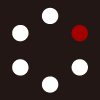
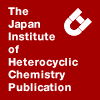
HETEROCYCLES
An International Journal for Reviews and Communications in Heterocyclic ChemistryWeb Edition ISSN: 1881-0942
Published online by The Japan Institute of Heterocyclic Chemistry
e-Journal
Full Text HTML
Received, 15th May, 2012, Accepted, 2nd August, 2012, Published online, 13th August, 2012.
DOI: 10.3987/COM-12-S(N)18
■ α-SUBSTITUTED UNSYMMETRICAL PHTHALOCYANINES WITH ONE THROUGH THREE TTF UNITS AND THEIR OPTICAL AND ELECTROCHEMICAL PROPERTIES
Takeshi Kimura,* Nobuhiro Takahashi, Tomoyuki Tajima, and Yutaka Takaguchi
Center for Instrumental Analysis, Iwate University, 4-3-5 Ueda, Morioka, Iwate 020-8551, Japan
Abstract
α-Substituted unsymmetrical phthalocyanines fused with one through three TTF units were prepared on treatment of mixed 3,6-dioctyltetrathia- fulvalenophthalonitrile and 3,6-dioctoxyphthalonitrile with lithium alkoxide at 120 °C. The optical and electrochemical properties of the products were determined by UV-vis spectroscopy and cyclic voltammetry.Phthalocyanines (Pcs) have actual and potential applications as catalysts, optical devices, semiconductors, sensitizers for photodynamic therapy for cancer, and so on.1 To develop new functional materials based on phthalocyanines, scientists have been required to synthesize a new type of compounds. Therefore, there have been a great number of reports about functionalized Pcs and related compounds fused with a variety of π-aromatic systems.1,2 Pcs with α-substituents were recently reported to show Q-band absorption at a wavelength longer than β-substituted derivatives,3 which offer the possibility of tuning the wavelength of their absorption and emission bands. On the other hand, tetrathiafulvalene (TTF) is a strong electron donor and its electrochemical property is useful to develop new functional materials.4 TTF derivatives have been bonded to Pcs by using some linkers and the electron transfer process was estimated between the TTF unit and the Pc core.5 In contrast, Decurtins et al. first reported Pcs with four TTF units directly fused to the macrocycle.6 By this procedure, however, it is impossible to introduce any substituents on the α-positions of Pcs.7 Although unsymmetrical Pcs can possess unique optical and electrochemical properties that are different from symmetrical ones,1 there are a few reports of unsymmetrical Pcs fused with the TTF unit.8 So, we tried to prepare α-substituted unsymmetrical Pcs fused with the TTF unit. This paper reports the preparation of unsymmetrical α-octyloxy Pcs with one through three TTF units and their optical and electrochemical properties.
As a starting compound for α-substituted unsymmetrical Pcs with the TTF unit, TTF annelated 3,6-dioctylphthalonitrile (1) was prepared from 1,4-dichlorobenzene via seven step reactions by the procedure reported previously.7 In addition, 3,6-dioctyloxyphthalonitrile (2) was synthesized on treatment of 2,3-dicyanodihydrobenzoquinone with octyl bromide. Since three approaches have been used to prepare unsymmetrical Pcs up to now, we tried the statistical procedure.9 Thus, phthalonitriles 1 and 2 were mixed in a 1 : 6 ratio and the mixture was treated with lithium alkoxide in 1-hexanol at 120 °C for 3 h to give mono-TTF derivative 3 (25%), di-TTF derivatives 4 (3%) and 5 (1%) as adjacent and opposite isomers, and tri-TTF derivative 6 (trace), after purification with column chromatography using silica gel and BioBeads (Scheme 1). By this process, we could not isolate α-octaoctyloxyphthalocyanine well.
