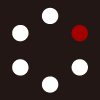
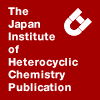
HETEROCYCLES
An International Journal for Reviews and Communications in Heterocyclic ChemistryWeb Edition ISSN: 1881-0942
Published online by The Japan Institute of Heterocyclic Chemistry
e-Journal
Full Text HTML
Received, 5th October, 2012, Accepted, 12th November, 2012, Published online, 22nd November, 2012.
■ ASYMMETRIC INTRAMOLECULAR CONJUGATE ADDITION OF α-AMINO ACID DERIVATIVES VIA RACEMIZATION-FREE EQUILIBRIUM OF INTERMEDIARY CHIRAL ENOLATES
Daiki Monguchi, Tomoyuki Yoshimura, Kazuyuki Irie, Kazuhiro Hayashi, Swapan Majumdar, Takahiro Sasamori, Norihiro Tokitoh, and Takeo Kawabata*
Institute for Chemical Research, Kyoto University, Uji, Kyoto 611-0011, Japan
Abstract
A highly enantioselective route to a multi-substituted indoline derivative via intramolecular conjugate addition of an α-amino acid derivative has been developed. Enantioselectivity of the reaction was almost independent from the temperature, while the diastereoselectivity was totally dependent on the temperature. High diastereomeric ratios observed at the higher temperatures were assumed to be the results from an equilibrium process between axially chiral enolate intermediates.INTRODUCTION
Asymmetric construction of a tetrasubstituted carbon center is still one of the challenging tasks in synthetic organic chemistry. We have developed a method for the enantioselective construction of contiguous tetra- and trisubstituted stereocenters by intramolecular conjugate addition of enolates derived from α-amino acid derivatives via memory of chirality.1-3 This method enables to produce multi-substituted nitrogen heterocycles in a highly enantioselective manner. We sought to apply this method to enantioselective synthesis of multi-substituted indoline derivatives because the indoline skeleton frequently appears in biologically active natural and unnatural compounds.4 Here we report highly enantioselective synthesis of a multi-substituted indoline derivative via intramolecular conjugate addition of enolates derived from an α-amino acid derivative. The enantioselectivity of the reactions was found to be almost independent from the reaction temperature, while the diastereoselectivity was highly dependent on the reaction temperature. The diastereoselectivity increased at the higher temperaturewithout significant loss of enantioselectivity. The thermodynamic equilibrium between chiral enolate intermediates has been proposed for this phenomenon.
RESULTS AND DISCUSSION
Precursor 1 for the indoline synthesis was readily prepared from L-valine as shown in Scheme 1. Compound 1-I was obtained by Cu-catalyzed N-arylation of L-valine according to the Ma’s protocol in 86% yield.5 Transformation of 1-I into 1 was performed via compounds 1-II and 1-III in 60% overall yield (See Supporting Information). Treatment of 1 with 1.2 equiv of potassium hexamethyldisilazide (KHMDS) in DMF at –60 °C gave an inseparable 57:43 mixture of 2a and 2b in a combined yield of 68% via an intramolecular conjugate addition reaction (Scheme 1). Enantiomeric purity of 2a and 2b was determined to be 98% ee and 97% ee, respectively, after their conversion to amides 3a and 3b.
We then examined the reaction at various temperatures and the results are shown in Figure 1. The diastereomeric ratio was found to be totally dependent on the temperature, while the ee was almost independent from the temperature. For example, the reaction at –40 °C gave a 63:37 diastereo mixture of 2a (94% ee) and 2b (91% ee) in a combined yield of 63%, while that at 27 °C gave a 9:91 diastereo mixture of 2a (95% ee) and 2b (93% ee) in a combined yield of 89%. In order to investigate the origin of the high diastereoselectivity at the higher temperature, the possibility of equilibrium was examined. Treatment of a 60:40 mixture of 2a (93% ee) and 2b (93% ee) with 1.2 equiv of KHMDS in DMF at 0 °C for 10 min gave a 6:94 mixture of 2a (91% ee) and 2b (92% ee) in a combined yield of 81% (Scheme 2). This result clearly indicates that the high diastereomeric ratios observed in the asymmetric intramolecular conjugate addition of 1 at higher temperatures were the results from thermodynamic equilibrium. Although the intervention of chiral enolate intermediates with short half-lives of racemization for this equilibrium process is assumed, the decrease in the enantiomeric purity of 2a and 2b was less than 2%.
