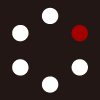
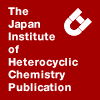
HETEROCYCLES
An International Journal for Reviews and Communications in Heterocyclic ChemistryWeb Edition ISSN: 1881-0942
Published online by The Japan Institute of Heterocyclic Chemistry
e-Journal
Full Text HTML
Received, 14th May, 2012, Accepted, 5th July, 2012, Published online, 13th July, 2012.
DOI: 10.3987/COM-12-S(N)17
■ SULFUR- AND N-ACYL CARBONYL OXYGEN-ASSISTED HETEROLYSIS OF THE C(=O)–S BOND IN EXCITED-STATE (Z)-N-ACYL-α-DEHYDRO(1-NAPHTHYL)ALANINE THIOESTERS
Yosuke Hosoi, Tetsutaro Igarashi, and Tadamitsu Sakurai*
Department of Material and Life Chemistry, Faculty of Engineering, Kanagawa University, 3-27-1 Rokkakubashi, Kanagawa-ku, Yokohama 221-8686, Japan
Abstract
Irradiation of the title thioesters (Z)-1 in nitrogen-saturated 1,2-dichloroethane at wavelengths greater than 280 nm was found to afford (Z)-4-(1-naphthylmethylene)-5(4H)-oxazolone derivatives (Z)-2, (E)-2, alkyl-substituted thiols 3, and (E)-1 without forming any thio-radical-derived products. An analysis of the substituent and solvent effects on the reactivity of 1 and the product distribution demonstrated that the heterolytic C(=O)–S bond cleavage products 2 and 3 are not derived from the long-lived acylium and thiolate ions but from the protonated oxazolone/thiolate ion pair. The latter intermediates were assumed to be formed by the sulfur-assisted nucleophilic addition of the N-acyl carbonyl oxygen to the thioester carbonyl carbon in the excited state, followed by the elimination of the thiolate ion from the resulting adducts.Organic photochemistry has continued to contribute to the development of efficient methods for synthesizing various heterocyclic compounds.1–3 In addition, owing to the fact that many of these compounds exhibit potent pharmacological activities, recent photochemical research is focusing primarily on the diverse photocyclization and photocycloaddition reactions of heteroatom-containing organic compounds including their asymmetric photoreactions.2,3 In the course of a systematic study of the photoinduced-electron-transfer (PET)-initiated reductive cyclization reactions of N-acyl-α-dehydroamino acid derivatives,4,5 we discovered that N-acyl-α-dehydroarylarylalanine alkyl esters in their excited states readily undergo one-electron reduction in the presence of an aliphatic amine to eventually afford the corresponding 4,5-dihydrooxazole derivatives with high selectivities.5 The high photostability of these products possessing two asymmetric carbons in the dihydrooxazole ring enabled usto explore the unprecedented asymmetric photocyclization initiated by PET.6 We found that without he amine, excited-state N-acyl-α-dehydroarylalanine alkyl and aryl esters undergo intriguing cyclizations to mainly form the corresponding isoquinoline and 4-arylmethylene-5(4H)-oxazolone derivatives, respectively.7,8 The observed contrasting cyclization mode was assumed to be due to a large difference in electronic distribution between the excited states of these two derivatives.
The replacement of the ester oxygen in α-dehydroarylalanine alkyl esters by sulfur is predicted to influence on the photoreactivity of these alkyl esters and the mode of the ester C(=O)–O bond cleavage. A comprehensive literature survey of the photochemical behavior of thioester derivatives revealed that the C(=O)–S bond in these derivatives has a strong tendency to undergo a homolytic cleavage reaction affording the corresponding acyl and thio radicals.9 Thus, we predict that the irradiation of N-acyl-α-dehydroarylalanine thioester will induce a new mode of cyclization. To elucidate the role played by the sulfur atom in the photochemical behavior of this kind of thioester derivative, N-acyl-α-dehydro(1-naphthyl)alanine alkyl thioesters 1a–d (Chart 1) were synthesized followed by an examination of their photoreactivity and photoproduct composition.
The starting α-dehydronaphthylalanine thioesters in the (Z)-configuration were prepared in 30–40% yields by a Knoevenagel-type condensation between 1-naphthaldehyde and N-acylglycine in acetic anhydride containing sodium acetate, followed by ring-opening reactions of the resulting (Z)-4-(1-naphthylmethylene)-5(4H)-oxazolone derivative, mediated by equimolar amounts of phenylmethanethiol [(Z)-1a and (Z)-1b], p-tolylmethanethiol [(Z)-1c], or 2-acetylaminoethanethiol [(Z)-1d] in dichloroethane containing triethylamine.10 The crude thioesters were purified by column chromatography on silica gel (230 mesh, Merck) using chloroform as an eluent. To isolate major photoproducts, a nitrogen-saturated 1,2-dichloroethane solution (250 mL) containing (Z)-1a (4.0 ´ 10–3 mol dm–3) was irradiated at wavelengths greater than 280 nm from a 400-W high-pressure Hg lamp for 3 h at room temperature. Preparative thin-layer chromatography of the resulting reaction mixture using silica gel (eluent: chloroform) led to the isolation of (Z)-4-(1-naphthylmethylene)-2-phenyl-5(4H)-oxazolone [(Z)-2a], (E)-2a, phenylmethanethiol (3a), and (E)-1a in 17, 19, 10, and 25% yields, respectively (Scheme 1).11
The 1H NMR spectral analysis of the above reaction mixture revealed the negligible formation of the expected isoquinoline derivative and dibenzyl disulfide. Moreover, control experiments showed that (Z)-1a in 1,2-dichloroethane did not undergo any reaction without irradiation, whereas (Z)-2a and 3a were isolated in 42 and 18% yields, respectively, when an acetonitrile solution of (Z)-1a (4.0 x 10–3 mol dm–3) was allowed to stand for 3 h in a dark place at room temperature. In addition to the previous finding,8 the above observations confirm that 2a and 3a detected in 1,2-dichloroethane are derived from the exclusive heterolytic cleavage of the C(=O)–S bond of the excited-state 1a in this much less polar solvent than acetonitrile. Since these photoproducts underwent negligible decompostion under the irradiation conditions tested, the progress of the observed photocyclization was monitored by 1H NMR spectroscopy, and the results
are shown in Table 1.12 The material balance analysis of the products, 2a and 3a, predicts the appearance of an equimolar mixture of 2a [(Z)-2a + (E)-2a] and 3a but as shown in Table 1, the latter product was formed in a much lower composition than predicted. The finding that (Z)-1a in deuterated 1,2-dichloroethane, irradiated under the same conditions, affords 2a and 3a in a nearly 1:1 composition ratio (Table 1) suggests that the removal of the solvent under reduced pressure causes a technical loss of the latter somewhat volatile product.
To propose a mechanism whereby (Z)-1 in its excited state is converted into the corresponding (Z)- and (E)-oxazolone and thiol derivatives via the exclusive heterolytic cleavage of the C(=O)–S bond, we explored substituent and solvent effects on the conversion (photoreactivitity) of the starting 1 and the 1-derived product composition. A summary of these effects is shown in Table 2.12 Clearly, the replacement of the benzoyl group in (Z)-1a by the acetyl group lowered its photoreactivity by a factor of approximately 2 [see (Z)-1b], whereas the p-methylbenzyl and acetylaminoethyl substituents attached to the sulfur atom of (Z)-1c and (Z)-1d, respectively, exerted only a minor effect on the reactivity of (Z)-1a. The former finding suggests the participation of N-acyl carbonyl oxygen in the rate-determining step for the photocyclization reaction of (Z)-1. On the other hand, our previous study showed that the photochemical conversion of N-acetyl-α-dehydroarylalanine aryl esters into the corresponding 5(4H)-oxazolone proceeds through the acylium/aryloxide ion-pair intermediate where the acylium ion is trapped by methanol as its methyl ester derivative.8 To confirm whether such a long-lived acylium ion intermediate is involved in the elimination/cyclization reaction of (Z)-1, (Z)-1a was chosen as a typical thioester derivative and irradiated in nitrogen-saturated 1,2-dichloroethane containing 10 vol% methanol. As can be seen from Table 2, there was negligible formation of the expected N-benzoyl-α-dehydro(1-naphthyl)alanine methyl ester. In addition, when a methanol solution of (Z)-1a (4.0 x 10–3 mol dm–3) was allowed to stand for 1 h in a dark place at room temperature, it was converted to (Z)-2a and 3a without affording any other products as in acetonitrile (conversion, 14%). The above observations are consistent with the mechanism shown in Scheme 2 where 2 and 3 are formed through the protonated oxazolone/thiolate ion-pair intermediates (Z)-I and (E)-I, which are not derived from the corresponding long-lived acylium and thiolate ions but directly from the reactions of (Z)-1 and (E)-1 in the excited state, respectively.
Prior to describing the formation mechanism of the ion pair I, we have to explain the origin of the (E)-isomer of 2. The data given in Table 2 show that the irradiation of (E)-1a as the starting thioester derivative affords (Z)-1a, (E)-1a, (Z)-2a, (E)-2a, and 3a in almost the same compositions as that of (Z)-1a, irrespective of irradiation time (0.5 and 3 h). This confirms a much more rapid interconversion between (Z)-1a and (E)-1a compared with the conversion of 1a to 2a. Furthermore, the observation of the photoisomerization of (Z)-2a and (E)-2a, along with the selective transformation of the ground-state (Z)-1a to (Z)-2a in acetonitrile, establishes that both (E)-1 and (Z)-2 serve as the precursors of the (E)-isomer of 2, as depicted in Scheme 2. Meanwhile, since the N-acyl carbonyl oxygen may function as a nucleophilic atom, the oxazolone ring is considered to be constructed by the addition of this oxygen to the carbonyl carbon in the excited state, followed by the elimination of the thiolate ion. Thus, we propose that thioester derivatives (Z)-1 and (E)-1 adopt sulfur-assisted conjugated structures (Z)-II* and (E)-II*, respectively, in the excited state, resulting in an acceleration of the nucleophilic addition of the N-acyl carbonyl oxygen (Scheme 3). The elimination of the thiolate ion from the adducts (Z)-III and (E)-III and subsequent proton transfer within the ion pairs (Z)-I and (E)-I afford (Z)-2 and (E)-2, respectively, along with 3.
If the presence of the N-acyl group in 1 is an indispensable prerequisite for the exclusive heterolysis of the C(=O)–S bond, we predict that the removal of this group will increase the contribution of the homolytic C(=O)–S bond cleavage mode. To verify our prediction, benzylthio trans-cinnamate (4) was synthesized followed by an examination of the 4-derived product distribution and composition in methanol. Since the cinnamate 4 in the ground state did not undergo any solvolytic reaction in this polar protic solvent, nitrogen-saturated methanol solutions of 4 (4.0 x 10–3 mol dm–3, 10 mL x 10) were irradiated in parallel for 30 min on a merry-go-round irradiation apparatus under the same conditions as above, and the irradiated solutions were combined and concentrated in vacuo to dryness (conversion ≈ 100%, 1H NMR spectral analysis). Column chromatography of the resulting residue with silica gel (eluent: hexane-chloroform) enabled us to isolate methyl trans-cinnamate (5), phenylmethanethiol (3a), methyl 3-benzylthio-3-phenylpropanoate (6), and dibenzyl disulfide (7) in 38, <1, 36, and 8% yields, respectively (Scheme 4).13 Since 6 was readily formed by the reaction of 5 with 3a,14 the advent of 5 and 6 is an indication of the intervention of a long-lived acylium ion intermediate derived from the heterolytic C(=O)–S bond cleavage. In contrast, 7 is a homolytic C(=O)–S bond cleavage product, and hence, its appearance
confirms the participation of a radical mechanism even in the photolysis in the polar protic solvent methanol, which is consistent with our prediction. Therefore, we conclude that the N-acyl group in (Z)-1 plays a decisive role in inducing the above-mentioned sulfur-assisted addition/elimination mechanism that enables the exclusive heterolysis of the C(=O)–S bond. The sulfur atom in thioester 1 is considered to assist the nucleophilic addition of the N-acyl carbonyl oxygen to the thioester carbonyl carbon by stabilizing the transition state for this addition reaction.
The mechanistic considerations described above led us to propose that the ability of the thioester 1 to induce the heterolytic cleavage of the C(=O)–S bond is much greater in the excited state than in the ground state. This means that the C(=O)–S bond in 1 undergoes an exclusive photoheterolysis even in polymer film having a low polarity. Accordingly, our previous finding that the generation of 1-naphthylmethylene-substituted oxazolone in polymer film enhances the refractive index of this film renders the photocyclization of 1 (affording the oxazolone 2 and the thiol 3) one of the good reaction systems for the photochemical control of the film refractive index.15
ACKNOWLEDGMENTS
This research was partially supported by a “Scientific Frontier Research Project” from the Ministry of Education, Culture, Sports, Science and Technology in Japan.
References
1. ‘Photochemistry of Heterocyclic Compounds,’ ed. by O. Buchardt, Wiley-Interscience, New York, 1976.
2. a) ‘Synthetic Organic Photochemistry,’ ed. by W. M. Horspool, Plenum, New York, 1984; CrossRef b) ‘Synthetic Organic Photochemistry,’ ed. by A. G. Griesbeck and J. Mattay, Marcel Dekker, New York, 2005; c) A. G. Griesbeck, N. Hoffmann, and K. Warzecha, Acc. Chem. Res., 2007, 40, 128. CrossRef
3. a) S. R. L. Everitt and Y. Inoue, ‘Organic Molecular Photochemistry,’ ed. by V. Ramamurthy and K. S. Schanze, Marcel Dekker, New York, 1999, pp. 71–130; b) Y. Inoue, ‘Chiral Photochemistry,’ ed. by Y. Inoue and V. Ramamurthy, Marcel Dekker, New York, 2004, pp. 129–177; CrossRef c) N. Hoffmann and J.-P. Pete, ‘Chiral Photochemistry,’ ed. by Y. Inoue and V. Ramamurthy, Marcel Dekker, New York, 2004, pp. 179–233; CrossRef d) A. Bauer, F. Westkamper, S. Grimme, and T. Bach, Nature, 2005, 436, 1139. CrossRef
4. a) K. Maekawa, T. Igarashi, K. Kubo, and T. Sakurai, Tetrahedron, 2001, 57, 5515; CrossRef b) T. Motohashi, K. Maekawa, K. Kubo, T. Igarashi, and T. Sakurai, Heterocycles, 2002, 57, 269; CrossRef c) K. Maekawa, H. Kajiwara, Y. Iseya, T. Igarashi, and T. Sakurai, Heterocycles, 2003, 60, 637; CrossRef d) K. Maekawa, A. Shinozuka, M. Naito, T. Igarashi, and T. Sakurai, Tetrahedron, 2004, 60, 10293; CrossRef e) K. Maekawa, K. Fujita, K. Iizuka, T. Igarashi, and T. Sakurai, Heterocycles, 2005, 65, 117. CrossRef
5. a) K. Maekawa, T. Sasaki, K. Kubo, T. Igarashi, and T. Sakurai, Tetrahedron Lett., 2004, 45, 3663; CrossRef b) K. Maekawa, N. Hishikawa, K. Kubo, T. Igarashi, and T. Sakurai, Tetrahedron, 2007, 63, 11267; CrossRef c) Y. Sato, A. Yoshida, T. Igarashi, and T. Sakurai, Heterocycles, 2010, 81, 997. CrossRef
6. a) Y. Sasaki, K. Maekawa, H. Watanabe, T. Matsumoto, K. Kubo, T. Igarashi, and T. Sakurai, Tetrahedron Lett., 2007, 48, 4765; CrossRef b) H. Watanabe, K. Maekawa, T. Igarashi, and T. Sakurai, Heterocycles, 2007, 74, 149; CrossRef c) Y. Sato, Y. Haruyama, T. Igarashi, and T. Sakurai, Heterocycles, 2010, 82, 603; CrossRef d) H. Yadai, Y. Sato, T. Igarashi, and T. Sakurai, Heterocycles, 2012, 84, 737. CrossRef
7. H. Hoshina, H. Tsuru, K. Kubo, T. Igarashi, and T. Sakurai, Heterocycles, 2000, 53, 2261. CrossRef
8. H. Hoshina, K. Nakayama, T. Igarashi, and T. Sakurai, Heterocycles, 2002, 57, 1239. CrossRef
9. a) K. Praefcke, Tetrahedron Lett., 1973, 973; CrossRef b) J. H. Penn and F. Liu, J. Org. Chem., 1994, 59, 2608; CrossRef c) M. Tada, T. Yoshihara, and K. Sugano, J. Chem. Soc., Perkin Trans. 1, 1995, 1941; CrossRef d) M. Takahashi, T. Fujita, S. Watanabe, and M. Sakamoto, J. Chem. Soc., Perkin Trans. 2, 1998, 487. CrossRef
10. Selected data for (Z)-1a: mp 157.0–158.0 °C (EtOAc-hexane); 1H NMR (500 MHz, CDCl3) δ = 4.31 (2H, s), 7.27 (1H, dd, J = 6.9, 6.9 Hz), 7.34 (2H, dd, J = 7.4, 8.0 Hz), 7.37–7.41(5H, m), 7.48–7.56 (3H, m), 7.55 (1H, s), 7.61 (1H, d, J = 7.4 Hz), 7.70 (2H, d, J = 7.4 Hz), 7.83 (1H, d, J = 8.0Hz), 7.86 (1H, d, J = 7.4 Hz), 7.99 (1H, s), 8.00 (1H, d, J = 8.0 Hz); 13C NMR (125 MHz, CDCl3) δ = 33.9, 124.2, 125.3, 126.4, 126.6, 127.0, 127.2, 127.4 (2C), 127.6, 128.5, 128.7 (2C), 128.9, 129.0 (2C), 129.1, 129.9, 130.3, 131.3, 132.2, 133.2, 133.5, 133.6, 137.0, 166.2, 190.1. Anal. Calcd for C27H21NO2S: C, 76.57; H, 5.00; N, 3.31. Found: C, 76.85; H, 4.71; N, 3.29.
11. Selected data for (Z)-2a: 1H NMR (500 MHz, CDCl3) δ = 7.55 (2H, dd, J = 7.4, 7.4 Hz), 7.56 (1H, dd, J = 8.0, 8.0 Hz), 7.62 (1H, dd, J = 7.4, 7.4 Hz), 7.64 (1H, dd, J = 7.4, 7.4 Hz), 7.65 (1H, dd, J = 7.4, 8.0 Hz), 7.90 (1H, d, J = 8.0 Hz), 7.97 (1H, d, J = 8.0 Hz), 8.13 (1H, s), 8.20 (2H, d, J = 7.4 Hz), 8.31 (1H, d, J = 8.0 Hz), 9.03 (1H, d, J = 7.4 Hz). These 1H NMR spectral data were in good agreement with those of a previously prepared sample.5b
Selected data for (E)-2a: 1H NMR (500 MHz, CDCl3) δ = 7.52–7.64 (6H, m), 7.91 (1H, d, J = 8.0 Hz), 7.96 (1H, d, J = 8.6 Hz), 8.12 (1H, d, J = 8.0 Hz), 8.13 (1H, d, J = 8.0 Hz), 8.14 (1H, d, J = 6.3 Hz), 8.32 (1H, s), 8.37 (1H, d, J = 6.9 Hz); 13C NMR (125 MHz, CDCl3) δ = 123.6, 125.3, 125.6, 126.4, 127.4, 128.1 (2C), 128.5, 128.6, 129.1 (2C), 129.2, 129.5, 132.0, 133.2, 133.6, 135.3, 136.4, 162.1, 164.7. ESI-TOF-MS m/z calcd for C20H13NO2: 300.1025 [M + H]+. Found: 300.1002.
Selected data for 3a: 1H NMR (500 MHz, CDCl3) δ = 1.75 (1H, t, J = 7.4 Hz), 3.74 (2H, d, J = 7.4 Hz), 7.22–7.25 (1H, m), 7.30–7.33 (4H, m). These 1H NMR spectral data were consistent with those of a commercially available authentic sample.
Selected data for (E)-1a: 1H NMR (500 MHz, CDCl3) δ = 3.95 (2H, s), 7.05 (2H, d, J = 7.4 Hz), 7.15–7.21 (3H, m), 7.36 (1H, dd, J = 7.4, 8.0 Hz), 7.37 (1H, d, J = 6.9 Hz), 7.48–7.52 (4H, m), 7.56 (1H, dd, J = 6.9, 7.4 Hz), 7.83 (1H, d, J = 8.0 Hz), 7.86–7.87 (1H, m), 7.90 (2H, d, J = 7.4 Hz), 8.03–8.04 (1H, m), 8.52 (1H, s), 8.73 (1H, s); 13C NMR (125 MHz, CDCl3) δ = 34.3, 125.0, 125.3, 125.4, 126.3, 126.6, 127.2 (2C), 127.3, 128.2, 128.4, 128.5 (2C), 128.8 (2C), 128.9, 129.0 (2C), 129.1, 131.9, 132.2, 133.6, 133.8, 134.5, 136.7, 166.0, 190.6. ESI-TOF-MS m/z calcd for C27H21NO2S: 446.1191 [M + Na]+. Found: 446.1141..
12. To monitor the progress of the reaction for (Z)-1a, nitrogen-saturated 1,2-dichloroethane solutions of this thioester (4.0 × 10–3 mol dm–3, 10 mL × 5) were irradiated in parallel using a merry-go-round irradiation apparatus (light source: 400-W high-pressure Hg lamp, filter: Pyrex glass) for a given period of time at room temperature. After irradiation, the solutions were concentrated in vacuo to dryness and the resulting residues were subjected to 1H NMR spectral analysis. The composition of the photoproducts was estimated on the basis of the area ratio of the signals characteristic of each product.
13. Selected data for trans-4: mp 70.0–71.0 °C (hexane-EtOAc); 1H NMR (500 MHz, CDCl3) δ = 4.26 (2H, s), 6.72 (1H, d, J = 16.0 Hz), 7.25 (1H, dd, J = 7.0, 7.0 Hz), 7.30 (2H, d, J = 8.0 Hz), 7.38 (2H, dd, J = 7.0, 8.0 Hz), 7.38–7.40 (3H, m), 7.53–7.55 (2H, m), 7.63 (1H, d, J = 16.0 Hz); 13C NMR (125 MHz, CDCl3) δ = 33.2, 124.6, 127.3, 128.4 (2C), 128.6 (2C), 128.8 (2C), 128.9 (2C), 130.6, 134.0, 137.6, 140.9, 189.1. Anal. Calcd for C16H14OS: C, 75.55; H, 5.55. Found: C, 75.27; H, 5.43.
Selected data for trans-5: 1H NMR (500 MHz, CDCl17) δ = 3.76 (3H, s), 6.42 (1H, d, J = 16.0 Hz), 7.33–7.34 (3H, m), 7.46–7.48 (2H, m), 7.68 (1H, d, J = 16.0 Hz)
Selected data for 6: 1H NMR (500 MHz, CDCl17) δ = 2.82 (1H, dd, J = 7.5, 12.5 Hz), 2.86 (1H, dd, J = 8.5, 12.5 Hz), 3.46 (1H, d, J = 14.0 Hz), 3.55 (1H, d, J =14.0 Hz), 3.57 (3H, s), 4.16 (1H, dd, J = 7.5, 8.5 Hz), 7.20–7.47 (10H, m); 13C NMR (125 MHz, CDCl3) δ = 35.6, 41.2, 44.8, 51.7, 126.7, 127.5, 127.8 (2C), 128.4 (2C), 128.5 (2C), 128.9 (2C), 137.7, 141.0, 170.9. ESI-TOF-MS m/z calcd for C17H18O2S: 309.0925 [M + Na]+. Found: 309.0883.
Selected data for 7: 1H NMR (500 MHz, CDCl3) δ = 3.60 (4H, s), 7.23–7.33 (10H, m).
1H NMR spectral data for trans-5 and 7 were consistent with those of the corresponding commercially available authentic samples.
14. A. F. Meindertsma, M. M. Pollard, B. L. Feringa, J. G, de Vries, and A. J. Minnaard, Tetrahedron: Asymmetry, 2007, 18, 2849. CrossRef
15. H. Nakajima, H. Komatsu, H. Iikura, T. Igarashi, and T. Sakurai, Polym. J., 2010, 42, 670. CrossRef