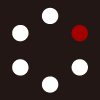
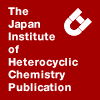
HETEROCYCLES
An International Journal for Reviews and Communications in Heterocyclic ChemistryWeb Edition ISSN: 1881-0942
Published online by The Japan Institute of Heterocyclic Chemistry
e-Journal
Full Text HTML
Received, 17th June, 2012, Accepted, 6th September, 2012, Published online, 21st September, 2012.
DOI: 10.3987/COM-12-S(N)35
■ Advances in Siloxane-Based Coupling Reactions: Application of Palladium-Mediated Allyl-Aryl Coupling to the Synthesis of Pancratistatin Derivatives. The Formal Total Synthesis of (±)-7-Deoxypancratistatin
Krupa H. Shukla and Philip DeShong*
Department of Chemistry and Biochemistry, University of Maryland, 7950 Baltimore Avenue, College Park, MD 20742, U.S.A.
Abstract
Palladium-mediated coupling of an allylic carbonate and an aryl siloxane has been applied to the formal total synthesis of 7-deoxypancratistatin and pancratistatin analogues. The key coupling reaction involved the use of a novel palladium olefin complex resulting in regio- and stereoselective arylation yielding a tetracyclic A-C ring intermediate. The observed regioselectivity of the coupling reaction was consistent with a model in which an unsymmetrical π-allyl palladium complex was formed. Coupling of a variety of substituted phenyl siloxane derivatives was achieved using the new Pd(0) system to provide access to novel pancratistatin derivatives.INTRODUCTION
The Amaryllidaceae alkaloids (+)-pancratistatin1 (1) and (+)-7-deoxypancratistatin2 (2) have attracted considerable attention in the synthetic community due to their potent antiviral and antitumor activity (Figure 1). Recently, Pandey and co-workers have shown that pancratistatin selectively induces apoptosis in various types of cancer cell lines (breast, colon, prostate, neuroblastoma, melanoma, and leukemia) at micromolar concentrations.3 In spite of their interesting biological profiles, (+)-pancratistatin and (+)-7-deoxypancratistatin have found limited clinical applications because of their low natural abundance. Therefore, there is a need to design a practical and scalable route for the preparation of multigram quantities of these antitumor alkaloids.
An obvious approach for the synthesis of pancratistatin (1) and 7-deoxypancratistatin (2) involves the formation of a bond between the aromatic ring (A ring) and a functionalized cyclohexane (C ring) (Scheme 1).4-13 The majority of the synthetic routes that have been reported to date employ the A-C coupling strategy; however, they are either too lengthy or low yielding for practical preparations of the natural products. The purpose of this study was to develop an efficient synthetic route to antitumor alkaloids pancratistatin (1) and 7-deoxypancratistatin (2) utilizing palladium-catalyzed allylic-aryl coupling methodology developed in our group,14 thus providing multigram quantities of these compounds for clinical evaluation. Denmark has reported the alternative allylic-aryl coupling reaction in which an aryl bromide is coupled to an allylic silonate.15
In order to establish the viability of the siloxane coupling methodology, diol 8, an analogue of 7-deoxypancratistatin was synthesized (Scheme 2). As previously reported, the key reaction in the synthesis of diol 8 was stereoselective construction of a carbon-carbon bond between the A and C rings via coupling of aryl siloxane 4 with allylic carbonate 3.16 The regio- and stereoselectivity of the coupling reaction was consistent with the formation of an aryl-π-allyl intermediate 5 that underwent reductive elimination to produce a mixture of urethanes 6 and 7 (6:7 = 1:1.6). We proposed that the poor regioselectivity of this coupling occurred due to formation of a “symmetrical” π-allyl palladium complex 5. In this instance, reductive elimination from complex 5 is equally probable onto either carbon 1 or 3, resulting in the formation of two regioisomers, 6 and 7 as outlined in Scheme 2. However, we anticipated that the coupling reaction of the allylic carbonate to provide the actual natural products, pancratistatin and 7-deoxypancratistatin, would show excellent regioselectivity due to the additional oxygen substituents (vide infra).
RESULTS AND DISCUSSION
Precursors for the coupling reaction in the (±)-7-deoxypancratistatin system were prepared as outlined in Schemes 3 and 4. Allylic carbonate 12 was synthesized from diene 917 through the cycloaddition of a nitroso dienophile (Scheme 3). This synthetic strategy is similar to the methodology employed by Martin,18 Hudlicky19 and Yan20 in the syntheses of narciclasine alkaloids. We utilized racemic diene 9 in this study, due to the ability to prepare it in large quantities. Hudlicky has previously reported the synthesis of (+)-enantiomer analogue of diene 9.21,22 The Diels-Alder reaction of diene 9 with the acyl nitroso moiety generated in situ, provided racemic hydroxamate 10.23 Reduction of the N-O bond generated allylic alcohol 11,24 and subsequent esterification of the alcohol with ethylchloroformate provided allylic carbonate 12.