The similar effects of temperature on diastereoselectivity were observed in another five-membered cyclization (Scheme 3). Treatment of 4 with 1.2 equiv of KHMDS at –78 °C in THF-DMF for 30 min gave a 80:20 mixture of 5a (91% ee) and 5b (94% ee) in a combined yield of 81%,1 while that in DMF at 0 °C for 10 min gave an 11:89 mixture of 5a (96% ee) and 5b (97% ee) in a combined yield of 53%. The asymmetric conjugate addition of 4 in DMF at 0 °C preceded in the slightly higher enantioselectivity than that in THF-DMF at –78 °C. This could be ascribed to the higher enantiomeric purity of the intermediary axially chiral enolate generated in DMF solution than that in THF-DMF solution. We have reported that use of KHMDS in DMF is most suitable for highly enantioselective asymmetric intramolecular alkylation in retention of configuration via memory of chirality, while the use of KHMDS in less polar solvents resulted in the reduced enantioselectivity. Furthermore, the use of LTMP in THF promotes the reaction to proceed in inversion of the configuration.9c
The relative and absolute configuration of 2a was determined to be (2R,3R) by an X-ray crystallographic analysis of 3a (Figure 2).6 The relative and absolute configuration of 5a was already determined to be (2R,3S) by an X-ray crystallographic analysis of its derivative.1 Thus, intramolecular conjugate addition of 1 and 4 was found to proceed with retention of configuration at the newly formed tetrasubstituted carbon center.7
A rationale for the stereochemical course of asymmetric intramolecular conjugate addition is shown in Scheme 4. A conformational search of 1 gave stable conformers, I and II.8 Deprotonation of conformer II with KHMDS, where the C(α)-H bond is oriented antiperiplanar with respect to the N-Boc bond, would be preferable to that of conformer I, where the C(α)-H bond is oriented antiperiplanar with respect to the N-Ar bond. This assumption is based on our rationale for the previous stereochemical results, in which deprotonation of N-Boc-N-alkyl-α-amino acid derivatives preferentially took place from the conformer in which C(α)-H bond is oriented antiperiplanar with respect to the N-Boc bond.9,10 Deprotonation of conformer II would give enantiomerically enriched enolate A with a chiral (aS)-C-N axis. Enolate B with a chiral (aS)-C-N axis and different conformation would be formed by fast rotation of a C-C bond. Importantly, both enolates A and B have same axial chirality around the C-N bond. Enolate A would undergo intramolecular conjugate addition from its re-face to give enolate C with retention of the configuration at C(2). Similarly, enolate B would give enolate D with the same absolute configuration at C(2) as that of C. Protonation of C and D at the work-up process would give (2R,3R)-2a and (2R,3S)-2b, respectively. Thermodynamically more stable diastereomer 2b was expected to be obtained via the equilibrium process between enolates C and D. A DFT calculation also indicated that a cis-enolate such as D is more stable than a trans-enolate such as C by 1~2 kcal/mol (For the calculation, methyl esters instead of ethyl and tert-butyl esters and methyl carbamate instead of tert-butyl carbamate were employed, respectively. See Suppoting Information for the details). The decrease in enantiomeric purity of 2a and 2b was less than 2% even after the equilibrium between the chiral enolates has been achieved (Scheme 2). This indicates that the interconversion between chiral enolates A and B via C-C bond rotation is much faster than the racemization of axially chiral enolates A and B via the C-N bond rotation to give ent-A and ent-B, respectively. Similarly, the diastereomeric ratio (5a:5b=11:89) obtained by the reaction of 4 at 0 °C (Scheme 3) is assumed to be the results from the thermodynamic equilibrium of the intermediary chiral enolates. The observed high ee of 5a (96% ee) and 5b (97% ee) indicates negligible extent of racemization of the intermediary chiral enolates during the equilibrium process.