Although only Pc with one TTF unit and six β-octyloxy groups [βPcTTF1] could be isolated in the β-octyloxy derivatives,8b adjacent isomer 4, opposite isomer 5, and 6 could be separated as well as 3. It seemed that α-octyloxy derivatives are highly polarized molecules compared with β-octyloxy derivatives. As a result, α-octyloxy derivatives 3, 4, 5, and 6 could be isolated, respectively. Compound 3 was further reacted with nickel (II) acetate at 150 °C in DMF for 3 h to produce the corresponding nickel (II) complex 3-Ni in 99% yield. In the 1H NMR spectra of 3 and 3-Ni, one singlet peak was observed for two methylthio groups, and one singlet and two doublet peaks appeared for aromatic protons, respectively. Adjacent isomer 4 showed two singlet peaks for the methylthio groups and two doublet peaks for the aromatic protons. In contrast, we could observe the methylthio groups and the aromatic protons as one singlet peak for opposite isomer 5. In the spectrum of 6, two singlet peaks for the methylthio groups were observed in a 1 : 2 integral ratio and one singlet peak was found for the aromatic protons. Inner pyrrole protons of the products were observed at δ = 0.19 ppm for 3, δ = 0.22 ppm for 4, δ = 0.07 ppm for 5, and δ = 0.22 ppm for 6. βPcTTF1 showed the chemical shift for the inner pyrrole protons at δ = –3.71 ppm8b and the signal of α-octaoctylphthalocyanine fused with four TTF units [PcTTF4] was observed at δ = 0.17 ppm.7b On the other hand, the octyl and octyloxy groups at α-positions of the products showed complex signals in the spectra. Matrix assisted laser desorption ionization time of flight mass spectrometry (MALDI-TOF MS) experiments showed the corresponding molecular ion peak for Pcs produced: m/z = 1774.17 [M+] for 3, m/z = 2010.47 [M+] for 4, m/z = 2010.37 [M+] for 5, m/z = 2244.96 [M+–2] for 6, and m/z = 1830.24 [M+] for 3-Ni (matrix: dithranol).
In the UV-vis spectrum, the Q-band absorption of α-octyloxy derivative 3 was observed at 771 nm together with its second component (750 nm) as shown in Figure 1a. In contrast, βPcTTF1 showed the Q band absorption as split peaks at 681 nm and 705 nm.8b While the nickel complex βPcTTF1Ni showed the absorption as a broad signal at 680 nm,8b the Q band absorption of the nickel complex 3-Ni was observed at 744 nm as a sharp signal. The Q band absorption of the α-octyloxy derivatives 3 and 3-Ni shifted to the lower energy field about 66 nm and 64 nm compared with that of βPcTTF1 and βPcTTF1Ni, respectively. In addition, we could detect that the Q band absorptions of compounds 4, 5, and 6 are similar to that of the mono-TTF derivative 3 and the λmax values are 768 nm, 765 nm, and 760 nm, respectively. While the second component of 6 was not clear, compounds 4 and 5 showed a shoulder peak at 750 nm and 748 nm in the Q band absorption, respectively. The Q band absorption of α-octaoctyloxyphthalocyanine was observed at 774 nm and the inner pyrrole protons of this compound were observed at δ = 0.23 ppm in the 1H NMR spectrum. Since it was suggested that Pcs with the Q band absorption at the longer wavelength have the smaller ring current,3a we could explain that the chemical shifts of the inner pyrrole protons of 3, 4, 5, and 6 were observed at the lower magnetic field than that of βPcTTF1. It was reported that while the electron donating group at the α-positions shifts the Q band absorption to the lower energy field, that at the β-positions cause the blue shift of the absorption.3a Therefore, the blue shift of the Q band absorption could be caused with increasing the number of the TTF unit and decreasing the number of the α-octyloxy group. The wavelength of the Q band absorption is in the following order: 3 > 4 (adjacent) > 5 (opposite) > 6. On the other hand, the Q band absorption of PcTTF4 was observed at 770 nm which is shorter wavelength than that of 3 but a lower energy region than those of 4, 5, and 6. To explain the Q band absorption of PcTTF4, a molecular symmetry and the stability of HOMO and LUMO may be important but the reason is not clear.
On measurement of the UV-vis spectrum of 3 in the presence of two times of iodine, the Q band absorption was slowly changed in the spectrum. The absorption at 771 nm gradually decreased and a new absorption appeared at the longer wavelength. After 18 h, the broadened and red shifted Q band absorption (824 and 863 nm) was observed in the spectrum as shown in Figure 1a. It is expected that compound 3 and iodine produced an electron transfer complex in the solution. In the 1H NMR spectrum measured in the presence of excess amount of iodine, compound 3 showed broadened signals for the octyl, octyloxy, and aromatic protons but we could not observe the signal for the methylthio groups. On the other hand, when the solution was measured by ESR at room temperature, one strong broadened signal was found at g = 2.00763 in the spectrum (Figure 2). The g value of the electron transfer complex 3•+ could be related to the radical cation of TTF.10 These results suggest that 3•+ has a radical cationic character, and the charge generated on the TTF unit can delocalize to the π-aromatic system of the central phthalocyanine core.