Aryl siloxane 4 was synthesized from commercially available aryl bromide 13 according to a procedure previously reported by Manoso and DeShong (Scheme 4).25 Aryl bromide 13 was converted to the Grignard reagent, which was quenched with tetraethylorthosilicate to form aryl siloxane 4. This procedure is general for the preparation of aryl siloxane derivatives, and has been employed to synthesize aryl siloxanes with a variety of substituents.26
While the siloxane methodology was successfully applied to the synthesis of a (±)-7-deoxypancratistatin analogue 8 (Scheme 2), attempts to couple carbonate 12 and siloxane 4 under analogous conditions for the synthesis of (±)-7-deoxypancratistatin (2) failed to produce the desired adduct 16 (Scheme 5). To understand the causes of the failure of the coupling reaction, a detailed mechanistic study was undertaken.27 This mechanistic study of the siloxane coupling reaction indicated that the best Pd(0) system for the allyl-aryl cross-coupling reaction would have palladium bonded to sterically demanding, but weakly σ-bonding ligands. This set of ligand requirements is not found in the typical Pd(0) complexes employed for the coupling of aryl derivatives, since Pd(0) is typically stabilized by strong σ-donating ligand systems (phosphines). However, there are few Pd(0) complexes containing alkenes as the only ligands that possess these characteristics (Figure 2).28-39 The catalytic activity of this family of Pd(0)-olefin complexes has not been widely investigated. A few of these Pd(0)-olefin complexes have been employed as catalysts in Suzuki-Miyaura and Mizoroki-Heck coupling reactions.40-42 Also, Fairlamb has recently reported the use of ion-tagged π-acidic alkene ligands in the presence of palladium precatalyst to catalyze allyl-aryl cross coupling reactions in an ionic liquid.43 Accordingly, we surveyed a series of Pd(0)-olefin complexes for the allyl-aryl coupling reaction and found that tri-olefin Pd-complex 1431,32 was effective for this particular coupling reaction. The coupling of allylic carbonate 12 and aryl siloxane 4 in the presence of 50 mol% 14 produced the desired coupling product 16 in 35% yield (Scheme 5). Also, rearranged allyl alcohol, generated from rearrangement and hydrolysis of allylic carbonate 12, was isolated (18-32%) under various reaction conditions. The decomposition of Pd-complex 14 to palladium black may also have contributed to lower product yield.
As anticipated utilizing the model developed previously, the Pd(COD)(NQ) 14 mediated coupling reaction of allylic carbonate 12 exclusively produced regioisomer 16. Due to steric bulk arising from the acetonide moiety, an “unsymmetrical” π-allyl palladium complex 15 is formed, where palladium resides further from the acetonide group (Scheme 5).44 The regiochemistry of carbamate 16 was established using 1H-1H COSY analysis (see Supporting Information). The formation of the carbamate 16 via palladium-mediated allylic-arylation constituted the formal total synthesis of natural product (±)-7-deoxypancratistatin (2). The spectra of carbamate 16 were identical to those reported by Hudlicky in his synthesis of (+)-7-deoxypancratistatin.5
According to Hudlicky's synthesis of (+)-7-deoxypancratistatin, the key steps after the formation of carbamate 16 include the installation of a trans-diol in allylic-alcohol 17 via directed epoxidation, and a Friedel-Crafts acylation to generate the B ring (Scheme 6). Following Hudlicky's procedure, the carbamate 16 was deprotected to generate allylic alcohol 17. Epoxidation of allylic alcohol 17 was performed in benzene as reported by Hudlicky.5 However, the poor solubility of diol 17 in benzene led to longer reaction times and extensive decomposition of the diol. Using acetonitrile as the solvent improved solubility of diol 17, and NMR analysis of the crude product indicated the formation of a diastereomeric mixture of epoxides 18 in which the β-epoxide predominated (traces of α-epoxide observed by 1H NMR). This result was analogous to the results reported by Hudlicky who had reported the conversion β-epoxide to 7-deoxypancratistatin.5 Accordingly, at this point the formal total synthesis of 7-deoxypancratistatin was complete, and the focus of our remaining efforts were to demonstrate that this coupling strategy could be utilized for the preparation of pancratistatin-related compounds for potential biological evaluation.