In summary, an indoline derivative with contiguous tetra- and trisubstituted carbon centers was prepared by intramolecular conjugate addition of an α-amino acid derivative in highly enantioselective manner. Enantioselectivity of the reaction was almost independent from the reaction temperature, while diastereoselectivity was totally dependent on the temperature. A racemization-free equilibrium process between the axially chiral enolate intermediates was proposed to be the origin for the high diastereoselectivity observed in the reactions at the higher temperatures.
EXPERIMENTAL
General. Melting points were measured with a Yanagimoto micro point apparatus and uncorrected. NMR spectra were obtained with Varian Gemini 200 (200 MHz), JEOL JNM-AL 400, or JEOL JNM-ECX 400 (400 MHz) spectrometers, chemical shifts being given in ppm units (tetramethylsilane or chloroform as internal standards, indicating 0 or 7.24, respectively). IR spectra were recorded with a JASCO FT/IR–300 spectrometer. Specific rotations were measured with a Horiba SEPA–200 automatic digital polarimeter. MS spectra were recorded with a JEOL JMS–DX300 mass spectrometer. TLC analysis and preparative TLC were performed on commercial glass plates bearing a 0.25 mm layer and 0.5 mm layer of Merck Kiesel–gel 60 F254, respectively. Silica gel column chromatography was carried out by Wakogel C–200, Fuji Silysia BW–1277H, or Nacalai Tesque Silica gel 60 (150–325 mesh). Dehydrated solvents (THF, ether, hexane, dichloromethane, and toluene; <50 ppm water contents) were purchased from Kanto Chemical CO., Inc. and used without further treatment.
Asymmetric intramolecular conjugate addition of 1 (Scheme 1 and Fig. 1 in the text): Potassium hexamethyldisilazide (KHMDS) (1.40 M in THF, 0.16 mL, 0.23 mmol) was slowly added to a solution of 1 (84 mg, 0.19 mmol) in DMF (2.0 mL) at –60 °C. The reaction mixture was stirred at the same temperature for 10 min, and then quenched with sat. aq. NH4Cl solution and extracted with AcOEt. The organic layer was dried over Na2SO4, filtered, and evaporated to give a residue. The crude product was purified by column chromatography (12:1 hexane-AcOEt) to give a 57:43 diastereomeric mixture of 2a and 2b (57 mg, 68% combined yield) as a viscous liquid. Separation and identification of each diastereomer were carried out after their conversion into 3a and 3b. Their optical purities were determined by chiral HPLC using 3a and 3b.
Equilibrium experiment (Scheme 2): KHMDS (93 µL, 0.43 M in THF, 0.04 mmol) was added to a solution of a 60:40 diastereomeric mixture of 2a (93% ee) and 2b (93% ee) (16 mg, 0.04 mmol) in DMF (0.5 mL) at 0 °C. After stirring for 10 min at the same temperature, the reaction was quenched by addition of sat. aq. NH4Cl and the resulting mixture was stirred for 10 min and extracted with AcOEt. The extracts were washed with brine and dried over Na2SO4, filtered and concentrated. The residue was purified by PTLC (hexane/AcOEt = 3/1, Rf = 0.3) to give a 6:94 diastereomeric mixture of 2a (91% ee) and 2b (92% ee) (13 mg, 81% combined yield).
Conversion of 2a and 2b into their p-bromoanilide derivatives 3a and 3b (Scheme 1): 4 M HCl in AcOEt solution (1 mL) was added to an inseparable diastereomeric mixture of 2a and 2b (57 mg, 0.13 mmol) at room temperature. After stirring for 20 h, the reaction mixture was evaporated to give a residue. To the residual oil were added WSC-HCl (37 mg, 0.20 mmol), DMAP (1.0 mg) and p-bromoaniline (22 mg, 0.13 mmol) and the resulting mixture was dissolved in CH2Cl2 (1 mL) under Ar at room temperature. After stirring for 40 h, the reaction mixture was quenched with 1 M HCl and extracted with CH2Cl2. The organic layer was washed with brine, dried over Na2SO4, filtered, and evaporated. The residual oil was purified by preparative TLC with hexane/AcOEt (3/1, Rf = 0.3) to give 3a (23 mg, 0.05 mmol) and 3b (20 mg, 0.05 mmol) in 75% combined yield.
3a: colorless needles, mp 125-127 °C; [α]D20 –173.5 (c 0.70, CHCl3, 94% ee), HPLC conditions: Chiralcel OD column, 254 nm, flow: 1.00 mL/min, 7% i-PrOH in hexane, time: 25.2 min (minor), 30.7 min (major); IR (CHCl3) 3362, 2973, 1723, 1662, 1604, 1533, 1487, 1394, 1306, 1222, 1070, 1013, 823, 770 cm-1; 1H-NMR (400 MHz, CDCl3) δ: 7.40 (d, J = 8.8 Hz, 2H), 7.31 (d, J = 8.8 Hz, 2H), 7.07–7.02 (m, 3H), 6.73 (d, J = 7.6 Hz, 1H), 6.67 (t, J = 7.6 Hz, 1H), 4.85 (br s, 1H), 4.23–4.06 (m, 3H), 2.84 (dd, J = 4.0, 13.6 Hz, 1H), 2.32–2.17 (m, 2H), 1.21 (t, J = 7.2 Hz, 3H), 1.07 (d, J = 7.2 Hz, 3H), 1.00 (d, J = 6.8 Hz, 3H); 13C-NMR (100 MHz, CDCl3) δ: 174.5, 169.6, 149.1, 136.7, 131.9, 130.6, 128.5, 124.9, 121.5, 119.9, 116.9, 110.5, 61.6, 43.9, 38.6, 31.2, 18.8, 18.3, 14.3; MS (EI) m/z 446 (81Br, M+), 444 (79Br, M+), 403, 401, 373, 371, 323, 321, 293, 292, 230, 202, 172, 130 (100%), 91, 77, 65; HRMS (EI) m/z calcd. for C22H25BrN2O3 (M+) 444.1049, 446.1028. Found 444.1038, 446.1031. Anal. Calcd for C22H25BrN2O3: C, 59.33 H, 5.66 N, 6.29. Found: C, 59.19 H, 5.84 N, 6.00.
3b: yellowish oil: [α]D20 +99.4 (c 0.70, CHCl3, 93% ee), HPLC conditions: Chiralpak AD column, 254 nm, flow: 1.00 mL/min, 20% i-PrOH in hexane, time: 9.98 min (minor), 14.2 min (major); IR (CHCl3) 3357, 2968, 1723, 1665, 1600, 1533, 1489, 1394, 1248, 1033, 824, 771 cm-1; 1H-NMR (400 MHz, CDCl3) δ: 7.61 (br s, 1H), 7.42-7.37 (m, 4H), 7.04 (t, J = 7.6 Hz, 1H), 6.94 (d, J = 7.6 Hz, 1H), 6.68–6.64 (m, 2H), 4.42 (br s, 1H), 4.25–4.09 (m, 2H), 3.96 (t, J = 7.2 Hz, 1H), 2.70 (dd, J = 6.8, 14.8 Hz, 1H), 2.50 (dd, J = 7.6, 14.8 Hz, 1H), 2.31–2.24 (m, 1H), 1.24 (t, J = 7.2 Hz, 3H), 0.89 (d, J = 6.8 Hz, 3H), 0.88 (d, J = 6.8 Hz, 3H); 13C-NMR (100 MHz, CDCl3) δ: 174.7, 169.4, 149.5, 136.9, 132.0, 129.7, 128.4, 123.6, 121.3, 119.2, 116.8, 109.6, 61.6, 45.1, 40.2, 35.5, 18.1, 16.7, 14.2; MS (EI) m/z 446 (81Br, M+), 444 (79Br, M+), 403, 401, 373, 371, 323, 276, 247, 231, 202 (100%), 172, 130, 83, 58; HRMS (EI) m/z calcd. for C22H25BrN2O3 (M+) 444.1049, 446.1028. Found 444.1049, 446.1036. Anal. Calcd for C22H25BrN2O3: C, 59.33 H, 5.66 N, 6.29. Found: C, 58.93 H, 5.59 N, 6.15.
