaker than those of 6b and 6c (Figure 5B). That is, absorption per one pyridine unit is weaker for 6a, probably because of hypochromism caused by π-interaction in the helix, corresponding to the stronger CD.
In summary, new α-D-mannoside-linked ethynylpyridine oligomers have been investigated to improve their chiral helical higher-order structure. Convergent syntheses involving Huisgen reaction yielded a variety of ethynylpyridine oligomers, with changing the lengths of oligomer and linker, and the kind of substituent at the end of the oligomer. As a result, the 6-mer 6a, of which ethynylpyridine and triazole moieties are tethered directly and the opposite end is protected by 1-hydroxy-1-methylethyl group showed an intense CD band around 330 nm. The CD intensity was much improved compared to our previous mannoside-linked oligomer which has a longer linker.
References
1. For recent reviews for chiral helical synthetic oligomers: (a) E. Yashima and K. Maeda, 'Foldamers', ed. by S. Hecht and I. Huc, Wiley-VCH, Weinheim, 2007, pp. 331−366; CrossRef (b) T. Sierra, 'Chirality at the Nanoscale', ed. by D. B. Amabilino, Wiley-VCH, Weinheim, 2009, pp. 115−189; CrossRef (c) E. Yashima, K. Maeda, H. Iida, Y. Furusho, and K. Nagai, Chem. Rev., 2009, 109, 6102; CrossRef (d) I. Saraogi and A. D. Hamilton, Chem. Soc. Rev., 2009, 38, 1726; CrossRef (e) E. Yashima, K. Maeda, and Y. Furusho, Acc. Chem. Res., 2008, 41, 1166; CrossRef (f) E. Yashima and K. Maeda, Macromolecules, 2008, 41, 3; CrossRef (g) D. J. Hill, M. J. Mio, R. B. Prince, T. S. Hughes, and J. S. Moore, Chem. Rev., 2001, 101, 3893; CrossRef (h) T. Nakano and Y. Okamoto, Chem. Rev., 2001, 101, 4013. For the molecular recognition functions of synthetic helical foldamers, see also references in ref. 3. CrossRef
2. H. Abe, D. Murayama, F. Kayamori, and M. Inouye, Macromolecules, 2008, 41, 6903. CrossRef
3. H. Abe, H. Makida, and M. Inouye, Tetrahedron, 2012, 68, 4353. CrossRef
4. (a) H. Abe, K. Okada, H. Makida, and M. Inouye, Org. Bioorg. Chem., 2012, 10, 6930; CrossRef (b) S. Takashima, H. Abe, and M. Inouye, Chem. Commun., 2012, 48, 3330; CrossRef (c) S. Takashima, T. Yamamoto, H. Abe, and M. Inouye, Heterocycles, 2012, 84, 355; CrossRef (d) S. Takashima, H. Abe, and M. Inouye, Chem. Commun., 2011, 47, 7455; CrossRef (e) H. Abe, S. Takashima, T. Yamamoto, and M. Inouye, Chem. Commun., 2009, 2121; CrossRef (f) H. Abe, H. Machiguchi, S. Matsumoto, and M. Inouye, J. Org. Chem., 2008, 73, 4650; CrossRef (g) M. Waki, H. Abe, and M. Inouye, Angew. Chem. Int. Ed., 2007, 46, 3059; CrossRef (h) M. Waki, H. Abe, and M. Inouye, Chem. Eur. J., 2006, 12, 7639; CrossRef (i) H. Abe, N. Masuda, M. Waki, and M. Inouye, J. Am. Chem. Soc., 2005, 127, 16189; CrossRef (j) M. Inouye, M. Waki, and H. Abe, J. Am. Chem. Soc., 2004, 126, 2022. CrossRef
5. (a) T. E. Malah, A. Ciesielski, L. Piot, S. I. Troyanov, U. Mueller, S. Weidner, P. Samorì, and S. Hecht, Nanoscale, 2012, 4, 467; CrossRef (b) D. Zornik, R. M. Meudtner, T. E. Malah, C. M. Thiele, and S. Hecht, Chem. Eur. J., 2011, 17, 1473; CrossRef (c) R. M. Meudtner and S. Hecht, Angew. Chem. Int. Ed., 2008, 47, 4926. CrossRef
6. S. Park and I. Shin, Org. Lett., 2007, 9, 1675. CrossRef
7. Preparation of 6a; A typical procedure for Huisgen reaction. To a mixture of 9a (30.4 mg, 20.8 μmol), 2-azidoethyl α-D-mannopyranoside (α-Man-N3, 11.2 mg, 44.9 μmol), sodium ascorbate (2.7 mg, 13.5 μmol), TBTA (2.4 mg, 4.5 μmol), CH2Cl2, (1 mL), and t-BuOH (1 mL) was added a water (1 mL) solution of CuSO4 (0.71 mg, 4.5 μmol). After the mixture was stirred for 22.5 h at room temperature, additional α-Man-N3 (11.2 mg, 44.9 μmol) was added to the mixture. The reaction mixture was stirred additionally for 5.5 h, and then a water (0.3 mL) solution of CuSO4 (0.71 mg, 4.5 μmol), sodium ascorbate (2.7 mg, 13.5 μmol), and TBTA (2.4 mg, 4.5 μmol) was added to the mixture. The resulting reaction mixture was stirred for 15 h and concentrated by a rotary evaporator, and the resulting residue was subjected to silica gel column chromatography (eluent: CHCl3 to CHCl3/MeOH = 20:1) to afford 6a (28.9 mg, 81% based on 9a) as a pale brown solid. mp 90−95 °C; νmax (KBr) 3353, 2925, 2855, 2354, 1584, 1548 cm-1; 1H NMR (300 MHz, CDCl3) δ 8.56 (s, 1 H), 7.60 (d, J = 2.4 Hz, 1 H), 7.16−6.91 (m, 11 H), 4.96 (s, 1 H), 4.83 (br s, 1 H), 4.72−4.46 (br m, 3 H), 4.14−3.90 (m, 14 H), 3.88−3.71 (br m, 3 H), 3.60−3.48 (br, 2 H), 1.90−1.70 (m, 12 H), 1.61 (s, 6 H), 1.52−1.12 (m, 60 H), 0.96−0.80 (m, 18 H); δC (75 MHz, CDCl3) 165.44, 165.27, 143.80, 143.64, 114.41, 114.45, 87.60, 87.43, 87.09, 77.24, 68.88, 65.33, 31.89, 31.23, 29.30, 28.98, 28.85, 25.95, 22.78, 14.24; HRMS (ESI) Calcd for C103H137N9O13 (M + Na+): 1732.0266; Found: 1732.0260.
8. (a) J. E. Hein, L. B. Krasnova, M. Iwasaki, and V. V. Fokin, Org. Synth., 2011, 88, 238; (b) T. R. Chan, R. Hilgraf, K. B. Sharpless, and V. V. Fokin, Org. Lett., 2004, 6, 2853. CrossRef
9. G. J. Clarkson and D. M. Haddleton, QSAR Comb. Sci., 2007, 26, 1220. CrossRef
10. M. Dowlut, D. G. Hall, and O. Hindsgaul, J. Org. Chem., 2005, 70, 9809. CrossRef