The palladium-mediated allylic-arylation approach reported above involved coupling of allylic carbonate 12 with aryl siloxane 4 (Scheme 5). Furthermore, the coupling reaction can be performed at ambient temperature without affecting the yield. Though the yield for the key reaction was modest, the ability to couple using readily available precursors is a distinct advantage over the allylic-arylation methodology reported by Trost4 and Hudlicky5 (Scheme 1). Also, due to the ease of preparation of coupling partners, the siloxane coupling methodology is particularly well suited for the commercial synthesis of these natural products and subsequent derivatives as outlined in the Scheme 7. Aryl siloxane coupling with allylic carbonate 12 produced the coupling products (20, 22, 24, and 26), as summarized in the Table 1. These coupling products can be converted to a variety of pancratistatin derivatives (Scheme 7).5
This study demonstrated that novel tri-olefin Pd-complex 14 (Figure 2) could be employed in the synthesis of (±)-7-deoxypancratistatin (2). Extensive optimization studies were performed to identify the appropriate palladium-olefin complex. During these studies, we noticed differences in the reactivity as well as stability of metal-olefin complexes when the electronics and sterics of the olefin ligand of complex 14 were altered. Subtle changes, such as inclusion of an aromatic ring, modification of the electronic nature of the diene, as well as type of electron withdrawing olefin, strongly affected yields of the allyl-aryl coupling reaction. While the yield of the coupled product obtained under these conditions was modest, this is a marked improvement over the coupling reactions employing traditional Pd(0) catalysts such as Pd-dba and Pd-phosphine complexes in which minimal (< 5%) coupling was observed. Future studies shall focus on the development of novel Pd-olefin complexes 27 (Figure 3) and application of these complexes in a variety of coupling reactions. Pd(0) complexes such as 14 and its congeners were reported in 198331, however, the utilization of these complexes in Pd(0)-mediated allyl-aryl couplings has not been reported previously. In our systems, complex 14 has been shown to be the only Pd(0) complex capable of actuating the allylic-aryl coupling. Accordingly, these complexes, such as 14 and 27, may prove to be valuable additions to the arsenal of coupling methodology for systems in which the more traditional Pd(0) catalysts such as Pdx(dba)y are ineffective. We shall report on the use of complexes 27 in coupling reactions in due course.
In conclusion, palladium-mediated allylic-arylation has been applied to the formal total synthesis of (±)-7-deoxypancratistatin (2) via Hudlicky's intermediate 16 (Schemes 5 and 6). The key reaction of this approach was the coupling of an allylic carbonate 12 and an aryl siloxane 4 in the presence of a novel Pd(0) olefin complex, Pd(COD)(NQ) 14, which resulted in stereoselective arylation to form a single constitutional isomer. This coupling approach provided yields that were comparable to the allyl-aryl coupling methodology reported by Trost4 and Hudlicky5; however, this protocol is an improvement on the previously reported methods due to the ease of preparation as well as stability of the coupling precursors 12 and 4. Additionally, this coupling strategy can be utilized to prepare a variety of pancratistatin derivatives. Future goals are to further develop olefin based palladium complexes to optimize the key reaction.
EXPERIMENTAL
All reactions were run under an atmosphere of argon unless otherwise noted. Glassware used in the reactions was dried for a minimum of 12 h in an oven at 120 °C. Tetrahydrofuran was distilled from sodium/benzophenone ketyl, while methylene chloride, pyridine and dimethylformamide were distilled from calcium hydride. Infrared spectra were recorded on a Nicolet 560 FT-IR spectrophotometer. Samples used for obtaining infrared spectra were either dissolved in carbon tetrachloride or taken neat. IR band positions are reported in reciprocal centimeters (cm-1) and relative intensities are listed as br (broad), s (strong), m (medium), or w (weak). Nuclear magnetic resonance (1H, 13C NMR) spectra were recorded on a 400 or 500 MHz spectrometer. Chemical shifts are reported in parts per million (δ) and coupling (J values) are reported in hertz (Hz). Spin multiplicities are indicated by the following symbols: s (singlet), d (doublet), t (triplet), q (quartet), m (multiplet), br s (broad singlet) and br d (broad doublet). Low resolution mass spectrometry (LRMS) and high resolution mass spectrometry (HRMS) were obtained on a JEOL SX-02A instrument.
2,2-Dimethyl-3a,7a-dihydrobenzo[d][1,3]dioxole 917 and Pd(COD)(NQ) 1431,32 were prepared by previously reported procedures. Triethoxy(phenyl)silane 21 was purchased from Sigma-Aldrich. Benzo[d][1,3]dioxol-5-yltriethoxysilane 4,16 (4-methylphenyl)triethoxysilane 2326 and (4-chlorophenyl)triethoxysilane 2525 were prepared from commercially available 5-bromo-1,3-benzodioxole, p-bromotoluene and p-chloroiodobenzene respectively according to the procedure reported by the DeShong group.
Hydroxamate (10): To 32.8 g (75.9 mmol, 1.40 equiv.) of NBu4+IO4- (tetrabutylammonium periodate) under argon was added chloroform (110 mL) and DMF (55.0 mL). The reaction mixture was cooled to 0 °C and then 8.25 g (54.2 mmol, 1.00 equiv.) of diene 9 was added. Finally, 4.93 g (54.2 mmol, 1.00 equiv.) of hydroxamic acid (HONHCO2Me) dissolved in chloroform (55.0 mL) and DMF (18.0 mL) was added via addition funnel. The reaction mixture was stirred at 0 °C to room temperature for 3 days. The product was extracted with Et2O (5 × 100 mL), washed with H2O (100 mL), dried over MgSO4, concentrated in vacuo to give the crude hydroxamate 10 as an orange oil. Flash column chromatography on silica gel (30% EtOAc/70% hexane, Rf = 0.11) gave 4.06 g (31%) of hydroxamate 10 as a light yellow solid; mp 146-148 °C; IR (CCl4) 3087 (w), 2999 (m), 2985 (m), 2935 (m), 1719 (s), 1440 (s), 1379 (s), 1336 (s), 1250 (s), 1215 (s) cm-1; 1H NMR (400 MHz, CDCl3) δ 6.45-6.35 (m, 2H), 5.03-5.00 (m, 1H), 4.87-4.84 (m, 1H), 4.53-4.46 (m, 2H), 3.70 (s, 3H), 1.27 (s, 3H), 1.26 (s, 3H); 13C NMR (100 MHz, CDCl3) δ 158.4, 130.6, 129.4, 110.9, 73.0, 72.5, 71.3, 53.6, 52.8, 25.5, 25.3; HRMS (ESI) calcd for C11H16O5N (M+H)+ 242.1029, found 242.1016.