Preparation of 4: A mixture of phenylalanine ethyl ester hydrochloride (229 mg, 1.0 mmol), K2CO3, (304 mg, 2.2 mmol), 4-bromo-1-butene (169 mg, 1.25 mmol), NaI (15 mg, 0.1 mmol), and DMF (1 mL) was heated at 70 °C for 4 h with stirring. The reaction mixture was poured into ice-water mixture and extracted with Et2O. The combined organic layers were washed with brine, dried over Na2SO4, filtered and concentrated under reduced pressure. The crude material was passed through the short pad of silica gel. Elution with hexane/AcOEt (5/1, Rf = 0.3) gave N-monoalkyl product. A mixture of the N-monoalkyl product, Boc2O (262 mg, 1.2 mmol), and DIPEA (143 mg, 1.1 mmol) was dissolved in CH2Cl2 (1 mL). After stirring for 40 h at room temperature, the reaction was quenched with cold water and extracted with CH2Cl2. The combined organic layers were washed with brine, dried over Na2SO4, filtered and concentrated under reduced pressure. The residual oil was loaded on a silica gel column and eluted with hexane/AcOEt (4/1, Rf = 0.3) to give a colorless oil. Ozone was passed through a solution of the crude product in MeOH (1 mL) at –60 °C until blue color appeared. Me2S (1 mL) was added to the mixture and the resulting mixture was warmed up to room temperature. After evaporation of solvent and excess Me2S, the crude product was dissolved in CH2Cl2 and (tert-butoxycarbonylmethylene)triphenylphosphorane (489 mg, 1.3 mmol) was added. The resulting mixture was stirred at room temperature for 20 h. After removal of volatiles, the crude product was loaded on a silica gel column and eluted with hexane/AcOEt (9/1, Rf = 0.3) to afford 4 (312 mg, 0.70 mmol) in 70% yield in 4 steps.
4: viscous liquid (mixture of rotamers), [α]D20 –98.5 (c 1.00, CHCl3); IR (CHCl3) 2990, 2965, 1740, 1705, 1440, 1365, 1162 cm-1; 1H-NMR (400 MHz, CDCl3) δ: 7.30–7.14 (m, 5H), 6.70–6.65 (m, 1H), 5.68–5.61 (m, 1H), 4.30–4.09 (m, 2H), 3.97–3.93 (m, 1H), 3.33–3.10 (m, 3H), 2.74-2.57 (m, 1H), 2.22–2.12 (m, 2H), 1.46 (br s, 18H), 1.31–1.22 (m, 3H); 13C-NMR (100 MHz, CDCl3) δ: 170.6, 170.5, 165.3, 154.4, 154.0, 144.1, 143.9, 137.8, 137.7, 128.9, 128.3, 128.1, 126.4, 126.2, 124.1, 124.0, 80.6, 80.1, 79.9, 79.8, 65.7, 63.1, 62.3, 61.2, 61.0, 47.9, 47.4, 36.2, 35,3, 31.6, 30.7, 28.2, 28.1, 28.0, 15.2, 14.1; MS (EI) m/z 447(M+), 391, 374, 335, 318, 306, 274, 218, 206 (100%), 132, 91; HRMS (EI) m/z calcd. for C25H37NO6 (M+) 447.2621. Found 447.2614. Anal. Calcd for C25H37NO6: C, 67.09 H, 8.33 N, 3.13. Found: C, 66.73 H, 8.43 N, 3.15.
Asymmetric intramolecular conjugate addition of 4 at –78 °C (Scheme 3 in the text): KHMDS (0.56 M in THF, 1.0 mL, 0.55 mmol) was diluted with THF-DMF (1:1, 3.5 mL) and cooled down to –78 °C. A solution of 4 (223 mg, 0.50 mmol) in THF-DMF (1:1, 1.5 mL) was added over 5 min. After stirring for 30 min, the reaction mixture was quenched with aq. NH4Cl and extracted with AcOEt. The organic layer was washed with brine, dried over Na2SO4, filtered, and evaporated to give crude compound. The residual oil was purified by preparative TLC with hexane/AcOEt (5/1, Rf = 0.3) to afford a 4/1 diastereomeric mixture of 5a and 5b (145 mg, 0.32 mmol) in 81% combined yield. A small amount of a 4/1 diastereomeric mixture of 5a and 5b was separated by preparative TLC (hexane/AcOEt = 5/1) to take spectra data. The optical purities of each diastereomer were determined by chiral HPLC after their conversion to the corresponding N-benzoylamides.
A 1/1 mixture of rotamers of 5a: sticky solid; [α]D20 –22.6 (c 1.50, CHCl3, 91% ee). IR (CHCl3) 2977, 1730, 1695, 1478, 1454, 1395, 1153 cm-1; 1H-NMR (400 MHz, d8–toluene) δ: 7.20–7.17 (m, 2H), 7.07–7.03 (m, 3H), 4.24–4.12 (m, 1H), 4.03–3.95 (m, 1H), 3.90–3.82 (m, 0.5H), 3.62 (d, J = 14.6 Hz, 0.5H), 3.48–3.35 (m, 1H), 3.26–3.19 (m, 0.5H), 3.09–2.93 (m, 2H), 2.87 (d, J = 14.6 Hz, 0.5H), 2.72 (dd, J = 4.7 , 16.2 Hz, 0.5H), 2.57 (dd, J = 5.6, 15.9 Hz, 0.5H), 2.40 (dd, J = 10.0, 16.2 Hz, 0.5H), 2.23 (dd, J = 9.3, 16.2 Hz, 0.5H), 1.65–1.59 (m, 0.5H), 1.53–1.47 (m, 0.5H), 1.46 (s, 4.5H), 1.35 (s, 4.5H), 1.34 (s, 9H), 1.09 (t, J = 7.2 Hz, 1.5H), 1.08 (t, J = 7.2 Hz, 1.5H), 0.86-0.68 (m, 1H); 13C-NMR (100 MHz, CDCl3) δ: 173.6, 173.5, 171.1, 170.9, 153.5, 153.3, 137.9, 137.6. 130.7, 130.6, 128.4, 128.1, 126.5, 126.3, 81.0, 80.9, 80.5, 79.6, 70.0, 69.9, 61.3, 61.2, 46.7, 46.6, 45.8, 44.5, 35.9, 34.8, 34.7, 29.1, 28.5, 28.3, 28.1, 14.2, 14.1; MS (EI) m/z 447 (M+), 402, 356, 318, 300, 262, 244, 200 (100%), 154, 91; HRMS (EI) m/z calcd. for C25H37NO6 (M+) 447.2621. Found 447.2622. Anal. Calcd for C25H37NO6: C, 67.09 H, 8.33 N, 3.13. Found: C, 66.71 H, 8.24 N, 3.05.