The redox potentials of 3, 3-Ni, 4, and 5 were determined by cyclic voltammetry; scan rate: 200 mV/s (Table 1). The voltammogram of 3 showed two quasi-reversible couples for oxidation (E1/2 = 0.34 and 0.69 V) and two reversible couples for reduction (E1/2 = –1.52 and –1.18 V) (Figure 1b). In the voltammogram of 3-Ni, we could observe two quasi-reversible couples for oxidation (E1/2 = 0.33 and 0.84 V) and two reversible couples for reduction (E1/2 = –1.75 and –1.31 V). The voltammgrams of the cis isomer 4 and the trans isomer 5 showed two quasi-reversible couples for oxidation and two reversible couples for reduction. The redox potentials of 4 are similar to those of 5 and the first oxidation potentials of these compounds are slightly higher than that of mono-TTF derivative 3. The first oxidation potentials of α-octyloxy derivatives 3 and 3-Ni are lower than those of βPcTTF1, βPcTTF1Ni, and PcTTF4.
To estimate the HOMO–LUMO gap of the products, we calculated the potential difference between 1st oxidation (1st oxid) and 1st reduction (1st redn) potentials (E1oxid–E1redn). These potential differences could be correlated to the HOMO–LUMO gap calculated from the λmax values of the respective Q band absorption.
In conclusion, we prepared α-substituted unsymmetrical phthalocyanines fused with one through three TTF units from mixed 3,6-dioctyltetrathiafulvalenephthalonitrile and 3,6-dioctyloxyphthalonitrile. The wavelength of the Q band absorption is in the following order: 3 > 4 (adjacent) > 5 (opposite) > 6, which is related to the numbers of the TTF units at the β-positions and the octyloxy groups at the α-positions. The first oxidation potential of α-octyloxy derivatives 3 and 3-Ni is lower than that of 4, 5, βPcTTF1, βPcTTF1Ni, and PcTTF4. The electron transfer complex generated from compound 3 and iodine has a radical cationic character in the solution, and the charge generated on the TTF unit can delocalize to the π-aromatic system of the central phthalocyanine core.
EXPERIMENTAL
General
NMR spectra were measured with a Bruker AVANCE-500 spectrometer. Mass spectra were obtained using a JEOL JMS-700 mass spectrometer and a PerSeptive Biosystems Voyager RP-DE mass spectrometer. UV-vis spectra were recorded with a JASCO Ubest V-570 spectrometer. A Hokuto Denko Co. Model HAB-151 apparatus was applied to measurements of redox potentials. ESR spectrum was measured by JEOL JES-FA100 ESR spectrometer. Bio-beads (SX-1) for column chromatography was purchased from Nippon Bio-Rad Laboratories. All measurements for redox potentials were performed by cyclic voltammetry, using Ag/AgNO3 (0.01 mol/l) as a reference electrode, glassy carbon as a working electrode, and Pt wire as a counter electrode (scan rate: 200 mV/s). A solution of n-Bu4NClO4 in CH2Cl2 (0.1 mol/l) was used as an electrolyte. The oxidation potential of ferrocene was observed at E1/2 = 0.09 V by the apparatus without any correction.
4,7-Dioctyl-5,6-dicyano-2-(4’,5’-bis(methylthio)-1’,3’-dithiole-2’-ylidene)benzo[d]-1,3-dithiole (1)
Compound (1) was prepared by using a method described previously.7
Preparation of phthalocyanines (3), (4), (5), and (6) with one through three TTF units.