Methyl 7-hydroxy-2,2-dimethyl-3a,4,7,7a-tetrahydrobenzo[d][1,3]dioxol-4-ylcarbamate (11): To 3.77 g (15.6 mmol, 1.00 equiv.) of the hydroxamate 10 dissolved in 160 mL acetonitrile and 8.00 mL distilled water was added 4.96 g (18.8 mmol, 1.20 equiv.) of molybdenum hexacarbonyl (Mo(CO)6). The reaction mixture was refluxed at 85 °C for 48 h. The black-brown reaction mixture was vacuum filtered through celite and the filtrate was concentrated in vacuo to give crude alcohol-carbamate 11 as brown solid. Flash column chromatography on silica gel (75% EtOAc/25% hexane, Rf = 0.49) gave 2.98 g (79%) of allylic alcohol 11 as a white solid; mp 86-88 °C; IR (CCl4) 3617 (m), 3449 (m), 3414 (w), 3049 (w), 2995 (m), 2942 (m), 2909 (m), 1730 (s), 1551 (m) cm-1; 1H NMR (400 MHz, CDCl3) δ 5.91-5.88 (m, 1H), 5.78-5.74 (m, 1H), 5.4 (br d, J = 8 Hz, 1H), 4.21-4.18 (m, 3H), 4.08-4.06 (m, 1H), 3.7 (s, 3H), 3.1 (br s, 1H), 1.4 (s, 3H), 1.3 (s, 3H); 13C NMR (100 MHz, CDCl3) δ 156.6, 131.1, 129.7, 109.1, 79.2, 76.8, 68.9, 52.2, 51.1, 26.9, 24.6; HRMS (ESI) calcd for C11H18O5N (M+H)+ 244.1185, found 244.1180. 1H NMR spectrum was identical to the data previously reported.19
Methyl 7-(ethoxycarbonyloxy)-2,2-dimethyl-3a,4,7,7a-tetrahydrobenzo[d][1,3]dioxol-4-ylcarbamate (12): To 2.95 g (12.1 mmol, 1.00 equiv.) of allylic alcohol 11 in 40.0 mL anhydrous CH2Cl2 and 1.47 mL (18.2 mmol, 1.50 equiv.) anhydrous pyridine was added 1.80 mL (18.2 mmol, 1.50 equiv.) of ethyl chloroformate dropwise via syringe under argon. The reaction was allowed to stir at room temperature for 7 days. Additional 1.50 equiv. of pyridine and 1.50 equiv. of ethyl chloroformate were added and reaction mixture was stirred for 24 h. The reaction mixture was extracted with CH2Cl2 (5 × 50 mL), washed with H2O (50 mL), dried over MgSO4 and concentrated in vacuo. Flash chromatography on silica gel (30% EtOAc/70% hexane, Rf = 0.33) afforded 3.27 g (86%) of the allylic carbonate 12 as a off-white solid; mp 63-65 °C; IR (CCl4) 3442 (m), 2992 (m), 2956 (m), 2906 (m), 1751 (s), 1733 (s), 1554 (m), 1508 (s) cm-1; 1H NMR (400 MHz, CDCl3) δ 5.86-5.80 (m, 2H), 5.2 (br s, 1H), 5.1 (s, 1H), 4.30-4.27 (m, 1H), 4.19-4.14 (q, J = 8 Hz, 4H), 3.6 (s, 3H), 1.4 (s, 3H), 1.28-1.24 (m, 6H); 13C NMR (100 MHz, CDCl3) δ 156.4, 154.2, 131.3, 127.0, 109.2, 75.9, 75.8, 73.9, 64.3, 52.1, 50.3, 26.8, 24.7, 14.1; HRMS (ESI) calcd for C14H22O7N (M+H)+ 316.1396, found 316.1383.