A 1/1 mixture of rotamers of 5b: sticky solid; [α]D20 –73.9 (c 0.90, CHCl3, 94% ee). IR (CHCl3) 2977, 2876, 1730, 1695, 1454, 1393, 1152 cm-1; 1H-NMR (400 MHz, d8–toluene) δ: 7.36–7.32 (m, 2H), 7.18–7.14 (m, 2H), 7.07–7.04 (m, 1H), 4.17 (d, J = 14.0 Hz, 0.5H) 4.10–3.77 (m, 3H), 3.56–3.51 (m, 0.5H), 3.19 (d, J = 14.4 Hz, 1H), 2.85–2.75 (m, 1H), 2.60–2.42 (m, 2H), 2.01 (dd, J = 15.6, 10.0 Hz, 0.5H), 1.97 (dd, J = 16.0, 10.8 Hz, 0.5H), 1.56–1.41 (m, 10H), 1.36–1.34 (m, 10H), 1.03–0.94 (m, 3H); 13C-NMR (100 MHz, CDCl3) δ: 172.8, 172.7, 171.2, 170.9, 154.1, 153.5, 136.7, 136.4, 131.2, 131.0, 128.2, 127.9, 126.7, 126.4, 80.9, 80.8, 80.6, 79.6, 70.3, 70.2, 61.3, 61.1, 47.1, 46.8, 42.2, 41.0, 37.3, 36.4, 36.2, 36.1, 28.5, 28.4, 28.1, 28.0, 14.4, 14.3; MS (EI) m/z 447 (M+), 419, 374, 346, 328, 300, 262, 228, 200 (100%), 154; HRMS (EI) m/z calcd. for C25H37NO6 (M+) 447.2621. Found 447.2633. Anal. Calcd for C25H37NO6: C, 67.09 H, 8.33 N, 3.13. Found: C, 66.98 H, 8.31 N, 3.06.
HPLC conditions for N-benzoylamides
N-Benzoylamide derived from 5a: Chiralpak AD column, flow: 1.75 ml/min, 10% i-PrOH in hexane, time: 11.7 min (major), 17.8 min (minor).
N-Benzoylamide derived from 5b: Chiralpak AD column, flow: 1.5 ml/min, 6% i-PrOH in hexane, time: 10.9 min (major), 13.4 min (minor).
References
1. T. Kawabata, S. Majumdar, K. Tsubaki, and D. Monguchi, Org. Biomol. Chem., 2005, 3, 1609. CrossRef
2. For reviews on asymmetric synthesis via memory of chirality, see (a) T. Kawabata and K. Fuji, Topics in Stereochemistry, 2003, 53, 175; (b) H. Zhao, D. Hsu, and P. R. Carlier, Synthesis, 2005, 1; CrossRef (c) T. Kawabata, ‘Asymmetric Synthesis and Application of α-Amino Acids,’ ACS Symposium Series 1009, 2009, pp.31-56.
