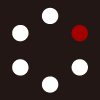
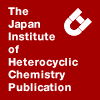
HETEROCYCLES
An International Journal for Reviews and Communications in Heterocyclic ChemistryWeb Edition ISSN: 1881-0942
Published online by The Japan Institute of Heterocyclic Chemistry
e-Journal
Full Text HTML
Received, 29th June, 2012, Accepted, 20th July, 2012, Published online, 24th July, 2012.
DOI: 10.3987/COM-12-S(N)76
■ Selective Introduction of Four Contiguous Stereocenters on the B-Ring of 4-Hydroxyzinowol
Masafumi Iwatsu, Daisuke Urabe, Hidenori Todoroki, Kengo Masuda, and Masayuki Inoue*
Graduate School of Pharmaceutical Sciences, University of Tokyo, 7-3-1 Hongo, Bunkyo-ku, Tokyo 113-0033, Japan
Abstract
4-Hydoxyzinowol is a bioactive polyoxygenated dihydro-β-agarofuran sesquiterpenoid. Here we describe construction of four contiguous cis-oriented stereocenters on the B-ring of 4-hydroxyzinowol. Introduction of a C7-isopropenyl group by the 1,4-addition of isopropenyl magnesium bromide was effectively assisted by methyl aluminum bis(2,6-di-tert-butyl-4-methylphenoxide). Taking advantage of the presence of the C7-substituent, three hydroxy groups were installed in a stereoselective fashion at the C6, 8 and 9 positions. In this study, we employed a new reagent combination of Sc(OTf)3 and Zn(OTf)2 for the hydrolysis of the cyclic acetal on the rigid oxabicyclo[3.2.1]octane structure.4-Hydoxyzinowol (1), isolated from Zinowiewia costaricensis, is a polyoxygenated dihydro-β-agarofuran sesquiterpenoid.1,2 The compound shows a reversal effect against P-glycoprotein-overexpressing multi-drug resistant (MDR) cells, and thus it is expected to be used in the treatment of MDR cancer. The core structure of 1 is composed of trans-decaline (AB-ring) attached by a tetrahydrofuran moiety (C-ring), and six acyloxy and one hydroxy groups densely decorate the AB-ring. Because of its highly oxygenated tricyclic structure, 1 poses a formidable synthetic challenge.3 As an initial phase of our synthetic study on 1, we decided to develop an efficient route to the functionalized B-ring structure. Here we report the stereoselective synthesis of compound 2 bearing four contiguous cis-oriented stereocenters on the B-ring (Scheme 1). Compound 2 would serve as an advanced intermediate for construction of the entire structure of 1.
The retrosynthesis of 2 is illustrated in Scheme 1. Compound 2 was envisioned to be prepared from 3 through C8-hydroxylation and stepwise reduction of C6- and C9-ketones. Stereochemistries at the C6, 8 and 9 positions would be established by taking advantage of the presence of the C7-substituent. Specifically, the bulky C7 three-carbon unit was expected to exert a conformational or steric bias for controlling the face-selective reactions on the B-ring. Installation of the C7-substituent of 3 would in turn be achieved by 1,4-addition of the isopropenyl group to the naphthoquinone monoketal 4. The 1,4-acceptor 4 was then to be prepared from the known naphthalene derivative 54 via chemoselective oxidative dearomatization.
The synthesis started with the oxidative dearomatization of 5 using PhI(OCOCF3)2 in a 1 : 4 mixture of CH3CN and ethylene glycol, leading to the naphthoquinone monoketal 6 (Scheme 2).5 After the protecting group of the phenolic hydroxy group of 6 was changed from Ac to MOM using the standard deprotection/protection procedure, 1,4-addition of the isopropenyl group was investigated. The copper-promoted addition of the isopropenyl group to 4 provided 3 in low yield, due to reductive formation of dihydroquinone from the quinone monoketal 4. On the other hand, methyl aluminum bis(2,6-di-tert-butyl-4-methylphenoxide) (MAD) smoothly enabled conjugate addition of isopropenyl magnesium bromide to 4, providing 3 in 96% yield.6 Then, the isopropenyl group of 3 was epoxidized by treating with m-CPBA to afford 8 as the C11 epimeric mixture (dr = 2.7 : 1).
Next, introduction of the C8S*-hydroxy group was explored (Scheme 3). The typical protocols for the α-hydroxylation of the ketone (e.g. TMS enol ether formation and subsequent m-CPBA epoxidation) proceeded exclusively from the opposite face to the bulky C7-substituent, generating the undesired C8R*-hydroxy group. Thus, an in situ inversion process was investigated to attain C8S*-hydroxylation from the sterically hindered side. After many unsuccessful experiments, it was found that subjection of 8 to PhI(OAc)2 and KOt-Bu in MeOH7 afforded the desired C8S*-isomer 12 in 60% yield.8
The inversion process is rationalized as the following (Scheme 3). The present hydroxylation of 8 is initiated by enolization of the C9-ketone by KOt-Bu, and the following anti-selective C8R*-iodination generates 9. Potassium methoxide attacks the C9-ketone of 9 and subsequent SN2 displacement of the iodine atom with the resultant C9-alkoxide inverts the C8-stereocenter of 10 to generate 11. Methanolysis of the unstable epoxide 11 gives the requisite 12.
Having constructed the C8S*-hydroxy group, the C6-hydroxy group was installed through stereoselective reduction (Scheme 4). Before doing so, the epoxide of 12 was reduced by LiAlH4 to form the C11-tertiary alcohol 13. The C6-ketone 15, the reduction substrate, was then constructed through a two-step process. Treatment of 13 under the anhydrous protic conditions promoted intramolecular acetal formation between the C11-hydroxy group and the C9-dimethyl acetal to afford 14, and simultaneous removal of the cyclic acetal and the MOM groups of 14 under the aqueous protic conditions produced 15.9 Reduction of the C6-ketone of the oxabicyclo[3.2.1]octane structure 15 with NaBH4 proceeded from the convex face, leading to the C6-alcohol 16 as a single product. The NOE correlation between H6 and H8, and the coupling constant between H7 and H8 (J = 0 Hz) determined the relative stereochemistry at the C6, 7 and 8 positions of 16.
To synthesize the requisite C9-alcohol of 2 from 16, hydrolysis of the acetal was required to liberate the C9-ketone (Table 1). Although the strong Brønsted acid, H2SO4, was effective for the hydrolysis of 16, the tetrahydroxylated ketone 17 decomposed under the reaction conditions (entry 1). After an extensive screening of Lewis acids, it was found that Sc(OTf)3 converted the rigid acetal 16 to ketone 17 in higher yield (entry 2). Although the lower catalyst loading of Sc(OTf)3 decreased the yield of 17 (entry 3), the reagent combination of a catalytic Sc(OTf)3 and a stoichiometric Zn(OTf)2 allowed the high-yielding formation of 17 (97% yield, entry 4). To clarify the role of Zn(OTf)2, 16 was treated only with Zn(OTf)2 (entry 5). However, these conditions resulted in no transformation, and the clean recovery indicated that Zn(OTf)2 functioned as the assisting reagent for the Sc(OTf)3-catalyzed reaction.10 LiOTf was less effective to increase the yield, suggesting that the multivalent nature of Zn(OTf)2 was important for acetal cleavage (entry 6).
From these experiments, the mechanism of the hydrolysis is proposed in Scheme 5. Strongly Lewis-acidic Sc(OTf)3 initially cleaves the acetal of 16 to afford the Sc3+-complex 18, which is stabilized by the three chelating hydroxy groups. Sc(OTf)3 in the complex 18 is exchanged by multivalent and more concentrated Zn(OTf)2, regenerating the Sc(OTf)3 catalysis.11,12 Finally, the aqueous workup converts the Zn2+-complex 19 to 17. In this proposed mechanism, the acid-labile 17 is not exposed in the reaction mixture, and thus its facile decomposition is prevented.
The targeted compound 2 was synthesized from 17 in two steps (Scheme 6). The phenolic hydroxy group of tetraol 17 was chemoselectively protected as the TIPS ether. The reduction of the C9-ketone of 20 with NaBH4 proceeded selectively from the less hindered face of the B-ring to produce 213 (dr = 13:1). The NOE correlation between H7 and H9 confirmed that all the three hydroxy groups at the C6, 8 and 9 positions, and the substituent at the C7 position in 2 were cis-oriented.
In summary, introduction of four contiguous stereocenters at the C6, 7, 8 and 9 positions on the B-ring of 4-hydroxyzinowol was achieved from the naphthalene derivative in 13 steps. The C7-isopropenyl group was installed on the naphthoquinone derivative via MAD-assisted 1,4-addition. The hydroxy groups at the C6, 8 and 9 positions were introduced by taking advantage of the presence of the C7-substituent. Specifically, C8-hydroxylation proceeded from the face hindered by the C7-substituent by employing PhI(OAc)2 and KO-tBu in MeOH. Reduction from the convex face of the oxabicyclo[3.2.1]octane compound, which was constructed through intramolecular acetal formation, set the requisite C6-stereocenter. The C9-hydroxy group was then generated by stereoselective reduction of the C9-ketone from the less hindered side of the B-ring, affording 2 with the cis-oriented C6, 7, 8 and 9-substituents. In addition, the new reagent combination of Sc(OTf)3 and Zn(OTf)2 was established for the hydrolysis of the acetal on the rigid oxabicyclo[3.2.1]octane structure. Further study toward the total synthesis of 4-hydroxyzinowol from 2 is currently underway in our laboratory.