Triethoxy(7-methoxybenzo[d][1,3]dioxol-5-yl)silane (19): Aryl siloxane 19 was prepared from the corresponding aryl bromide, which was prepared according to the procedure of Magnus and Sehbat.6 A solution of 1.5 g (6.5 mmol) of aryl bromide,6 0.24 g (9.8 mmol) of fresh magnesium turnings and one crystal of iodine in 15 mL of dry THF was refluxed under an argon atmosphere for 2 h. The reaction mixture was cooled to 25 °C and then added dropwise via cannula to a solution of tetraethyl orthosilicate (4.1 g, 20 mmmol) in 5.0 mL of THF. The reaction mixture was stirred for 1 h at 25 °C, then quenched by the addition of 5.0 mL of ethanol and concentrated in vacuo. The crude reaction mixture was then partitioned between 50 mL of water and 4 × 50 mL of Et2O, and the combined organic layers were dried over Na2SO4 and concentrated in vacuo. Purification of the residue by flash chromatography (5% EtOAc/95% hexane, Rf = 0.25) gave 1.0 g (50%) of siloxane 19 as a colorless oil; IR (CCl4) 2975 (m), 2926 (m), 2885 (m), 1622 (m), 1504 (m), 1412 (m), 1114 (s) 1082 (s) cm-1; 1H NMR (400 MHz, CDCl3) δ 6.84 (s, 1H), 6.8 (s, 1H), 6.0 (s, 2H), 3.9 (s, 3H), 3.8 (q, J = 7 Hz, 6H), 1.2 (t, J = 7 Hz, 9H); 13C NMR (100 MHz, CDCl3) δ 148.8, 143.9, 137.3, 124.4, 114.1, 108.0, 101.3, 58.7, 56.5, 18.2; LRMS (FAB) 315 ((M+1), 47), 314 ((M+), 100), 313 (44), 269 (39), 165 (20); HRMS (FAB) calcd for C14H22O6Si (M+) 314.1186, found 314.1198.
Methyl 2,2-dimethyl-3a,4,5,7a-tetrahydro-5,5'-bibenzo[d][1,3]dioxol-4-ylcarbamate (16): To 462 mg (1.63 mmol, 2.00 equiv.) of aryl siloxane 4 and 256 mg (0.813 mmol, 1.00 equiv.) of allylic carbonate 12 dissolved in 15.0 mL anhydrous THF was added 1.63 mL (1.63 mmol, 2.00 equiv.) TBAF under argon. This was followed by addition of 152 mg (0.407 mmol, 0.500 equiv.) Pd(COD)(NQ). The reaction mixture was heated at 55 °C for 24 h. The reaction was then quenched by addition of 30.0 mL H2O. The product was extracted with 3 × 50 mL CH2Cl2 and washed with H2O. The combined organic extracts were dried over MgSO4, filtered and concentrated in vacuo to give brown oil. Flash column chromatography on silica gel (30% EtOAc/70% hexane, Rf = 0.17) gave 98 mg (35%) of carbamate 16 (racemic) as off-white solid, mp 177-179 °C (hexane-EtOAc); IR (CCl4) 3467 (w), 3442 (w), 3042 (w), 2995 (w), 2881 (w), 1730 (s), 1504 (s), 1486 (s), 1250 (s) cm-1; 1H NMR (500 MHz, (CD3)2CO) δ 6.8 (d, J = 8 Hz, 1H), 6.68-6.65 (m, 2H), 6.2 (br d, J = 8 Hz, 1H), 5.97-5.93 (m, 3H), 5.9 (d, J = 10 Hz, 1H), 4.7 (t, J = 5 Hz, 1H), 4.25-4.22 (m, 1H), 3.6 (q, J = 10 Hz, 1H), 3.5 (br d, J = 10 Hz, 1H), 3.4 (s, 3H), 1.5 (s, 3H), 1.3 (s, 3H); 13C NMR (125 MHz, (CD3)2CO) δ 157.4, 148.6, 147.4, 136.9, 136.6, 124.8, 122.5, 109.9, 109.4, 108.8, 101.9, 77.9, 73.3, 56.9, 51.6, 47.1, 28.7, 26.6; HRMS (ESI) calcd for C18H22O6N (M+H)+ 348.1447, found 348.1440. 1H and 13C NMR spectra of carbamate 16 in CDCl3 are identical with that reported by Hudlicky.5 The regiochemistry of carbamate 16 was established using 1H-1H COSY (500 MHz, (CD3)2CO) (see Supporting Information).
Methyl 7'-methoxy-2,2-dimethyl-3a,4,5,7a-tetrahydro-5,5'-bibenzo[d][1,3]dioxol-4-ylcarbamate (20): Carbamate 20 was prepared from allylic carbonate 12 as described for the preparation of carbamate 16 except aryl siloxane 19 was used in place of aryl siloxane 4; white solid (30% yield); mp 164-165 °C (pentane-EtOAc); IR (Neat) 3292 (w), 2995 (w), 2949 (w), 2906 (w), 1697 (s), 1558 (s), 1508 (m), 1079 (s), 1043 (s) cm-1; 1H NMR (500 MHz, (CD3)2CO) δ 6.5 (d, J = 2 Hz, 1H), 6.4 (d, J = 2 Hz, 1H), 6.2 (br d, J = 8 Hz, 1H), 5.9 (m, 3H), 5.88 (d, J = 10 Hz, 1H), 4.7 (br s, 1H), 4.25-4.24 (m, 1H), 3.84 (s, 3H), 3.6 (q, J = 10 Hz, 1H), 3.47-3.44 (m, 4H), 1.5 (s, 3H), 1.3 (s, 3H); 13C NMR (125 MHz, (CD3)2CO) δ 157.5, 149.9, 144.5, 137.3, 136.8, 135.0, 124.8, 109.9, 109.2, 103.0, 102.1, 77.9, 73.4, 57.0, 56.7, 51.7, 47.3, 28.7, 26.5; HRMS (ESI) calcd for C19H24O7N (M+H)+ 378.1553, found 378.1550.