Lithium (40 mg, 5.76 mmol) was placed in a glass reactor and 1-hexanol (2 mL) was added under Ar. The solution was stirred at 120 °C for 10 minutes and added to a mixture of 1 (125 mg, 0.2 mmol) and 2 (460 mg, 1.2 mmol). The solution was stirred at 120 °C for 3 h. After cooling to room temperature, MeOH was added and the resulting blue-green precipitate was filtered. The residue was purified by column chromatography (Wakogel C-300HG, CHCl3 and BioBeads, CHCl3) to produce 3 (25%, 89 mg), 4 (3%, 6.1 mg), 5 (1.8 mg, 1%), and 6 (trace); 3: 1H NMR (500 MHz, CDCl3) δ 0.19 (s, 2H, NH), 0.67 (t, J = 6.9 Hz, 6H, CH3), 0.79-0.86 (m, 18H, CH3), 0.86-1.70 (m, 80H, CH2), 2.01 (quint, J = 7.3 Hz, 4H, CH2), 2.19 (quint, J = 7.3 Hz, 8H, CH2), 2.26 (quint, J = 7.3 Hz, 4H, CH2), 2.51 (s, 6H, SCH3), 4.63 (t, J = 7.3 Hz, 4H, CH2), 4.67 (t, J = 7.3 Hz, 4H, CH2), 4.84 (t, J = 7.3 Hz, 8H, CH2), 7.52 (d, J = 7.9 Hz, 2H, ArH), 7.585 (d, J = 7.9 Hz, 2H, ArH), 7.593 (s, 2H, ArH); MALDI-TOF-MS (m/z) 1774.173 [M+]; 4: 1H NMR (500 MHz, CDCl3) δ 0.22 (s, 2H, NH), 0.68 (t, J = 6.9 Hz, 6H, CH3), 0.76 (t, J = 6.9 Hz, 6H, CH3), 0.79-0.86 (m, 12H, CH3), 1.00-1.38 (m, 48H, CH2), 1.38-1.54 (m, 16H, CH2), 1.54-1.69 (m, 16H, CH2), 1.91-2.09 (m, 8H, CH2), 2.16-2.29 (m, 8H, CH2), 2.512 (s, 6H, SCH3), 2.516 (s, 6H, SCH3), 4.35 (t, J = 7.6 Hz, 4H, CH2), 4.62 (t, J = 7.1 Hz, 4H, CH2), 4.69 (t, J = 7.1 Hz, 4H, CH2), 4.83 (t, J = 7.3 Hz, 4H, CH2), 7.55 (d, J = 8.7 Hz, 2H, ArH), 7.61 (d, J = 8.7 Hz, 2H, ArH); MALDI-TOF-MS (m/z) 2010.371 [M+]; 5: 1H NMR (500 MHz, CDCl3) δ 0.07 (s, 2H, NH), 0.66 (t, J = 6.9 Hz, 12H, CH3), 0.85 (t, J = 6.9 Hz, 12H, CH3), 0.98-1.54 (m, 64H, CH2), 1.54-1.71 (m, 16H, CH2), 1.99 (quint, J = 7.3 Hz, 8H, CH2), 2.25 (quint, J = 7.3 Hz, 4H, CH2), 2.52 (s, 6H, SCH3), 4.60 (t, J = 7.3 Hz, 8H, CH2), 4.66 (t, J = 7.3 Hz, 8H, CH2), 7.50 (s, 4H, ArH); MALDI-TOF-MS (m/z) 2010.371 [M+]; 6: 1H NMR (500 MHz, CDCl3) δ 0.22 (s, 2H, NH), 0.68 (t, J = 6.9 Hz, 6H, CH3), 0.74 (t, J = 7.1 Hz, 6H, CH3), 0.75 (t, J = 6.9 Hz, 6H, CH3), 0.83 (t, J = 6.8 Hz, 6H, CH3), 0.99-1.69 (m, 64H, CH2), 1.52-1.69 (m, 16H, CH2), 1.89-2.02 (m, 12H, CH2), 2.21-2.28 (m, 4H, CH2), 2.510 (s, 12H, SCH3), 2.514 (s, 6H, SCH3), 4.31-4.4.39 (m, 8H, CH2), 4.56-4.68 (m, 8H, CH2), 7.50 (s, 2H, ArH); MALDI-TOF-MS (m/z) 2244.955 [M+–2].
Preparation of phthalocyaninato nickel (II) (3-Ni)
Phthalocyanine (3) (39.2 mg, 0.022 mmol) and Ni(OAc)2•4H2O (62 mg, 0.25 mmol) were placed in a glass reactor, and DMF (5 mL) was added under Ar. The solution was stirred at 155 °C for 3 h. After cooling the reactor, methanol was added to the reaction mixture. The green precipitate was filtered and the residue was dissolved in chloroform. After evaporation, the product was purified by column chromatography (Wakogel C-300HG, CHCl3) to give 3-Ni in 99% yield (40.0 mg); 3-Ni: 1H NMR (500 MHz, CDCl3) δ 0.69 (t, J = 6.9 Hz, 6H, CH3), 0.81-0.88 (m, 18H, CH3), 1.00-1.68 (m, 80H, CH2), 1.92 (quint, J = 7.3 Hz, 4H, CH2), 2.15-2.28 (m, 12H, CH2), 2.52 (s, 6H, SCH3), 4.57 (t, J = 7.3 Hz, 8H, CH2), 4.76 (t, J = 6.8 Hz, 8H, CH2), 7.45 (d, J = 8.9 Hz, 2H, Ar-H), 7.49 (s, 2H, ArH), 7.50 (d, J = 8.9 Hz, 2H, ArH); MALDI-TOF-MS (m/z) 1830.244 [M+].