ACKNOWLEDGEMENTS
This research was financially supported by the Funding Program for Next Generation World-Leading Researchers (JSPS) to M.In. and a Grant-in-Aid for Young Scientists (B) (JSPS) to D.U. Fellowships to M.Iw. and H.T. from JSPS are gratefully acknowledged.
References
1. For reviews on dihydro-β-agarofuran sesquiterpenoids, see: A. C. Spivey, M. Weston, and S. Woodhead, Chem. Soc. Rev., 2002, 31, 43; CrossRef J.-M. Gao, W.-J. Wu, J.-W. Zhang, and Y. Konishi, Nat. Prod. Rep., 2007, 24, 1153. CrossRef
2. F. Muñoz-Martínez, C. R. Mendoza, I. L. Bazzocchi, S. Castanys, I. A. Jiménez, and F. Gamarro, J. Med. Chem., 2005, 48, 4266. CrossRef
3. For a total synthesis of polyoxygenated dihydro-β-agarofuran sesquiterpenoid, see: J. D. White, N. S. Cutshall, T.-S. Kim, and H. Shin, J. Am. Chem. Soc., 1995, 117, 9780; CrossRef J. D. White, H. Shin, T.-S. Kim, and N. S. Cutshall, J. Am. Chem. Soc., 1997, 119, 2404; CrossRef For synthetic studies on related compounds, see: H. C. Barrett and G. Büchi, J. Am. Chem. Soc., 1967, 89, 5665; CrossRef A. Asselin, M. Mongrain, and P. Deslongchamps, Can. J. Chem., 1968, 46, 2817; CrossRef J. A. Marshall and M. T. Pike, J. Org. Chem., 1968, 33, 435; CrossRef C. H. Heathcock and T. R. Kelly, Chem. Commun., 1968, 267; CrossRef G. Büchi and H. Wüest, J. Org. Chem., 1979, 44, 546; CrossRef J. W. Huffman and P. C. Raveendranath, Tetrahedron, 1987, 43, 5557; CrossRef A. C. Spivey, S. J. Woodhead, M. Weston, and B. I. Andrews, Angew. Chem., Int. Ed., 2001, 40, 769; CrossRef W.-D. Z. Li, G. Zhou, X. Gao, and Y. Li, Tetrahedron Lett., 2001, 42, 4649; CrossRef G. Mehta and R. S. Kumaran, Tetrahedron Lett., 2003, 44, 7055; CrossRef F.-D. Boyer, T. Prangé, and P.-H. Ducrot, Tetrahedron: Asymmetry, 2003, 14, 1153; CrossRef A. Siwicka, D. Cuperly, L. Tedeschi, R. L. Vézouët, A. J. P. White, and A. G. M. Barrett, Tetrahedron, 2007, 63, 5903; CrossRef L. Jiao, M. Lin, L.-G. Zhuo, and Z.-X. Yu, Org. Lett., 2010, 12, 2528. CrossRef
4. J. Becher, O. A. Matthews, M. B. Nielsen, F. M. Raymo, and J. F. Stoddart, Synlett, 1999, 330. CrossRef
5. Y. Tamura, T. Yakura, J. Haruta, and Y. Kita, J. Org. Chem., 1987, 52, 3927. CrossRef
6. K. Maruoka, K. Nonoshita, and H. Yamamoto, Tetrahedron Lett., 1987, 28, 5723; CrossRef K. Maruoka, T. Itoh, M. Sakurai, K. Nonoshita, and H. Yamamoto, J. Am. Chem. Soc., 1988, 110, 3588; CrossRef A. J. Stern, J. J. Rohde, and J. S. Swenton, J. Org. Chem., 1989, 54, 4413. CrossRef
7. R. M. Moriarty, H. Hu, and S. C. Gupta, Tetrahedron Lett., 1981, 22, 1283; CrossRef R. M. Moriarty, O. Prakash, and W. A. Freeman, J. Chem. Soc., Chem. Commun., 1984, 927. CrossRef
8. Although the C11-stereochemistry of each stereoisomer of 8 was not determined, the separate experiments using major isomer 8A and minor isomer 8B revealed their different reactivities. The C8-hydroxylation of 8A provided 12A in 68% yield, while 8B was transformed to 12B in 49% yield under the same conditions. Therefore, the ratio of the C11-epimeric isomers was changed from 2.7 : 1 to 4.4 : 1 after the reaction of 8 in Scheme 3. CrossRef
9. Single-step conversion of 13 to 15 with aqueous 2 M HCl in THF decreased the yield of 15 (39% yield).
10. For selected reactions promoted by the Lewis acid/Lewis acid combination, see: N. Iwasawa and T. Mukaiyama, Chem. Lett., 1987, 463; CrossRef Y. Ukaji, N. Koumoto, and T. Fujisawa, Chem. Lett., 1989, 1623; CrossRef K. C. Nicolaou, C.-K. Hwang, and M. E. Duggan, J. Am. Chem. Soc., 1989, 111, 6682; CrossRef C. Y. Hong and Y. Kishi, J. Am. Chem. Soc., 1991, 113, 9693; CrossRef K. Higashi, K. Nakayama, E. Shioya, and T. Kusama, Chem. Pharm. Bull., 1991, 39, 2502; CrossRef K. Higashi and H. Susaki, Chem. Pharm. Bull., 1992, 40, 2019; CrossRef E. L. Myers, C. P. Butts, and V. K. Aggarwal, Chem. Commun., 2006, 4434; CrossRef S. Kamogawa, T. Ikeda, M. Kuriyama, Y. Matsumura, and O. Onomura, Heterocycles, 2010, 82, 325. CrossRef
11. The multiple chelating nature of Zn2+ would be necessary to replace Sc(OTf)3 from complex 18. For the coordination chemistry of Zn2+, see: C. W. Bock, A. K. Katz, and J. P. Glusker, J. Am. Chem. Soc., 1995, 117, 3754. CrossRef
12. Relatively weak Lewis acidity of Zn(OTf)2 compared to Sc(OTf)3 is attributable to the high yielding formation of 17. For papers on Sc(OTf)3-catalyzed acetal formation, see: S. Fukuzawa, T. Tsuchimoto, T. Hotaka, and T. Hiyama, Synlett, 1995, 1077; CrossRef K. Ishihara, Y. Karumi, M. Kubota, and H. Yamamoto, Synlett, 1996, 839; CrossRef M. Inoue, M. Sasaki, and K. Tachibana, J. Org. Chem., 1999, 64, 9416; CrossRef For papers on Sc(OTf)3-catalyzed acetal cleavage for C-glycosylations, see; T. Yamanoi and I. Yamazaki, Tetrahedron Lett., 2001, 42, 4009; CrossRef A. Ben, T. Yamauchi, T. Matsumoto, and K. Suzuki, Synlett, 2004, 225; CrossRef S. Sato, T. Akiya, T. Suzuki, and J. Onodera, Carbohydr. Res., 2004, 339, 2611; CrossRef T. Yamauchi, M. Shigeta, T. Matsumoto, and K. Suzuki, Heterocycles, 2005, 66, 153; CrossRef For reviews on Sc(OTf)3, see: S. Kobayashi, Eur. J. Org. Chem., 1999, 15; CrossRef S. Kobayashi, M. Sugiura, H. Kitagawa, and W. W.-L. Lam, Chem. Rev., 2002, 102, 2227. CrossRef
13. Physical data for 2: colorless oil; IR (neat) νmax 3390, 2944, 2867, 1585, 1467, 1280, 1036 cm-1; 1H NMR (400 MHz, CDCl3) δ 1.11 (9H, d, J = 7.8 Hz, CH3 of TIPS x 3), 1.14 (9H, d, J = 7.8 Hz, CH3 of TIPS x 3), 1.36 (3H, m, CH of TIPS), 1.46 (s, 3H, CH3), 1.58 (s, 3H, CH3), 1.61 (1H, d, J = 4.1 Hz, CHC(OH)(CH3)2), 2.99 (1H, d, J = 11.2 Hz, C=CCH(OH)CHOH), 3.34 (1H, d, J = 4.1 Hz, C=CCH(OH)CHC(OH)(CH3)2), 3.82 (1H, s, C(OH)(CH3)2), 3.97 (1H, d, J = 3.7 Hz, C=CCH(OH)CHOH), 4.50 (1H, dd, J = 11.2, 3.7 Hz, C=CCH(OH)CHOH), 4.70 (1H, dd, J = 3.7, 3.7 Hz, C=CCH(OH)CHOH), 5.53 (1H, dd, J = 4.1, 4.1 Hz, C=CCH(OH)CHC(OH)(CH3)2), 6.79 (1H, d, J = 8.0 Hz, CH=COTIPS), 7.25 (1H, dd, J = 8.0, 8.0 Hz, CH=CH-CH), 7.35 (1H, d, J = 8.0 Hz, C-CH=CH); 13C NMR (125 MHz, CDCl3) δ 12.9, 18.00, 18.03, 28.3, 28.7, 46.9, 65.1, 68.7, 71.4, 74.2, 116.8, 120.3, 126.9, 129.4, 138.1, 153.4; HRMS (ESI), calcd for C22H38O5SiNa 433.2386 (M+Na+), found 433.2393.