Methyl 2,2-dimethyl-5-phenyl-3a,4,5,7a-tetrahydrobenzo[d][1,3]dioxol-4-ylcarbamate (22): Carbamate 22 was prepared from allylic carbonate 12 as described for the preparation of carbamate 16 except aryl siloxane 21 was used in place of aryl siloxane 4; off-white solid (38% yield); mp 148-150 °C (hexane-EtOAc); IR (CCl4) 3469 (w), 3448 (w), 2987 (w), 1730 (s), 1507 (s), 1246 (s), 1221 (s) cm-1; 1H NMR (400 MHz, (CD3)2CO) δ 7.30-7.27 (m, 2H), 7.22-7.18 (m, 3H), 6.2 (br d, J = 8 Hz, 1H), 5.99-5.95 (m, 1H), 5.9 (d, J = 10 Hz, 1H), 4.7 (t, J = 5 Hz, 1H), 4.3 (m, 1H), 3.7 (q, J = 10 Hz, 1H), 3.5 (br d, J = 10 Hz, 1H), 3.4 (s, 3H), 1.5 (s, 3H), 1.3 (s, 3H); 13C NMR (100 MHz, (CD3)2CO) δ 157.3, 142.7, 136.8, 129.3, 129.1, 127.5, 124.8, 109.8, 77.9, 73.3, 56.7, 51.6, 47.4, 28.7, 26.5; HRMS (ESI) calcd for C17H22O4N (M+H)+ 304.1549, found 304.1563.
Methyl 2,2-dimethyl-5-p-tolyl-3a,4,5,7a-tetrahydrobenzo[d][1,3]dioxol-4-ylcarbamate (24): Carbamate 24 was prepared from allylic carbonate 12 as described for the preparation of carbamate 16 except aryl siloxane 23 was used in place of aryl siloxane 4; white solid (16% yield); mp 164-166 °C (hexane-EtOAc); IR (CCl4) 3467 (w), 3446 (w), 2991 (w), 2957 (w), 2930 (w), 2868 (w), 1730 (s), 1516 (s), 1380 (w), 1370 (w), 1241 (s), 1217 (s) cm-1; 1H NMR (400 MHz, (CD3)2CO) δ 7.09, 7.06 (ABq, JAB = 8 Hz, 4H), 6.2 (br d, J = 8 Hz, 1H), 6.0 (d, J = 10 Hz, 1H), 5.8 (d, J = 10 Hz, 1H), 4.68-4.67 (m, 1H), 4.27-4.23 (m, 1H), 3.7 (q, J = 10 Hz, 1H), 3.50-3.47 (m, 1H), 3.4 (s, 3H), 2.3 (s, 3H), 1.5 (s, 3H), 1.3 (s, 3H); 13C NMR (125 MHz, (CD3)2CO) δ 157.4, 139.7, 137.1, 136.9, 129.8, 129.2, 124.7, 109.9, 78.0, 73.4, 56.8, 51.6, 47.0, 28.7, 26.6, 21.1; HRMS (ESI) calcd for C18H24O4N (M+H)+ 318.1705, found 318.1711.
Methyl 5-(4-chlorophenyl)-2,2-dimethyl-3a,4,5,7a-tetrahydrobenzo[d][1,3]dioxol-4-ylcarbamate (26): Carbamate 26 was prepared from allylic carbonate 12 as described for the preparation of carbamate 16 except aryl siloxane 25 was used in place of aryl siloxane 4; white solid (38% yield); mp 202-204 °C (pentane-Et2O); IR (Neat) 3281 (w), 3099 (w), 3027 (w), 2985 (w), 1694 (s), 1558 (s), 1254 (s), 1211 (s), 1079 (s), 1047 (s) cm-1; 1H NMR (400 MHz, (CD3)2CO) δ 7.3 (d, J = 8 Hz, 1H), 7.2 (d, J = 8 Hz, 1H), 6.3 (br d, J = 8 Hz, 1H), 6.02-5.98 (m, 1H), 5.9 (d, J = 10 Hz, 1H), 4.7 (t, J = 5 Hz, 1H), 4.27-4.23 (m, 1H), 3.7 (q, J = 10 Hz, 1H), 3.55-3.53 (m, 1H), 3.4 (s, 3H), 1.4 (s, 3H), 1.3 (s, 3H); 13C NMR (125 MHz, (CD3)2CO) δ 157.4, 141.8, 136.1, 132.9, 131.1, 129.2, 125.4, 110.0, 77.7, 73.3, 56.8, 51.7, 47.0, 28.7, 26.5; HRMS (ESI) calcd for C17H21O4NCl (M+H)+ 338.1159, found 338.1140.
ACKNOWLEDGEMENT
The generous financial support of the National Institutes of Health, National Cancer Institute (CA 82169) and the University of Maryland is acknowledged. We thank Dr. Yiu-Fai Lam and Dr. Yue Li (University of Maryland) for their assistance obtaining NMR and mass spectral data, respectively. The support of the National Science Foundation for purchase of NMR instrumentation (CHE0615049) is acknowledged. We are also grateful to Dr. Tomáš Hudlický, Brock University, Canada for providing spectral data and experimental procedures for intermediates in the pancratistatin series. We also thank Frederick Emil Nytko, III (University of Maryland) for his assistance in reviewing this manuscript.