References
1. K. M. Kadish, K. M. Smith, and R. Guilard, ‘The Porphyrin Handbook’, Vols. 15-20, Academic Press, San Diego, 2003; J. Mack and N. Kobayashi, Chem. Rev., 2011, 111, 281. CrossRef
2. T. Fukuda, S. Masuda, and N. Kobayashi, J. Am. Chem. Soc., 2007, 129, 5480; CrossRef M. Abel, S. Clair, O. Ourdjini, M. Mossoyan, and L. Porte, J. Am. Chem. Soc., 2011, 133, 1203; CrossRef X. Ding, L. Chen, Y. Honsho, X. Feng, O. Saengsawang, J. Guo, A. Saeki, S. Seki, S. Irle, S. Nagase, V. Parasuk, and D. Jiang, J. Am. Chem. Soc., 2011, 133, 14510. CrossRef
3. a) N. Kobayashi, H. Ogita, N. Nonaka, and E. A. Luk’yanets, Chem. Eur. J., 2003, 9, 5123; CrossRef b) T. Honda, T. Kojima, N. Kobayashi, and S. Fukuzumi, Angew. Chem. Int. Ed., 2011, 50, 2725; CrossRef c) N. Kobayashi, T. Furuyama, and K. Sayoh, J. Am. Chem. Soc., 2011, 133, 19642. CrossRef
4. J. L. Segura and N. Martín, Angew. Chem. Int. Ed., 2001, 40, 1372; CrossRef J. O. Jeppesen and J. Becher, Eur. J. Org. Chem., 2003, 3245; CrossRef M. Iyoda, M. Hasegawa, and Y. Miyake, Chem. Rev., 2004, 104, 5085; CrossRef N. Martín, L. Sánchez, M. Á. Herranz, B. Illescas, and D. M. Guldi, Acc. Chem. Res., 2007, 40, 1015. CrossRef
5. a) M. A. Blower, M. R. Bryce, and W. Devonport, Adv. Mater., 1996, 63; CrossRef b) M. J. Cook, G. Cooke, and A. Jafari-Fini, Chem. Commun., 1996, 1925; CrossRef c) C. Farren, C. A. Christensen, S. FitzGerald, M. R. Bryce, and A. Beeby, J. Org. Chem., 2002, 67, 9130; CrossRef d) J. Sly, P. Kasák, E. Gomar-Nadal, C. Rovira, L. Górriz, P. Thordarson, D. B. Amabilino, A. E. Rowan, and R. J. M. Nolte, Chem. Commun., 2005, 1255. CrossRef
6. C. Loosli, C. Jia, S.-X. Liu, M. Haas, M. Dias, E. Levillain, A. Neels, G. Labat, A. Hauser, and S. Decurtins, J. Org. Chem., 2005, 70, 4988; CrossRef C. A. Donders, S.-X. Liu, C. Loosli, L. Sanguinet, A. Neels, and S. Decurtins, Tetrahedron, 2006, 62, 3543. CrossRef
7. a) T. Kimura, D. Watanabe, and T. Namauo, Heterocycles, 2008, 76, 1023; CrossRef b) T. Kimura, T. Namauo, N. Takahashi, Y. Takaguchi, T. Hoshi, and N. Kobayashi, J. Porphyrins Phthalocyanines, 2011, 15, 547. CrossRef
8. a) M. Kimura, S. Otsuji, J. Takizawa, Y. Tatewaki, T. Fukawa, and H. Shirai, Chem. Lett., 2010, 39, 812; CrossRef b) T. Kimura, N. Takahashi, T. Tajima, and Y. Takaguchi, Heterocycles, 2012, 84, 333. CrossRef
9. a) U. Michelsen, H. Kliesch, G. Schnurpfeil, A. K. Sobbi, and D. Wöhrle, Photochem. Photobiol., 1996, 64, 694; CrossRef b) N. Kobayashi, R. Kondo, S. Nakajima, and T. Osa, J. Am. Chem. Soc., 1990, 112, 9640; CrossRef c) C. C. Leznoff, P. I. Svirskaya, B. Khouw, R. L. Cerny, P. Seymour, and A. B. P. Lever, J. Org. Chem., 1991, 56, 82. CrossRef
10. F. Wudl, G. M. Smith, and E. J. Hufnagel, J. Chem. Soc., Chem. Commun., 1970, 1453. CrossRef