3. For recent examples of asymmetric synthesis based on memory of chirality, see: (a) G. Gerona-Navarro, B. A. Bonache, R. Herrnz, M. T. García-López, and R. González-Muñiz, J. Org. Chem., 2001, 66, 3538; CrossRef (b) M. A. Bonache, G. Gerona-Navarro, C. García-Aparicio, M. Alías, M. Martín-Martínez, M. T. García-López, P. López, C. Cativiela, and R. González-Muñiz, Tetrahedron: Asymmetry, 2003, 14, 2161; CrossRef (c) M. A. Bonache, G. Gerona-Navarro, M. Martín-Martínez, M. T. García-López, P. López, C. Cariviela, and R. González-Muñiz, Synlett, 2003, 1007; CrossRef (d) P. R. Carlier, H. Zhao, S. L. MacQuarrie-Hunter, J. C. DeGuzman, and D. C. Hsu, J. Am. Chem. Soc., 2006, 128, 15215; CrossRef (e) M. A. Bonache, C. Cativiela, M. T. García-López, and R. González-Muñiz, Tetrahedron Lett., 2006, 47, 5883; CrossRef (f) L. Klolaczkowski and D. M. Barnes, Org. Lett., 2007, 9, 3029; CrossRef (g) M. Branca, D. Gori, R. Guillot, V. Alezra, and C. Kouklovsky, J. Am. Chem. Soc., 2008, 130, 5864; CrossRef (h) M. Nechab, D. Campolo, J. Maury, P. Perfetti, N. Vanthuyne, D. Siri, and M. R. Bertrand, J. Am. Chem. Soc., 2010, 132, 14742. CrossRef
4. For a review, see: L. Dianyu, Z. Guowei, and X. Lan, Eur. J. Org. Chem., 2010, 3975. CrossRef
5. D. Ma, Y. Zhang, J. Yao, S. Wu, and F. Tao, J. Am. Chem. Soc., 1998, 120, 12459. CrossRef
6. Crystal data for 3a: C22H25BrN2O3, M = 445.35, monoclinic, a = 31.093(7) Å, b = 5.7381(10) Å, c = 11.952(2) Å, α = 90.00˚, β = 96.447(10)˚, γ = 90.00˚, V = 2119.0(7) Å3, T = 103(2) K, space group C2, Z = 4, μ(MoKα) = 0.71070 mm–1, 8805 reflections measured, 3907 independent reflections (Rint = 0.0383). The final R1 values were 0.0583 (I > 2σ(I)). The final wR(F2) values were 0.1453 (I > 2σ(I)). The final R1 values were 0.0865 (all data). The final wR(F2) values were 0.1733 (all data). The goodness of fit on F2 was 1.115. The Flack parameter was –0.034(17). CCDC 802933 contains the crystallographic data for 3a. These data can be obtained free of charge from The Cambridge Crystallographic Data Centre via www.ccdc.cam.ac.uk/products/csd/request/.
7. A similar phenomenon was observed in the asymmetric synthesis of β-lactams via axially chiral enolates, see: T. Yoshimura, M. Takuwa, K. Tomohara, M. Uyama, K. Hayashi, P. Yang, R. Hyakutake, T. Sasamori, N. Tokitoh, and T. Kawabata, Chem. Eur. J., DOI: 10.1002/chem.20121339. CrossRef
8. The stable conformers I and II were generated by a molecular modeling search (MCMM 50,000 steps) with OPLS 2005 force field with GB/SA solvation model for chloroform using MacroModel (V. 9.0). The difference in potential energies between I and II is estimated to be 1.41 kcal/mol (I is more stable than II). The stereoview structure of the conformers I and II is shown in Supporting information.
9. (a) T. Kawabata, H. Suzuki, Y. Nagae, and K. Fuji, Angew. Chem. Int. Ed., 2000, 39, 2155; CrossRef (b) T. Kawabata, S. Kawakami, and S. Majumdar, J. Am. Chem. Soc., 2003, 125, 13012; CrossRef (c) T. Kawabata, S. Matsuda, S. Kawakami, D. Monguchi, and K. Moriyama, J. Am. Chem. Soc., 2006, 128, 15394; CrossRef (d) T. Kawabata, K. Moriyama, S. Kawakami, and K. Tsubaki, J. Am. Chem. Soc., 2008, 130, 4153; CrossRef (e) K. Moriyama, H. Sakai, and T. Kawabata, Org. Lett., 2008, 10, 3883; CrossRef (f) F. Teraoka, K. Fuji, O. Ozturk, T. Yoshimura, and T. Kawabata, Synlett, 2011, 543. CrossRef
10. Recently asymmetric aldol reaction via memory of chirality has been developed, see: T. Watanabe, T. Yoshimura, S. Kawakami, T. Sasamori, N. Tokitoh, and T. Kawabata, Chem. Commun., 2012, 48, 5346. CrossRef