References
1. (a) G. R. Pettit, V. Gaddamidi, and G. M. Cragg, J. Nat. Prod., 1984, 47, 1018; CrossRef (b) G. R. Pettit, V. Gaddamidi, G. M. Cragg, D. L. Herald, and Y. Sagawa, J. Chem. Soc., Chem. Commun., 1984, 1693; CrossRef (c) G. R. Pettit, V. Gaddamidi, D. L. Herald, S. B. Singh, G. M. Cragg, J. M. Schmidt, F. E. Boettner, M. Williams, and Y. Sagawa, J. Nat. Prod., 1986, 49, 995. CrossRef
2. (a) B. Gabrielsen, T. P. Monath, J. W. Huggins, D. F. Kefauver, G. R. Pettit, G. Groszek, M. Hollingshead, J. J. Kirsi, W. M. Shannon, E. M. Schubert, J. DaRe, B. Ugarkar, M. A. Ussery, and M. J. Phelan, J. Nat. Prod., 1992, 55, 1569; CrossRef (b) S. Ghosal, S. Singh, Y. Kumar, and R. S. Srivastava, Phytochemistry, 1989, 28, 611. CrossRef
3. (a) C. Griffin, A. Karnik, J. McNulty, and S. Pandey, Mol. Cancer Ther., 2011, 10, 57; CrossRef (b) S. Chatterjee, J. McNulty, and S. Pandey, Melanoma Res., 2011, 21, 1; CrossRef (c) C. Griffin, C. Hamm, J. McNulty, and S. Pandey, Cancer Cell Int., 2010, 10, 6; CrossRef (d) S. Pandey, J. Kekre, J. Naderi, and J. McNulty, Artif. Cells, Blood Substitutes, Biotechnol., 2005, 33, 279; (e) C. Griffin, J. McNulty, C. Hamm, and S. Pandey, In: Cell Apoptosis Research Trends, ed. by C. V. Zhang, Nova Science Publishers, Inc., NY, USA, 2007, pp. 93-109.
4. B. M. Trost and S. R. Pulley, J. Am. Chem. Soc., 1995, 117, 10143. CrossRef
5. T. Hudlicky, X. Tian, K. Königsberger, R. Maurya, J. Rouden, and F. Boreas, J. Am. Chem. Soc., 1996, 118, 10752 and references cited therein. CrossRef
6. P. Magnus and I. K. Sebhat, Tetrahedron, 1998, 54, 15509. CrossRef
7. J. H. Rigby, U. S. M. Maharoof, and M. E. Mateo, J. Am. Chem. Soc., 2000, 122, 6624. CrossRef
8. H. Ko, E. Kim, J. E. Park, D. Kim, and S. Kim, J. Org. Chem., 2004, 69, 112. CrossRef
9. J. L. Aceña, O. Arjona, M. L. León, and J. Plumet, Org. Lett., 2000, 2, 3683. CrossRef
10. T. J. Doyle, M. Hendrix, D. VanDerveer, S. Javanmard, and J. Haseltine, Tetrahedron, 1997, 53, 11153. CrossRef
11. N. Chida, M. Jitsuoka, Y. Yamamoto, M. Ohtsuka, and S. Ogawa, Heterocycles, 1996, 43, 1385. CrossRef
12. For alternative synthetic routes see: (a) S. Danishefsky and J. Y. Lee, J. Am. Chem. Soc., 1989, 111, 4829; CrossRef (b) G. R. Pettit, N. Melody, and D. L. Herald, J. Org. Chem., 2001, 66, 2583; CrossRef (c) M. Li, A. Wu, and P. Zhou, Tetrahedron Lett., 2006, 47, 3707; CrossRef (d) A. Padwa and H. Zhang, J. Org. Chem., 2007, 72, 2570; CrossRef (e) A. E. Håkansson, A. Palmelund, H. Holm, and R. Madsen, Chem. Eur. J., 2006, 12, 3243; CrossRef (f) J. H. Dam and R. Madsen, Eur. J. Org. Chem., 2009, 4666; CrossRef (g) G. E. Keck, S. F. McHardy, and J. A. Murry, J. Org. Chem., 1999, 64, 4465. CrossRef
13. For reviews see: (a) U. Rinner and T. Hudlicky, Synlett., 2005, 365; CrossRef (b) Y. Chapleur, F. Chrétien, S. I. Ahmed, and M. Khaldi, Curr. Org. Synth., 2006, 3, 341; CrossRef (c) T. Hudlicky, ARKIVOC, 2006, vii, 276; (d) M. Manpadi and A. Kornienko, Org. Prep. Proced. Int., 2008, 40, 107; CrossRef (e) A. Kornienko and A. Evidente, Chem. Rev., 2008, 108, 1982; CrossRef (f) J. Collins, U. Rinner, M. Moser, T. Hudlicky, I. Ghiviriga, A. E. Romero, A. Kornienko, D. Ma, C. Griffin, and S. Pandey, J. Org. Chem., 2010, 75, 3069. CrossRef
14. (a) M.-R. Brescia and P. DeShong, J. Org. Chem., 1998, 63, 3156; CrossRef (b) M. E. Mowery and P. DeShong, J. Org. Chem., 1999, 64, 1684; CrossRef (c) R. Correia and P. DeShong, J. Org. Chem., 2001, 66, 7159. CrossRef
15. S. E. Denmark and N. S. Werner, J. Am. Chem. Soc., 2008, 130, 16382. CrossRef
16. K. H. Shukla, D. J. Boehmler, S. Bogacyzk, B. R. Duvall, W. A. Peterson, W. T. McElroy, and P. DeShong, Org. Lett., 2006, 8, 4183. CrossRef
17. N.-C. Yang, M.-J. Chen, and P. Chen, J. Am. Chem. Soc., 1984, 106, 7310. CrossRef
18. S. F. Martin and H. Tso, Heterocycles, 1993, 35, 85. CrossRef
19. T. Hudlicky, U. Rinner, D. Gonzalez, H. Akgun, S. Schilling, P. Siengalewicz, T. A. Martinot, and G. R. Pettit, J. Org. Chem., 2002, 67, 8726. CrossRef
