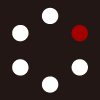
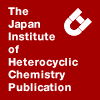
HETEROCYCLES
An International Journal for Reviews and Communications in Heterocyclic ChemistryWeb Edition ISSN: 1881-0942
Published online by The Japan Institute of Heterocyclic Chemistry
e-Journal
Full Text HTML
Received, 14th June, 2012, Accepted, 19th July, 2012, Published online, 23rd July, 2012.
DOI: 10.3987/REV-12-746
■ Total Synthesis of Manzacidins. An Overview and Perspective
Yasufumi Ohfune,* Kentaro Oe, Kosuke Namba, and Tetsuro Shinada
Graduate School of Science, Osaka City University, Sugimoto, Sumiyoshi-ku, Osaka 558-8585, Japan
Abstract
Manzacidins belong to a class of non-oroidin bromopyrrole alkaloids and have attracted significant attention from the synthetic community due to not only their expected biological activities but also to their novel structure consisting of a tetrahydropyrimidinecarboxylic acid with an ester-linked bromopyrrole unit. Of particular interest was the stereoselsective construction of the 1,3-diamino groups attached to chiral tertiary and quaternary carbon centers in the tetrahydropyrimidine unit. Since our total synthesis and structure confirmation of manzacidins A and C in 2000, many groups have accessed the enantio- and diastereoselective total syntheses of these alkaloidal amino acids based on novel synthetic methods. This review will focus on recent studies from our laboratory as well as others regarding the total syntheses of manzacidins A, B, C, and D reported to date.CONTENTS
1. Introduction
2. Total Synthesis of Manzacidins A and C by Our Group
2-1. First Total Synthesis and Absolute Configuration of Manzacidins A and C using Asymmetric Strecker Synthesis
2-2. Short and Diastereoselective Route to the Second Generation Total Synthesis of Manzacidins A and C using Diastereoselective Hydrogenation of α,β-Dehydroamino Acid
3. Total Synthesis of Manzacidin A and/or C by Other Groups
3-1. Total Synthesis of Manzacidins A and C using Rhodium-catalyzed C-H Insertion of Nitrene by Du Bois’ Group
3-2. Total Synthesis of Manzacidin C using Asymmetric Aza-Mannich Reaction by Lanter’s Group
3-3. Total Synthesis of Manzacidin A using Lewis Acid-catalyzed Asymmetric 1,3-Dipolar Cycloaddition by Maruoka’s Group
3-4. Total Synthesis of Manzacidin A using Lewis Acid-catalyzed Asymmetric 1,3-Dipolar Cycloaddition by Sibi’s Groups
3-5. Formal Total Synthesis of Manzacidins A and C using Cinchona Alkaloid-derived Organocatalysts by Deng’s Group
3-6. Total Synthesis of Manzacidin C using Chiral Silicon-based Lewis Acid-promoted Asymmetric Acylhydrazone-vinyl Ether Cycloadditions by Leighton’s Group
3-7. Total Synthesis of Manzacidin A and Formal Synthesis of Manzacidin C using Allyl Cyanate-to-isocyanate Rearrangement by Ichikawa’s Group
3-8. Total Synthesis of Manzacidin D using Isothiourea Iodocyclization by Mackay’s Group
4. Total Synthesis of Manzacidin B
4-1. Synthesis and Structure Determination of Manzacidin B by Our Group
4-2. Second Generation Total Synthesis of Manzacidin B by Our Group
4-3. Total Synthesis of Manzacidin B and Its Enantiomer and Diastereomers using Hatakeyama’s Epoxide-Opening by Mohapatra’s Group
5. Conclusion
1. INTRODUCTION
Bromopyrrole alkaloids are a novel family of marine natural products possessing potentially useful pharmacological activities which include α-adrenoceptor blockers, antagonists of serotonergic receptors, actomyosin ATPase activators, and immunosuppressive and anti-tumor properties.1 About 160 members of this class have been isolated so far from more than 20 different sponges of various genera, essentially belonging to Agelasidae, Axinellidae, and Halichondridae families. Oroidin (1) is considered the parent compound of this family. These alkaloids are classified according to their structures: (i) oroidin-like linear monomers in which hymenidin (2) belongs to this class; (ii) cyclized oroidin derivatives represented by himenin (3) and spongiacidin B (4) derived from 1 or 2, and dibromophakellin (6) and agelastatins A (7) and B (8) derived from dihydrooroidin (5); (iii) an oroidin-like dimer and its higher oligomers such as sceptrin (9), a [2+2] adduct of 1, ageliferin (10), a formal [4+2] adduct of 1, and dibromopalau’amine (12) and palau’amine (13) via axinellamine A (11) from 9 or 10 (Figure 1).2,3 Recently, non-oroidin bromopyrrole alkaloids without the imidazole ring originating from oroidin were isolated from several marine sponges. Bromopyrrolohomoarginine (14), agelongine (15), manzacidins A (16), B (18), C (17),4-6 and manzacidin D (19)7 belong to this class, whose structures are characterized by an ester linkage in place of the amide linkage between the bromopyrrole unit and the remaining part of the molecule except for 14 (Figure 2).
Among these alkaloids, manzacidins A (16), B (18), and C (17) were isolated from the Okinawan sponge Hymeniacidon sp. by Kobayashi et al. in 1991.4 Although biological activities of manzacidins were expected to be similar to those of other bromopyrrole alkaloids,1 their pharmacological profile remained unexploited because only minute quantities were available from the marine sponges. The structures of manzacidins A and C were proposed as the C4 (manzacidin numbering) diastereomers with the same 6S configuration based on their spectroscopic studies. Finally, their structures including absolute configuration have been confirmed to be (4S,6R)-16 and (4S,6S)-17, respectively, by our first total synthesis in 2000 as shown in Figure 2.8 The structure of manzacidin B was also confirmed to be (4R,5R,6R)-18 by our recent synthetic studies.9,10
Since our total synthesis of 16 and 17, these alkaloids have attracted much attention as synthetic targets from the synthetic community not only due to the presumed biological activities but also their novel structural features, i.e., the 1,3-diamino system in the tetrahydropyrimidine core in which one of the amino groups was attached to a C6 quaternary carbon center. Thus, many synthetic studies were focused on enantio- and diastereoselective methods for the construction of this structural unit. Many intriguing methods have been developed and successfully applied to the efficient total synthesis of manzacidins. An excellent review article on the manzacidin syntheses based on these novel synthetic methods has been reported by Maruoka et al. in 2008.11 After Maruoka’s review, many studies regarding the total syntheses of manzacidins have continuously appeared in the literature. In this review, our studies on the total synthesis of manzacidins including other groups reported to date are summarized.
2. TOTAL SYNTHESIS OF MANZACIDINS A AND C BY OUR GROUP
2-1. First Total Synthesis and Absolute Configuration of Manzacidins A and C using Asymmetric Strecker Synthesis
Not only manzacidins but also many natural products often possess a nitrogen-containing quaternary carbon center. Therefore, its enantio- and/or diastereoselective constructions have been one of the most challenging issues in organic synthesis for the last two decades.12,13 In particular, manzacidins have provided valuable opportunities for examining new synthetic methods for the construction of this novel structural unit involved in highly functionalized structures. We thought that stereoselective synthesis of optically active α-substituted α-amino acids from an achiral starting material would provide a useful chiral building block for the synthesis of many natural products including manzacidins. Therefore, our group has developed an asymmetric version of the Strecker synthesis for the preparation of various α-substituted α-amino acids.14 The asymmetric Strecker synthesis consisted of the following sequence of transformations: (i) formation of a cyclic ketimine intermediate from an α-acyloxy ketone, having an L- or D-amino acid as the acyloxy group which controls the facial selectivity of the cyanide addition; (ii) stereoselective addition of a cyanide ion to 1,4-oxazine to give the α-amino nitrile; (iii) oxidative conversion to the α-imino nitrile; and (iv) removal of the chirality transferring amino acid as a pyruvate derivative and simultaneous hydrolysis of the nitrile group under acidic conditions to give the optically active β-hydroxy α-substituted α-amino acid (Scheme 1). The chirality transferring processes in Scheme 1 were highly stereoselective giving various types of chiral acyclic and cyclic β-hydroxy-α-substituted α-amino acids. The presence of a hydroxyl group adjacent to the nitrogen-containing quaternary carbon center of the resulting amino acids was advantageous for the conversion to natural products and related biologically active compounds. Thus, the present asymmetric Strecker synthesis has proven to be a useful method for the enantio- or diastereoselective construction of the nitrogen-containing quaternary carbon center.12,13
The stereochemical relationship between manzacidins A (16) and C (17) was originally proposed as the C4 diastereomers.4 We hypothesized that their C4 configuration would be the same S by considering a plausible biosynthetic pathway that involves an (R)- or (S)-isocyanide intermediate 20 as is often seen in the biosynthesis of marine natural products.15 Thus, our plan for the total synthesis of manzacidin A and C was the use of the asymmetric Strecker synthesis starting with (2S)-allylglycinol (24) as shown in Scheme 2. This route relies on a stereoselective construction of the amino nitrile 21 by the Strecker synthesis of the amino ketone 23. However, an asymmetric Strecker synthesis using an amide system is unknown, and the stereochemistry of a cyanide addition to the imine 22 is unpredictable in this case because its C4 chiral center also affects the facial selectivity, while in our previous work, the nitrile addition to a six-membered ketimine intermediate occurred from the sterically less demanding re-face (Scheme 1).
Initially, Strecker precursors, 25 with L-Phe and 26 with D-Phe as the chirality and nitrogen-transferring group, were prepared, respectively, from (S)-24. To examine the effect of the chiral amino acid for the diastereoselective cyanide addition to the corresponding imine, the achiral glycine precursor 27 was also prepared. After chemoselective removal of the Boc group with TMSOTf/2,6-lutidine,16 the Strecker synthesis of the L-Phe derivative 25 with TMSCN underwent stereoselective cyanide addition to give (6R)-α-amino nitrile 28 as a single diastereomer. The D-Phe isomer 26 furnished (6S)-α-amino nitrile 29, exclusively. The reaction of the achiral glycyl derivative 27 gave a mixture of amino nitriles 30 in a 5:1 ratio, indicating that the cyanide addition preferentially occurred from the sterically less demanding re-face of the bicyclic framework. These results indicated that the cyanide addition to L- or D-Phe-derived imine, A or B, occurred from the opposite face of the benzyl group to those of the cyanide addition to the imine intermediate in a 6-membered case, despite the fact that the cyanide addition to L-Phe-derived imine A suffered severe steric demand from the bicyclic framework as observed in the glycyl case as shown in Scheme 3. Thus, the desired α-amino nitriles, 28 and 29, which corresponded to the stereochemistry of manzacidins A and C, respectively, were obtained with excellent stereocontrol.
Upon conversion of 28 into the corresponding α-imino nitrile 31, neither a standard oxidation method using t-BuOCl/Et3N nor other combinations of oxidants and bases were successful. Fortunately, the oxidation with ozone was quite effective to give the α-imino ketone 31 in 94% yield.17 Treatment with concd HCl resulted in removal of phenylpyruvic acid and simultaneous hydrolysis of the nitrile group to afford diaminocarboxylic acid 32. This was converted into the δ-lactone 34 via diol 33, to which a tetrahydropyrimidine ring was introduced to give 35. The synthesis of manzacidin A (16) was accomplished by coupling with the bromopyrrole moiety using 4-bromo-2-trichloroacetylpyrrole (36). Thus, the absolute configuration of natural (–)-manzacidin A could unambiguously be assigned to (4S,6R)-16 (Scheme 4).8 It is noteworthy that the present bromopyrrole ester-forming reaction has been employed by all other forthcoming syntheses of manzacidins as the standard method.
The synthesis of manzacidin C was started with the (6S)-amino nitrile 29. Oxidative removal of the D-Phe unit from 29 was performed by trioxorhenium oxidation,18 followed by hydrolysis of the resulting 37 with concd HCl to give cyclic urea 38 via a C-C bond cleavage of the putative intermediate C, because ozone was exceptionally ineffective for this oxidation. Hydrolysis of the cyclic urea 38 and a subsequent 6-step sequence of reactions gave methyl ester 39. This was converted to tetrahydropyrimidine 40 in 3 steps. The bromopyrrole moiety was introduced to 40 in a manner similar to that of 35 which gave manzacidin C (17). Thus, both natural (–)-manzacidin A and (+)-manzacidin C were found to possess the same 4S configuration. The synthetic sequence of manzacidin A involved 14 steps and the overall yield was 14%. Manzacidin C was synthesized in 15 steps and the overall yield was 3.5%.8
2-2. Short and Efficient Route to the Second Generation Total Synthesis of Manzacidins A and C using Diastereoselective Hydrogenation of α,β-Dehydroamino Acid Esters
Since our first total synthesis of manzacidins A and C in 2000,8 these natural products have received much attention in view of the enantio- and stereoselective construction of the 1,3-diamino system attached to the C4 tertiary and C6 quaternary stereogenic centers. However, the scalable method for preparing these alkaloids and their analogs remained a challenging program. Our group examined a short and efficient synthesis of manzacidins A (16) and C (17), and their enantiomers, ent-16 and ent-17, by a diastereoselective hydrogenation of α,β-dehydroamino acid esters using a chiral rhodium catalyst (Scheme 6). To examine the substrate-controlled or chiral catalyst-controlled hydrogenation, two types of α,β-dehydroamino acid esters, 41 and 42, were prepared by the Horner-Wadsworth-Emmons olefination of optically active α-methyl Garner aldehyde 4310 and ring-opened aldehyde 4819 with Schmidt’s dimethyl phosphonoglycinate.20
Attempts for the substrate-controlled hydrogenation of the acetonide (S)-41 using achiral catalyst systems revealed that the observed diastereoselectivities were poor to moderate giving a mixture of 4S and 4R isomers 44. The 1,4-reduction conditions did not improve the ratio to a satisfactory level. The sterically congested functional groups attached to the olefin, which sterically hindered both the re- and si-faces of the proposed conformer D, would prevent the diastereoselective hydrogenation pathway (Scheme 7).
Next, we examined the chiral catalyst-controlled hydrogenation reaction using a Rh(I)-(S,S)- or (R,R)-Et-DuPHOS catalyst.21,22 The N-Boc olefin (S)-41 was smoothly reduced with the Rh(I)-(S,S)-catalyst to give a 1:1 mixture of 45 and 46. On the other hand, the use of the (R,R)-catalyst proceeded in a highly diastereoselective manner to give 46 (ent-16 form) as the major isomer (13:1). The combination of (S)-41 with the (R,R)-catalyst can be viewed as a matched-pair double-asymmetric induction.23 These results indicated that the manzacidin A form can be prepared by the combination of (R)-41 with the Rh(I)-(S,S)-catalyst. In fact, the asymmetric hydrogenation gave (4S,6R)-47 as the major isomer in a 10:1 ratio (Scheme 8).
To achieve the diastereoselective hydrogenation for the synthesis of manzacidin C (17) and its enantiomer (ent-17), we considered that the release of the ring strain, which reduced the steric hindrance of the cyclic oxazolidine 43, could facilitate the chiral catalyst-controlled hydrogenation reaction. As expected, the reduction of the acyclic olefin (S)-42 with the (S,S)-Rh catalyst gave (4S,6S)-manzacidin C form 49 as the major product (dr = 9:1). When the (R,R)-catalyst was employed, the diastereoselectivity was inverted to give (4R,6S)-50, the ent-manzacidin A form, as the major product (dr = 6:1). The remaining ent-manzacidin C form 51 was prepared from (R)-42 with the (R,R)-catalyst (dr = 9:1). The facial selectivity of the present catalyst-controlled hydrogenation reaction would proceed through the proposed conformer E as shown in Scheme 9.
The resulting isomers 46, 47, 49, and 51 were subjected to the following sequence of transformations: (i) hydrolysis of the methyl ester, (ii) removal of the Boc groups, (iii) tetrahydropyrimidine formation, and (iv) esterification with 36 to give manzacidins A and C, and their respective enantiomers. It is noteworthy that heptafluorobutyric acid was an excellent additive for the distinct HPLC separation of a mixture of the manzacidins that enabled obtaining enantio- and diastereomerically pure manzacidins A (16) and C (17), and their enantiomers ent-16 and ent-17 on a 100-mg scale. Thus, the syntheses of 4 isomers of manzacidins A and C were accomplished using the diastereoselective hydrogenation of the α,β-unsaturated amino acid esters using the chiral Rh-DuPHOS catalyst. Manzacidin A was obtained from the (S)-α-methyl Garner aldehyde 46 in 6 steps (18.1% overall yield, 15.8% for ent-16), and manzacidin C was synthesized from (R)-Boc-α-methylserine via (R)-48 in 9 steps (21.6% overall yield, 24% for ent-17) as diastereomerically pure samples after the above-mentioned HPLC separation (Scheme 10).19
3. TOTAL SYNTHESIS OF MANZACIDIN A AND/OR C BY OTHER GROUPS
3-1. Total Synthesis of Manzacidins A and C using Rhodium-catalyzed C-H Insertion of Nitrene by Du Bois’ Group
Amination reaction of unactivated C-H bonds offers great potential as methods for the synthesis of amine derivatives. Du Bois et al. have established diastereoselective preparation of a 1,3-diamino system via an efficient γ-C-H amination reaction. Oxidation of a sulfamate ester with iodosobenzene diacetate and catalytic Rh2(OAc)4 afforded the corresponding six-membered ring insertion product through regioselective γ-C-H insertion of the [Rh]-nitrene complex (Scheme 11).24 The synthesis of manzacidin A (16) began with the preparation of (4R,6R)-4-hydroxy-6-methyl ester 53 (dr = 75:25) by the diastereoselective hydroxylation of the olefin 52 using a chiral Rh catalyst, (R)-PHANEPHOS. The treatment of the mixture with ClSO2NCO afforded a mixture of the sulfamate ester 54 from which a minor diastereomer was removed. The sulfamate ester 54 played dual roles, i.e., as a tether for the C-H amination at C6 and as a leaving group for the SN2 azidation reaction at C4. The key C-H amination reaction proceeded in a highly regio- and stereoselective manner to give cyclic sulfamate 55 as a single diastereomer. The resulting 55 was converted to tetrahydropyrimidine 57 by the following sequence of reactions; (i) protection with a Boc group, (ii) SN2-type substitution reaction at C4 with an azide group to give 56, (iii) reduction of the azide group and subsequent formylation of the resulting amino group, and (iv) tetrahydropyrimidine formation with POCl3. Removal of the global protecting groups followed by introduction of the bromopyrrole moiety according to the standard method8 gave manzacidin A (16) (Scheme 11). Manzacidin C (17) was prepared from (4R,6S)-4-hydroxy-6-methyl ester 58 in a manner similar to those of manzacidin A. The total numbers of synthetic sequences from 52 were 9 steps, and the overall yield was 28% for manzacidin A and 32% for manzacidin C.25
3-2. Total Synthesis of Manzacidin C using Asymmetric Aza-Mannich Reaction by Lanter’s Group
Chiral sulfinyl imine is a versatile building block for the asymmetric synthesis of chiral amines. Ellman et al. reported that α-deprotonation of chiral N-tert-butanesulfinyl imines provided metalloenamines, which, upon reaction with aldehydes, gave β-hydroxy-N-sulfinyl ketimines in high yields with high diastereoselectivities.26 The sulfinyl group also activates the imine, and the configurationally stable stereocenter at the sulfur atom can provide diastereofacial selectivity for nucleophilic additions. Moreover, the sulfinyl group can be readily cleaved by an acidic treatment.
Lanter et al. extensively applied this method to the addition reaction of the metalloenamine to N-tert-butylsulfonyl (Bus) imine to give β-sulfonamidosulfinyl imine in a highly diastereoselective manner. Subsequent addition of an organometallic reagent to the resulting products possessing an activated sulfinylimino group underwent a nucleophilic addition reaction to give 1,3-diamine in which one of the amino groups was attached to a quaternary carbon center. Based on these methods, Lanter and co-workers developed a novel route to manzacidin C (17) as shown in Scheme 12. Deprotonation of the sulfinyl imine 58 with LHDMS followed by reaction with tert-butylsulfonyl imine 59 afforded β-sulfonamidosulfinyl imine 60 in 85% yield (dr = 99:1). The quaternary carbon center at C6 was constructed by the highly diastereoselective addition of methylmagnesium bromide. After removal of the N-sulfinyl group of the resulting product, (4S,6S)-diamino compound 61 was obtained in 65% yield from 60. The 1,3-diamine 61 was converted to tetrahydropyrimidine 62. Oxidative cleavage of the styrene moiety followed by the removal of the benzyl protecting group afforded 40 which was converted to manzacidin C (17) using the standard method (Scheme 12).8 The total numbers of synthetic sequences from 58 were 9 steps and the overall yield was 27%.27
3-3. Total Synthesis of Manzacidin A using Lewis Acid-catalyzed Asymmetric 1,3-Dipolar Cycloaddition by Maruoka’s Group
Maruoka and co-workers reported a catalytic asymmetric 1,3-dipolar cycloaddition reaction of α-substituted acrolein with α-diazoacetates using titanium-BINOLate (composed of (S)-BINOL and Ti(Oi-Pr)4 or ((iPrO)3Ti)2O).28 Various optically active 2-pyrazolines were prepared in moderate yields with an enantiomeric excess of 80-94% according to the substrates employed. Because the cycloadducts possessed a nitrogen-containing quaternary carbon center and other functional groups corresponding to manzacidins, application of this method to the total synthesis of manzacidin A (16) was carried out. The synthesis began with the titanium BINOLate-catalyzed 1,3-dipolar cycloaddition of methacrylate with ethyl diazoacetate to give 2-pyrazoline 63 in 52% yield (95% ee). This was converted to bicyclic compound 64 in which the requisite formyl group as the amidine carbon was pre-installed. The resulting bicyclic compound 64 was treated with Raney-nickel to give tetrahydropyrimidine 35 as an 85:15 mixture of diastereomers. This transformation involved several reactions, i.e., (i) hydrolysis of the formamide acetal, (ii) reduction of the C-N double bond, (iii) the reductive cleavage of the N-N bond, (iv) formation of the cyclic amidine, and (v) hydrolysis of the ester group. Maruoka et al. proposed that the stereochemical outcome of this transformation involved the epimerization of the ester group between 65 and 66. The ester group was removed simultaneously via a lactonization to give 35. Whether the reaction involved the lactone intermediate 67 in Maruoka’s synthesis, our experimental results would support this hypothesis, i.e., tetrahydropyrimidine formation from the methyl ester 68 with an unprotected alcohol gave cleaved ester 35 due probably to the intermediary formation of the lactone 69, while the ester group was not removed using a protected alcohol under the same reaction conditions to give manzacidin A methyl ester 71.28 Finally, esterification of the tetrahydropyrimidine 35 according to the standard procedure8 gave manzacidin A (16). The total numbers of synthetic sequences are extremely short (5 steps) and the overall yield was 16%.29
3-4. Total Synthesis of Manzacidin A using Lewis Acid-catalyzed Asymmetric 1,3-Dipolar Cycloaddition by Sibi’s Group
Sibi and co-workers have developed an enantioselective 1,3-dipolar cycloaddition of ethyl diazoacetate with α,β-unsaturated carbonyl 73 using chiral ligand 72 and Mg(NTf2) 2 as a Lewis acid. The advantage of this method would be a diverse array of the substituent-attached unsaturated carbonyls providing the cycloadducts in good yields with an excellent enantiocontrol. The cycloadduct 74 was transformed to Maruoka’s bicyclic intermediate 64 in two steps. This was converted to manzacidin A according to the Maruoka’s procedure (Scheme 14).30 The total numbers of synthetic sequences are extremely short (5 steps) and the overall yield was 32%.31
3-5. Formal Total Synthesis of Manzacidins A and C using Cinchona Alkaloid-derived Organocatalysts by Deng’s Group
Deng and co-workers reported an asymmetric Michael addition of thiomethyl α-cyanopropionate (77) to 2-chloroacrylonitrile using cinchona alkaloid-based organocatalysts 75 and 76.32 The characteristic point of this catalytic system is the sequential asymmetric conjugate addition and protonation steps, which enabled the highly stereoselective formation of 1,3-tertiary and quaternary stereogenic centers corresponding to those of manzacidins A and C (Scheme 15). The use of the quinidine-derived catalyst 75 for the asymmetric conjugate addition and protonation afforded 78, and the enantiomeric catalyst bearing a thiourea moiety gave its C6-epimer 79 in excellent yields with high ees. Substitution of the chloride 78 with an azide group, selective methanolysis of the sterically less hindered nitrile group, and reduction of the thioester and ester groups gave the diol 80. After protection of the hydroxy groups, the hydration of the nitrile group provided amide 81. The azide group was converted to the corresponding Boc-protected amide, which, upon Hofmann rearrangement and removal of the TBDPS group, furnished the diol 33. Because the diol 33 was previously converted to manzacidin A by our group,8 the synthesis of 33 constitutes a formal asymmetric total synthesis of manzacidin A (16) in 13 steps.32 The formal synthesis of manzacidin C (17) was also accomplished from 79 by converting it to the known diol 82.8,33
3-6. Total Synthesis of Manzacidin C using Chiral Silicon-based Lewis Acid-promoted Asymmetric Acylhydrazone-vinyl Ether Cycloadditions by Leighton’s Group
Leighton and co-workers developed an asymmetric acylhydrazone-vinyl ether cycloaddition using silicon-based Lewis acid 83 to produce pyrazolidine products with high enantioselectivity as shown in Scheme 16.34 This method was expected to be applicable to the construction of the C4 and C6 chiral centers of manzacidin C (17) in a single operation when methallyl silyl ether was employed as the vinyl ether. This was accomplished by preactivation of the silane Lewis acid (R,R)-85 with AgOTf and the use of thienylhydrazone 84 to give the cycloadduct 86 as a single diastereomer. Formylation of the resulting hydrazide followed by reduction with SmI2 gave bisamide 87. Combination of PCl5 and 4-dimethylaminopyridine in CHCl3 was effective in the tetrahydropyrimidine formation, and subsequent deprotection with concd HCl produced the tetrahydropyrimidine 40, which, upon installation of the bromopyrrole side chain according to the standard procedure,8 gave manzacidin C (17). Thus, total synthesis of manzacidin C was accomplished starting from the thienylhydrazone 84 in 6 steps (26% overall yield).35
3-7. Total Synthesis of Manzacidins A and Formal Synthesis of Manzacidin C using Allyl Cyanate-to-isocyanate Rearrangement by Ichikawa’s Group
Ichikawa et al. developed an allyl cyanate-to-isocyanate [3,3] sigmatropic rearrangement, which is a highly stereoselective carbon–nitrogen bond-forming process at sterically encumbered positions.36 Conversion of the resulting isocyanate to Boc-carbamate was effected by acidic or basic conditions in a complementary manner. The synthesis of manzacidin A began with methyl L-lactate. This was converted to α,β-unsaturated ester 88, which, upon a 3 step sequence of reactions involving Horner-Wadsworth-Emmons olefination using the Schmidt’s reagent,20 gave dienamide ester 89. Hydrogenation with Rh-(S,S)-Et-DuPHOS catalyst21 furnished γ,δ-unsaturated amino acid 90 as a single diastereomer. Initial efforts for allyl cyanate-to-isocyanate rearrangement using 91 resulted in an intramolecular capture of the intermediary isocyanate by the Cbz nitrogen to give cyclic urea 92. To prevent this process, the nitrogen was masked as the bis-carbamate protected amine 93. Dehydration of 93 underwent the allyl cyanate-to-isocyanate [3,3] sigmatropic rearrangement followed by treatment with t-BuOH and TMSCl to provide Boc-carbamate 94 in 78% yield. Ozonolysis of 94 followed by reduction of the resulting aldehyde afforded 95. This was converted to the known lactone 34 in two steps. Following the procedures reported by our group,8,19 sequential deprotection and treatment with methyl orthoformate and TFA gave the tetrahydropyrimidine 35. Finally, esterification of 35 with 36 and HPLC purification furnished manzacidin A (16) in 46% yield (Scheme 17). Formal synthesis of manzacidin C (17) was performed according to the procedure for those of 35. The total number of the synthetic sequences for manzacidin A was 18 steps and the overall yield was 8.8%.37
3-8. Total Synthesis of Manzacidin D using Isothiourea Iodocyclization by Mackay’s Group
Manzacidin D (19) was isolated from a coralline demsponge, Astroschera willeyana, in 1997.7 The structure was assigned to an N-methylated form of manzacidin A, although the pyrrole moiety lacked the bromine atom. Only one report appeared on the total synthesis of racemic 19 by Mackay’s group based on the stereoselective isothiourea iodocyclization which directly formed the manzacidin D’s tetrahydropyrimidine framework.38 The synthesis was started with t-butyl 2-(isopropenyl)glycinate (96) prepared from Boc-Gly-O-t-Bu. This was converted to thiourea 97 possessing a requisite N-methyl group on the thiourea. The iodocyclization using IBr proceeded in a highly diastereoselective manner to give 98 (dr = 95:5). Solvolysis of the iodine group in 98 with excess silver trifluoroacetate and subsequent treatment with hydrogen sulfide under basic conditions produced thiourea 99. Exposure to H2O2-urea followed by acidic treatment gave 100 with a minimal amount of epimerization at C4. Esterification with 2-trichloroacetylpyrrole using a method slightly modified from that of the standard conditions8 gave racemic manzacidin D (19) (Scheme 18). The total numbers of the process included 11 steps and the overall yield was 15.7%.39
4. TOTAL SYNTHESIS OF MANZACIDIN B
4-1. Synthesis and Structure Determination of Manzacidin B by Our Group
The structure of manzacidin B, which showed UV and IR absorption similar to those of manzacidin A, was proposed to be a C5-hydroxylated form of manzacidin A (16) by Kobayashi et al. in 1991.4 Our synthetic absolute configuration determination of manzacidins A and C in 20008 led us to speculate another possibility for the relative and absolute configuration of manzacidin B (18) to be a C5-hydroxylated form of manzacidin C (either 101a or 101b), because the 1H NMR J values of manzacidin C (2.8 and 3.8 Hz between C4H and C5H) closely resembled that of manzacidin B (2.2 Hz) in which those of manzacidin A were 4.9 and 9.9 Hz. Furthermore, the fact that both manzacidins A and C possessed the same 4S-configuration suggested that manzacidin B would possess a 4S configuration as shown in Scheme 19. However, our after-mentioned synthetic studies confirmed the structure of manzacidin B to be (4R,5R,6R)-18, a C5 hydroxylated form of ent-manzacidin A, as depicted in Figure 2.10
To determine the relative and absolute configuration of manzacidin B, our initial attempts were the aldol condensation of (R)-α-methyl Garner aldehyde (R)-43 with an enolate derived from methyl glycinate as shown in Scheme 20. The reaction gave an inseparable mixture of products 102 (the product’s ratio was 36:4:9:1) in which, after conversion of the product’s mixture into manzacidin isomers, one of the minor isomers corresponding to the above 9 of 36:4:9:1 ratio (Scheme 20) was found to be identical with natural manzacidin B.40 These results indicated that manzacidin B possessed a 6S configuration as did manzacidin C (17). However, the relative configuration at C4 and C5 could not be determined by their extensive spectroscopic studies. The products were oily compounds and our efforts to prepare a crystalline derivative for X-ray crystallographic analysis were not successful.
We turned our attention to establishing the structure of manzacidin B by the syntheses of four possible diastereomers using diastereochemically defined synthetic routes as shown in Scheme 21.9 The (4R,5S)- and (4S,5R)-isomers of manzacidin B, 110 and 111, were prepared from the E-olefin 103 via the following sequence of reactions; (i) dihydroxylation of the E-olefin (dr = 3:1), (ii) SN2 azidation at C4, (iii) reduction of the resulting azide, (iv) removal of the global protecting groups to give diaminocarboxylic acids, 104 and 105, (v) tetrahydropyrimidine formation, and (iv) esterification with bromopyrrole carboxylate 36. Both the 1H and 13C NMR spectral data for (4R,5S)- and (4S,5R)-isomers, 110 and 111, were not identical with those of natural manzacidin B, indicating that the stereochemistry of manzacidin B is either the (4R,5R)- or (4S,5S)-isomer. In order to prepare the (4R,5R)- and (4S,5S)-isomers, the aldol condensation of an isocyanoacetate with (R)-43 was examined.41 The reaction of t-butyl isocyanoacetate with (R)-43 in the presence of a catalytic amount of CuCl afforded a mixture of the trans-oxazolines, 106 and 107, (106:107 = 1:7). Conversion of the mixture to the (4R,5R)- and (4S,5S)-isomers of manzacidin B, 18 and 112, was carried out by the following sequence of reactions; (i) hydrolysis of the trans-oxazolines to diaminocarboxylic acids, 108 and 109, (ii) tetrahydropyrimidine formation, and (iii) esterification with bromopyrrole carboxylate 36. The minor isomer was identical in all respects to those of natural manzacidin B (18). However, we could not obtain logical proof regarding whether the minor isomer possessed (4R,5S)- or (4S,5R)-configuration at this stage. During the course of these studies, we observed an unusual epimerization of the major isomer 112 under a bromopyrrole ester formation (NaH, DMF) to give (4S,5R)-isomer 111. This indicated that the major isomer 112 possessed the (4R,5R)-configuration. However, this structural assignment was incorrect leading to the absolute configuration of natural manzacidin B as (4S,5S,6R)-18 (Scheme 21).9 This was revised to (4R,5R,6R)-18 by our extensive studies as described below.
Shortly after publication of the above-mentioned results, we continuously examined the crystallization of the isocyanoacetate aldol products for X-ray crystallographic analysis. As a result, two crystalline compounds, 113 and 109, were obtained as shown in Scheme 22. The X-ray structural analysis of an N-formyl lactone 113, obtained from the minor isomer of the oxazoline route whose structure corresponded to that of natural manzacidin B, indicated that the correct structure of manzacidin B was (4R,5R,6R)-18. In addition, the structure of 112 was also revised to (4S,5S,6R)-112 by the X-ray structural analysis of 109 prepared from the oxazoline route’s major isomer 107.10 These results revealed that the isomerization of 112 with NaH/DMF to 111 did not occur. It was found that the 1H NMR of the isomerized product was obtained using its hydrochloric acid salt whose spectrum was quite similar to that of the TFA salt of 111. This misunderstanding led to the incorrect assignment of the stereochemistry of 18.9
Because manzacidins A (16) and C (17) are configurational isomers at C6 to become 6S or 6R, we proposed that both natural products were biosynthesized from an (S)-α-amino acid 20 (Scheme 2).8 Based on this hypothesis, the structure of manzacidin B (18) was considered to be a C5 hydroxylated form of manzacidin A or C. However, the absolute configuration of manzacidin B, confirmed by our studies, suggested its possible biosynthetic pathway as follows:10 (i) manzacidin B was derived from an (R)-α-amino acid via a different biosynthetic pathway from manzacidins A and C, or (ii) its biosynthesis involved an epimerization pathway at C4 after the hydroxylation of manzacidin C at C5 because both manzacidins B and C possessed the same (6R)-configuration.
4-2. Second Generation Total Synthesis of Manzacidin B by Our Group
Our first generation total synthesis of manzacidin B (18) was accomplished in 4 steps (11% overall yield) starting from the (R)-α-methyl Garner aldehyde 43. Although this route is short and efficient, the diastereoselectivity problem based on the isocyanoacetate aldol reaction which gave trans-oxazolines 106 as a minor isomer (106/107 = 1:7) remained to be improved.9 Therefore, we examined the second generation total synthesis of manzacidin B which was focused on the inversion of the stereoselectivity from (4S,5S)-107 to (4R,5R)-106 by the screening of the catalysts, the use of structurally alternate aldehydes of 43, and a double asymmetric induction23 using a chiral aldol donor (Scheme 23).
Catalyst screening revealed that the [bis(N-tert-butylsalicyliden-iminate)]copper (Cu(t-butSal)2) catalyst dramatically enhanced the reaction rate and its yield (5 min, 94%) in comparison to CuCl (4 h, 88%). However, the diastereomeric ratio of the products was not affected by this and other catalysts due probably to the rigid and densely functionalized structure of the aldehyde 43 which facilitated the substrate-controlled aldol reaction. In fact, the use of the ring-opened aldol acceptors, TBS protected (R)-48 and MOM-protected (R)-114, decreased the undesired (4S,5S)-selectivity, i.e., (4R,5R)-115/(4S,5S)-116 = 1:3 and (4R,5R)-117/(4S,5S)-118 = 2:3 (Scheme 24).
Based on these results, the double asymmetric induction using the ring-opened aldehydes, (R)-48 and (R)-114, with an isocyanoacetate possessing a chiral auxiliary was examined. Remarkable diastereoselectivities for the aldol reactions using camphorsultams,42 (1R)-119 and (1S)-119 were observed as shown in Scheme 25; in particular, combination of the MOM-protected (R)-114 with (1R)-119 showed the highest (4R,5R)-selectivity to give the desired (4R,5R)-120 as the major isomer (84%, dr = 13:1). The reaction of (R)-114 with (1S)-119 afforded (4S,5S)-121 as a sole diastereomer. The exclusive formation of (4S,5S)-121 (dr = >20:1) from the aldehyde (R)-114 with (1S)-119 can be explained as a matched-pair double-asymmetric induction. On the other hand, in spite of the mismatched pair between (R)-114 and (1R)-119, the chiral auxiliary-based diastereocontrol would be attributed to the high diastereoselective formation of the desired (4R,5R)-120 (dr = 13:1). The major isomer (4R,5R)-120 would be produced via a proposed transition state F as depicted in Scheme 25 where approaches from the re-face of the Z-enolate of (1R)-119 and the re-face of the aldehyde (R)-114 were the kinetically favored process. Finally, the removal of the camphor sultam group of the MOM-protected (4R,5R)-120 under alkaline hydrolysis followed by acidic treatment gave amino acid 108, which, upon 3-step conversion using our established method,8 gave manzacidin B (18) (Scheme 25). It is specially noted that the present synthetic process did not involve any oxidation-reduction sequences. The total number of processes from the aldehyde (R)-114 to 18 included 6 steps (48% overall yield, 38% from (R)-α-methylserine).10
5-3. Total Synthesis of Manzacidin B and its Enantiomer and Diastereomers using Hatakeyama’s Epoxide-Opening by Mohapatra’s Group
Mohapatra and co-workers reported an alternative total synthesis of manzacidin B. The key steps employed for the total synthesis were a chelation-controlled epoxidation of Z-allylic alcohol attached to the Garner aldehyde 123 and subsequent Hatakeyama’s epoxide-opening reaction43 for the stereoselective construction of the C5 and C6 chiral centers (Scheme 26). The synthesis began with a Z-selective Horner-Wadsworth-Emmons olefination of the Garner aldehyde (R)-123 using the Ando-type diaryl phosphonopropionate.44 The reaction gave a mixture of α,β-unsaturated esters in which the desired Z-isomer was produced as the major isomer (Z/E = 4:1). The Z-unsaturated ester was reduced to the corresponding allylic alcohol 124, which, upon chelation-controlled epoxidation, afforded epoxy alcohol 125 as a single distereomer. Conversion of the epoxy alcohol to the imidate 126 with trichloroacetonitrile followed by Hatakeyama’s intramolecular epoxide-opening reaction with SnCl4 furnished the oxazoline derivative 127 possessing 3 consecutive chiral centers and functional groups corresponding to ent-manzacidin B. This was converted to the Boc protected triol 128 in 2 steps. The less hindered hydroxy group was selectively oxidized to give δ-lactone 129. Construction of the tetrahydropyrimidine 122 was performed in two steps, and subsequent introduction of the bromopyrrole group using the established method8 gave ent-manzacidin B (ent-18). Manzacidin B was synthesized starting with (S)-Garner aldehyde (S)-123 in a manner similar to those of ent-18. Starting from the E-isomer of 124 or its enantiomer, the (4S,5S,6R) and (4R,5R,6S)-isomers of manzacidin B were also synthesized. The synthesis of 18 started with the (S)-Garner aldehyde 123 involved 10 steps and the overall yield was 10%.45
4. CONCLUSION
In conclusion, the examples discussed here illustrate the recent progress in the enantio- and/or diastereoselective total synthesis of manzacidins A-D.46 Our own approaches and those of other groups described in this review highlighted the utility of new synthetic methodologies which would encourage further investigations focusing on the highly efficient synthesis of the related biologically active alkaloids. Furthermore, these studies allowed the preparation of hundreds of milligrams of manzacidins A-C and their enantiomers and diastereomers which would enable detailed pharmacological evaluation of these alkaloids in the near future.
Finally, Table 1 summarizes the total numbers of the following parameters employed in the total synthesis included in this review: construction reactions, oxidations and reductions including both strategic and non-strategic redox, functional group interconversions, protecting group manipulations, reaction steps from the known starting material, and total yields. These data would be useful to account for the efficiency of the total synthesis47 in view of recently proposed step economy,48 atom economy,49 and redox economy,50 while these criteria (parameters) do not include other valuable factors in the total synthesis, e.g., the structure confirmation of natural products, ease of enabling the synthesis of diverse analogs for the SAR studies, and development of new synthetic methods during the synthetic studies.
ACKNOWLEDGEMENTS
We wish to acknowledge the contributions of our colleagues whose names are reported in the references. This study was supported by a grant from the Research for the Future Program (99L01204) from the Japan Society for the Promotion of Science (JSPS) and Grants-in-Aid for Scientific Research from JSPS and the Ministry of Education, Culture, Sports, Science and Technology, Japan.
References
1. A. Al-Mourabit, M. A. Zancanella, S. Tilvi, and D. Romo, Nat. Prod. Rep., 2011, 28, 1229; CrossRef F. Scala, E. Fattorusso, M. Menna, O. Taglialatela-Scafati, M. Tierney, M. Kaiser, and D. Tasdemir, Marine Drugs, 2010, 8, 2162; CrossRef A. Aiello, E. Fattorusso, M. Menna, and O. Taglialatela-Scafati, ‘Modern Alkaloids,’ ed. by E. Fattorusso and O. Taglialatela-Scafati, Wiley-VCH, 2007, pp. 271-304; CrossRef J. W. Blunt, B. R. Copp, W.-P. Hu, M. H. G. Munro, P. T. Northcote, and M. R. Prinsep, Nat. Prod. Rep., 2008, 25, 35; CrossRef T. Endo, M. Tsuda, T. Okada, S. Mitsuhashi, H. Shima, K. Kikuchi, Y. Mikami, J. Fromont, and J. Kobayashi, J. Nat. Prod., 2004, 67, 1262; CrossRef J. W. Blunt, B. R. Copp, M. H. G. Munro, P. T. Northcote, and M. R. Prinsep, Nat. Prod. Rep., 2003, 20, 1; CrossRef D. J. Faulkner, Nat. Prod. Rep., 2002, 19, 1; D. J. Faulkner, Nat. Prod. Rep., 1998, 15, 113.
2. I. B. Seiple, S. Su, I. S. Young, A. Nakamura, J. Yamaguchi, L. Jørgensen, R. A. Rodriguez, D. P. O’Malley, T. Gaich, M. Köck, and P. S. Baran, J. Am. Chem. Soc., 2011, 133, 14710. CrossRef
3. U. M. Reinscheid, M. Köck, C. Cychon, V. Schmidts, C. M. Thiele, and C. Griesinger, Eur. J. Org. Chem., 2010, 6900. CrossRef
4. J. Kobayashi, F. Kanda, M. Ishibashi, and H. Shigemori, J. Org. Chem., 1991, 56, 4574. CrossRef
5. Manzacidin A was also isolated from Mediterranean marine sponges belonging to Axinella genera. W. Hassan, E. S. Elkhayar, R. A. Edrada, R. Ebel, and P. Proksch, Nat. Prod. Commun., 2007, 2, 1.
6. N-Methylated form of manzacidin C was also isolated from Axinella brevistyla. S. Tsukamoto, K. Tane, T. Ohta, S. Matsunaga, N. Fusetani, and R. W. M. van Soest, J. Nat. Prod., 2001, 64, 1576. CrossRef
7. T. Jahn, G. M. König, A. D. Wright, G. Worheide, and J. Reitner, Tetrahedron Lett., 1997, 38, 3883. CrossRef
8. K. Namba, T. Shinada, H. Teramoto, and Y. Ohfune, J. Am. Chem. Soc., 2000, 122, 10708. CrossRef
9. T. Shinada, E. Ikebe, K. Oe, K. Namba, M. Kawasaki, and Y. Ohfune, Org. Lett., 2007, 9, 1765. CrossRef
10. T. Shinada, E. Ikebe, K. Oe, K. Namba, M. Kawasaki, and Y. Ohfune, Org. Lett., 2010, 12, 2170; CrossRef T. Shinada, K. Oe, and Y. Ohfune, Tetrahedron Lett., 2012, 53, 3250. CrossRef
11. T. Hashimoto and K. Maruoka, Org. Biomol. Chem., 2008, 6, 829. CrossRef
12. Y. Ohfune and T. Shinada, Eur. J. Org. Chem., 2005, 5127; CrossRef Y. Ohfune, K. Sakaguchi, and T. Shinada, ‘Asymmetric Synthesis and Application of α-Amino Acids’, ed. by V. A. Soloshonok and K. Izawa, American Chemical Society, Oxford University Press, 2009, pp. 57-71.
13. M. Alias, C. Cativiela, M. D. Díaz-de-Villegas, J. A. Galvez, and Y. Lapena, Tetrahedron, 1998, 54, 14963; CrossRef C. Cativiela and M. D. Díaz-de-Villegas, Tetrahedron: Asymmetry, 2007, 18, 569. CrossRef
14. S.-H. Moon and Y. Ohfune, J. Am. Chem. Soc., 1994, 116, 7405; CrossRef Y. Ohfune, S.-H. Moon, and M. Horikawa, Pure Appl. Chem., 1996, 68, 645; CrossRef M. Horikawa, T. Nakajima, and Y. Ohfune, Synlett, 1997, 253; CrossRef Y. Ohfune, K. Namba, I. Takada, T. Kan, N. Horikawa, and T. Nakajima, Chirality, 1997, 9, 459; CrossRef Y. Ohfune and M. Horikawa, J. Synth. Org. Chem. Jpn., 1997, 55, 982; CrossRef Y. Ohfune and T. Shinada, Bull. Chem. Soc. Jpn., 2003, 76, 1115. CrossRef
15. Isonitrile-containing marine natural products, see: A. D. Wright, G. M. König, C. K. Angerhofer, P. Greenidge, A. Linden, and R. Desqueyroux-Faundez, J. Nat. Prod., 1996, 59, 710; CrossRef J. Kobayashi, M. Tsuda, A. Nemoto, Y. Tanaka, K. Yazawa, and Y. Mikami, J. