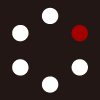
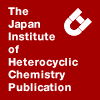
HETEROCYCLES
An International Journal for Reviews and Communications in Heterocyclic ChemistryWeb Edition ISSN: 1881-0942
Published online by The Japan Institute of Heterocyclic Chemistry
e-Journal
Full Text HTML
Received, 26th June, 2012, Accepted, 3rd August, 2012, Published online, 13th August, 2012.
DOI: 10.3987/COM-12-S(N)61
■ Synthesis of 2-Arylbenzimidazoles in Microfluidic Chip Reactor
Ming Lei,* Wei Li, Ruijun Hu, Wan Tian, Yanguang Wang, and Hong Zhang*
Department of Chemistry, Zhejiang University , 38 Zheda Road, Hangzhou, 310027, China
Abstract
A simple and efficient continuous flow synthesis of 2-arylbenzimidazoles from phenylenediamines and aromatic aldehydes in the presence of iodobenzene diacetate (IBD) has been developed by using a microfluidic chip reactor system. Various substituted 2-arylbenzimidazoles were obtained in high yields within 3 min under mild conditions. Compared with the classical batch systems, the present smooth procedure afforded highly selective due to the enhanced thermal and mass transfer.Benzimidazole is a very useful building block not only in the pharmaceutical science but also in several other fields such as agricultural, electronic, and polymer chemistry. Various substituted benzimidazole derivatives have been shown to exhibit versatile bioactivities and have found applications in diverse therapeutic and biological areas.1 The widespread interest in benzimidazole-containing structures has prompted extensive studies of benzimidazole derivative libraries and screen them for their various biological activities including antiulcer, antihypertensive, antiviral, antifungal, anticancer, anthelmintic, antiprotozoal, analgesic, anxiolytic, antiallergic, hormone antagonist, antidiabetic and antihistaminic, coagulant, anticoagulant, as well antioxidant activities.2
Combinatorial chemistry as a high-throughput synthetic technology has become a powerful tool for the discovery of new drugs and increasing interests have been drawn onto the application of microfluidic chips in this field.3 Automated microreactor-based (microfluidic chip) continuous flow systems have thepotential to greatly accelerate the production of small molecule libraries of drug-like structures (primarily heterocycles).4 In comparison with the classical batch systems, continuous microfluidic based reactors need less space, energy and reagents, and can offer many advantages including enhanced reagent mixing, small reaction volumes, precise parameter control, higher reproducibility, and enhanced selectivity in short reaction time but produce less wastes because of its short diffusion distance, large specific surface area, which could increase the thermal and mass transfer.
In general, there are two methods for the synthesis of 2-substituted benzimidazoles. One is the coupling of phenylenediamines and carboxylic acids5 or their derivatives (nitriles, imidates, or orthoesters),6 which often under strong acidic conditions, sometimes combined with very high temperatures or the using of microwave irradiation technique.6c The other method involves a two-step procedure that includes the oxidative cyclodehydrogenation of aniline Schiff’s bases, which are generated in situ from the condensation of phenylenediamines and aldehydes. In this transformation, various oxidants such as oxone,7 Pb(OAc)4,8 benzofuroxan,9 nitrobenzene,10 MnO2,11 2,3-dichloro-5,6-dicyanobenzoquinone (DDQ),12 oxone,13 1,4-benzoquinone,14 Na2S2O5,15 NaHSO3,16 and oxygen17 have been employed. In recent year, Wang18 and Du19 have developed a one-pot synthesis of 2-arylbenzimidazoles from phenylenediamines and aromatic aldehydes in the presence of iodobenzene diacetate (IBD) as an oxidant in which vigorous stirring is crucial for increasing the thermal and mass transfer. We envisaged that in continuous flow system this exothermic transformation would carry out more efficiently than in batch system by taking the advantage of excellent mixing. Our group had previously demonstrated the use of microfluidic chip reactors to achieve selective acylation of ferrocene.20 Compared with the classical batch systems, the method is rapid and highly selective due to the accurate temperature control and efficient mixing. More recently, we have developed a three-component 1,4-dipolar cycloaddition cascade approach for the synthesis of 2-(trifluoromethyl)-2H-[1,3]oxazino[2,3-a]isoquinolines in a continuous flow microreactor in which excellent mixing associated with microflow technique eliminated the formation of the corresponding byproduct and ensured the desired tandem transformation with very high selectivity and efficiency.21 As part of our ongoing research to develop new synthetic methods for the generation of heterocycle libraries based on microfluidic reactor, we herein report the microfluidic chip synthesis of 2-arylbenzimidazole derivatives.
Our reactor is a microfluidic chip fabricated in soda-lime glass with channels produced by standard photolithography and wet etching techniques.22 The internal geometry of the microfluidic chip is 500 µm × 100 µm × 1000 mm. The structure of the chip is illustrated in Scheme 1. PTFE tubing (inner diameter ID = 0.9 mm) was fitted to the inlet and outlet hole directly. The end of inlet tube was connected to a Harvard PHD 2000 syringe pump and the end of outlet tube was connected to a sample vial, which was used to collect the final solution.
We first investigated adapting the model reaction into a continuous flow process for the synthesis of 2-phenylbenzimidazole using o-phenylenediamine and benzaldehyde in the presence of IBD at different flow rates and temperatures. Preliminary test were performed at 20 °C. The ethanol solution of o-phenylenediamine (0.03 M) mixed with benzaldehyde (0.03 M) and the ethanol solution containing IBD (0.045 M) were introduced respectively through syringe pump at identical flow rates changed from 7.5 μL/min to 40 μL/min. The results are summarized in Table 1. When the flow rates were respectively set up at 7.5, 10, 12.5 and 15 μL/min, the yields of 2-arylbenzimidazole increased from 84% to 92% with the increase of flow rate and reached a peak at 15 L/min flow rate (Table 1, entries 1–4). While further increasing the flow rate led to decrease of the yields (Table 1, entries 5–8). At 30 °C and 35 °C, when flow rates set up at 10, 12.5, 15, 20 μL/min respectively, the reactions afforded the yields between 86% and 92% (Table 1, entries 2–5). In general, at 15 μL/min flow rate, the reaction could afford best yield.
Subsequently, a series of 2-arylbenzimidazole derivatives were synthesized to investigate the scope and limitation of this reaction using the optimized microfluidic chip procedure.23 As shown in Table 2, both electron-rich and electron-deficient benzaldehydes could afford 2-arylbenzimidazoles in good to excellent yields (Table 2, entries 1–10). Heteroaryl aldehydes such as 2-furyl and 2-thiophenecarboxaldehydes also gave good yields (Table 2, entries 11–12). In comparison, when perform the reaction in batch system, vigorous stirring is crucial for high yield. Otherwise the exothermic reaction would generate colored impurity. It is noteworthy that in microfluidic chip the reactions completed smoothly within 3 minutes. Moreover, by-product was not observed in all cases taking the advantage of enhanced thermal and mass transfer.
In conclusion, we have successfully developed a rapid and efficient process for the synthesis of 2-arylbenzimidazole derivatives using microfluidic chip as the reactor. Under the optimized conditions, a series of 2-arylbenzimidazole derivatives were synthesized in 85–93% isolated yields. This process was also applied to similar reactions using heteroaryl aldehydes as the substrates. Compared with the classical batch systems, the present smooth procedure afforded highly selective due to the excellent mixing. These advances will facilitate the rapid synthesis of these biologically important compounds. Further exploration of the reactivity features of this methodology as well as applications to the synthesis of complex molecules and the drug discovery process are in progress.
EXPERIMENTAL
All chemicals were reagent grade and used as purchased without further purification unless otherwise stated. Column chromatography was performed using silica gel 60 (300-400 mesh). Melting points were obtained on a YANACO micro melting point apparatus and are uncorrected. 1HNMR spectra were recorded in DMSO-d6 using TMS as an internal reference with a Bruker Avance-400 spectrometer operating at 400 MHz.
Typical Procedure for the Synthesis of 2-Arylbenzimidazoles in Microfluidic Chip.
2-Phenylbenzimidazole (1a). Ethanol solution of o-phenylenediamine (0.03 M) mixed with benzaldehyde (0.03 M) and the ethanol solution containing IBD (0.045 M) was transferred into separate gas-tight syringes. The syringes were placed in a Harvard PHD 2000 syringe pump which was set to deliver the reactants at identical flow rate of 15 μL/min. The output mixture was collected and then concentrated under vacuum. The residue was subjected to silica gel column chromatography with Pet–EA (1:4) as eluent to give the product; mp 290–292 °C (lit.,18 mp 289–291 °C); 1H NMR δ 12.93 (s, 1 H), 7.92 (d, J = 1.3 Hz, 2 H), 7.47-7.46 (m, 1 H), 7.24-7.19 (m, 4 H), 6.96-6.94 (m, 2 H).
2-(2-Methylphenyl)benzimidazole (1b): mp 199–201 °C (lit.,18 mp 199–200 °C); 1H NMR δ 12.81 (s, 1 H), 7.67–7.63 (m, 3 H), 7.37–7.27 (m, 5 H), 2.60 (s, 3 H).
2-(4-Methoxyphenyl)benzimidazole (1c): mp 223–225 °C (lit.,18 mp 224–226 °C); 1H NMR δ 12.72 (s, 1 H), 8.11 (d, J = 8.80 Hz, 2 H), 7.61 (d, J = 7.60 Hz, 1 H), 7.49 (d, J = 6.80 Hz, 1 H), 7.17-7.11 (m, 4 H), 3.84 (s, 3 H).
2-(4-Ethylphenyl)benzimidazole (1d): mp 143–145 °C (lit.,18 mp 143–145 °C); 1H NMR δ 12.80 (s, 1 H), 8.09 (d, J = 8.40 Hz, 2 H), 7.64 (d, J = 8.80 Hz, 1 H), 7.51 (d, J = 8.40 Hz, 1 H), 7.39 (d, J = 8.00 Hz, 2 H), 7.19-7.17 (m, 2 H), 2.68 (d, J = 7.60 Hz, 2 H), 1.23 (t, J = 7.80 Hz, 3 H).
2-(2-Chlorophenyl)benzimidazole (1e): mp 232–233 °C (lit.,18 mp 233–234 °C); 1H NMR δ 12.93 (s, 1 H), 8.44 (q, J = 3.10 Hz, 1 H), 7.71 (s, 2 H), 7.50 (t, J = 3.80 Hz, 1 H), 7.40-7.37 (m, 2 H), 7.33 (q, J = 3.06 Hz, 2 H).
2-(4-Chlorophenyl)benzimidazole (1f): mp 292–294 °C (lit.,18 mp 292–294 °C); 1H NMR δ 12.93 (s, 1 H), 8.02 (d, J = 8.45 Hz, 2 H), 7.67 (t, J = 2.88 Hz, 2 H), 7.43 (d, J = 8.40 Hz, 2 H), 7.31 (q, J = 3.05 Hz, 2 H).
2-(4-Bromophenyl)benzimidazole (1g): mp 299–301 °C (lit.,18 mp 299–300 °C); 1H NMR δ 12.98 (s, 1 H), 8.11 (d, J = 8.50 Hz, 2 H), 7.77 (d, J = 8.50 Hz, 2 H), 7.65 (s, 1 H), 7.54 (s, 1 H), 7.21 (s, 2 H).
2-(4-Fluorophenyl)benzimidazole (1h): mp 250–252 °C (lit.,18 mp 250–251 °C); 1H NMR δ 12.97 (s, 1 H), 8.04-8.02 (m, 2 H), 7.32-7.25 (m, 4 H), 7.20-7.18 (m, 2 H).
5-Methyl-2-phenylbenzimidazole (1i): mp 242–243 °C (lit.,18 mp 242–243 °C); 1H NMR δ 12.72 (s, 1 H), 8.12 (d, J = 7.51 Hz, 2 H), 7.48-7.42 (m, 4 H), 7.35 (s, 1 H), 7.01 (d, J = 8.10 Hz, 1 H), 2.40 (s, 3 H).
5-Methoxy-2-phenylbenzimidazole (1j): mp 143–145 °C (lit.,18 mp 143–145 °C); 1H NMR δ 12.72 (s, 1 H), 8.10 (d, J = 8.01 Hz, 2 H), 7.47-7.41 (m, 4 H), 7.10 (s, 1 H), 6.82 (dd, J = 8.72, 2.01 Hz, 1 H), 3.78 (s, 3 H).
2-(2-Furyl)benzimidazole (1k): mp 287–289 °C (lit.,18 mp 287–288 °C); 1H NMR δ 12.92 (s, 1 H), 7.94 (d, J = 1.20 Hz, 1 H), 7.55 (d, J = 1.50 Hz, 2H), 7.21–7.18 (m, 3 H), 6.74–6.72 (m, 1 H).
2-(2-Thienyl)benzimidazole (1l): mp 329–331 °C (lit.,18 mp 330–332 °C); 1H NMR δ 12.93 (s, 1 H), 7.82 (d, J = 2.6 Hz, 1 H), 7.73 (d, J = 3.2 Hz, 1 H), 7.58–7.55 (m, 2 H), 7.24–7.18 (m, 3 H).
ACKNOWLEDGEMENTS
Financial support of this work from National Science and Technology Support Program (No. 2012BAI13B06) is gratefully acknowledged.
References
1. B. Narasimhan, D. Sharma, and P. Kumar, Med. Chem. Res., 2012, 21, 269. CrossRef
2. (a) M. J. Tebbe, W. A. Spitzer, F. Victor, S. C. Miller, C. C. Lee, T. R. Sattelberg, E. McKinney, and C. J. Tang, J. Med. Chem., 1997, 40, 3937; CrossRef (b) T. Roth, M. L. Morningstar, P. L. Boyer, S. H. Hughes, R. W. Buckheit, and C. J. Michejda, J. Med. Chem., 1997, 40, 4199; CrossRef (c) A. R. Porcari, R. V. Devivar, L. S. Kucera, J. C. Drach, and L. B. Townsend, J. Med. Chem., 1998, 41, 1252; CrossRef (d) M. Tonelli, M. Simone, B. Tasso, F. Novelli, V. Boido, F. Sparatore, G. Paglietti, S. Pricl, G. Giliberti, S. Blois, C. Ibba, G. Sanna, R. Loddo, and P. La Colla, Bioorg. Med. Chem., 2010, 18, 2937; CrossRef (e) J. L. Wang, J. Zhang, Z. M. Zhou, Z. H. Li, W. Z. Xue, D. Xu, L, P. Hao, X, F, Han, F. Fei, T. Liu, and A. H. Liang, Eur. J. Med. Chem., 2012, 49, 183; CrossRef (f) S. C. Lee, D. Shin, J. M. Cho, S. Ro, and Y. G. Suh, Bioorg. Med. Chem. Lett., 2012, 22, 1891; CrossRef (g) R. R. Calvo, S. K. Meegalla, D. J. Parks, W. H. Parsons, S. K. Ballentine, M. L. Lubin, C. Schneider, R. W. Colburn, C. M. Flores, and M. R. Player, Bioorg. Med. Chem. Lett., 2012, 22, 1903. CrossRef
3. (a) L. Kang, B. G. Chung, R. A. Langer, and A. Khademhosseini, Drug Discovery Today, 2008, 13, 1; CrossRef (b) C. O. Kappe and D. Dallinger, Nature Rev. Drug Discovery, 2006, 5, 51; CrossRef (c) C. Wiles and P. Watts, Expert Opin. Drug Discovery, 2007, 11, 1487. CrossRef
4. (a) B. P. Mason, K. E. Price, J. L. Steinbacher, A. R. Bogdan, and D. T. McQuade, Chem. Rev., 2007, 107, 2300; CrossRef (b) I. R. Baxendale, J. J. Hayward, S. Lanners, S. V. Ley, and C. D. Smith, 'Microreactors in Organic Synthesis and Catalysis,' T. Wirth, Ed., Wiley-VCH, Weinheim, 2008; (c) W. Ehrfeld, V. Hessel, and H. Lowe, 'Microreactors: New Technology for Modern Chemistry,' Wiley, Weinheim, 2000; (d) C. M. Griffiths-Jones, M. D. Hopkin, D. Jönsson, S. V. Ley, D. J. Tapolczay, E. Vickerstaffe, and M. Ladlow, J. Comb. Chem., 2007, 9, 422. CrossRef
5. (a) M. R. Grimmet, 'Comprehensive Heterocyclic Chemistry,' Vol. 5, A. R. Katritzky and C. W. Rees, Eds., Pergamon Press, Oxford, 1984, 457; CrossRef (b) R. W. Middleton and D. G. Wibberley, J. Heterocycl. Chem., 1980, 17, 1757; CrossRef (c) T. Hisano, M. Ichikawa, K. Tsumoto, and M. Tasaki, Chem. Pharm. Bull., 1982, 30, 2996. CrossRef
6. (a) A. Czarny, W. D. Wilson, and D. W. Boykin, J. Heterocycl. Chem., 1996, 33, 1393; CrossRef (b) R. R. Tidwell, J. D. Geratz, O. Dann, G. Volz, D. Zeh, and H. Loewe, J. Med. Chem., 1978, 21, 613; CrossRef (c) K. Bourgrin, A. Loupy, and M. Soufiaoui, Tetrahedron, 1998, 54, 8055. CrossRef
7. F. F. Stephens and J. D. Bower, J. Chem. Soc., 1949, 2971. CrossRef
8. I. Bhatnagar and M. V. George, Tetrahedron, 1968, 24, 1293. CrossRef
9. H. Chikashita, S. Nishida, M. Miyazaki, Y.Morita, and K. Itoh, Bull. Chem. Soc. Jpn., 1987, 60, 737. CrossRef
10. B. Yadagiri and J. W. Lown, Synth. Commun., 1990, 20, 955. CrossRef
11. F. Patzold, F. Zeuner, T. H. Heyer, and H. J. Niclas, Synth. Commun., 1992, 22, 281. CrossRef
12. J. J. vanden Eynde, F. Delfosse, P. Lor, and Y. van Haverbeke, Tetrahedron, 1995, 51, 5813. CrossRef
13. R. L. Lombardy, F. A. Tanious, K. Ramachandran, R. R. Tidwell, and W. D. Wilson, J. Med. Chem., 1996, 39, 1452. CrossRef
14. E. Verner, B. A. Katz, J. R. Spencer, D. Allen, J. Hataye, W. Hruzewicz, H. C. Hui, A. Kolesnikov, Y. Li, C. Luong, A. Martelli, K. Radika, R. Rai, M. She, W. Shrader, P. A. Sprengeler, S. Trapp, J. Wang, W. B. Young, and R. L. Mackman, J. Med. Chem., 2001, 44, 2753. CrossRef
15. S. C. Austen and J. M. Kane, J. Heterocycl. Chem., 2001, 38, 979. CrossRef
16. P. L. Beaulieu, B. Haché, and E. von Moos, Synthesis, 2003, 1683. CrossRef
17. S. Lin and L. Yang, Tetrahedron Lett., 2005, 46, 4315. CrossRef
18. L. H. Du and Y. G. Wang, Synthesis, 2007, 675. CrossRef
19. L. H. Du and X. P. Luo, Synth. Commun., 2010, 40, 2880. CrossRef
20. R. J. Hu., M. Lei, H. S. Xiong, X. Mu, Y. G. Wang, and X. F. Yin, Tetrahedron Lett., 2008, 49, 387. CrossRef
21. M. Lei, W. Tian, R. J. Hu. W. Li, and H. Zhang, Synthesis, 2012, 44, 2519. CrossRef
22. J. Gao, X. F. Yin, and Z. L. Fang, Lab Chip, 2004, 4, 47 CrossRef