20. S. Elango and T. Yan, Tetrahedron, 2002, 58, 7335. CrossRef
21. T. Hudlicky, M. Stabile, D. T. Gibson, and G. M. Whited, Org. Synth., 1999, 76, 77.
22. T. Hudlicky and H. F. Olivo, Tetrahedron Lett., 1991, 32, 6077. CrossRef
23. N. E. Jenkins, R. W. Ware, R. N. Atkinson, and S. B. King, Synth. Commun., 2000, 30, 947. CrossRef
24. G. K. Tranmer and W. Tam, Org. Lett., 2002, 4, 4101. CrossRef
25. A. S. Manoso and P. DeShong, J. Org. Chem., 2001, 66, 7449. CrossRef
26. A. S. Manoso, C. Ahn, A. Soheili, C. J. Handy, R. Correia, W. M. Seganish, and P. DeShong, J. Org. Chem., 2004, 69, 8305. CrossRef
27. K. H. Shukla and P. DeShong, J. Org. Chem., 2008, 73, 6283. CrossRef
28. M. Green, J. A. K. Howard, J. L. Spencer, and F. G. A. Stone, J. Chem. Soc., Chem. Comm., 1975, 449. CrossRef
29. M. Green, J. A. K. Howard, J. L. Spencer, and F. G. A. Stone, Chem. Soc., Dalton Trans., 1977, 271. CrossRef
30. K. Itoh, F. Ueda, K. Hirai, and Y. Ishii, Chem. Lett., 1977, 877. CrossRef
31. M. Hiramatsu, K. Shiozaki, T. Fujinami, and S. Sakai, J. Organomet. Chem., 1983, 246, 203. CrossRef
32. Y. Yamamoto, T. Ohno, and K. Itoh, Organometallics, 2003, 22, 2267. CrossRef
33. J. Krause, G. Cestaric, K.-J. Haack, K. Seevogel, W. Storm, and K.-R. Pörschke, J. Am. Chem. Soc., 1999, 121, 9807. CrossRef
34. M. Schwalbe, D. Walther, H. Schreer, J. Langer, and H. Görls, J. Organomet. Chem., 2006, 691, 4868. CrossRef
35. A. M. Kluwer, C. J. Elsevier, M. Bühl, M. Lutz, and A. L. Spek, Angew. Chem. Int. Ed., 2003, 42, 3501. CrossRef
36. M. A. Grundl, J. J. Kennedy-Smith, and D. Trauner, Organometallics, 2005, 24, 2831. CrossRef
37. J. Masllorens, A. Pla-Quintana, T. Parella, and A. Roglans, ARKIVOC, 2010, iii, 203.
38. L. Canovese and F. Visentin, Inorg. Chim. Acta, 2010, 363, 2375. CrossRef
39. K. Blum, E. S. Chernyshova, R. Goddard, K. Jonas, and K.-R. Pörschke, Organometallics, 2007, 26, 5174. CrossRef
40. M. Moreno-Mañas, R. Pleixats, A. Roglans, R. M. Sebastián, and A. Vallribera, ARKIVOC, 2004, iv, 109.
41. A. Dachs, J. Masllorens, A. Pla-Quintana, A. Roglans, J. Farjas, and T. Parella, Organometallics, 2008, 27, 5768. CrossRef
42. E. Badetti, A.-M. Caminade, J.-P. Majoral, M. Moreno-Mañas, and R. M. Sebastián, Langmuir, 2008, 24, 2090. CrossRef
43. P. S. Bäuerlein, I. J. S. Fairlamb, A. G. Jarvis, A. F. Lee, C. Müller, J. M. Slattery, R. J. Thatcher, D. Vogt, and A. C. Whitwood, Chem. Commun., 2009, 5734. CrossRef
44. M. E. Hoke, M.-R. Brescia, S. Bogacyzk, P. DeShong, B. W. King, and M. T. Crimmins, J. Org. Chem., 2002, 67, 327. CrossRef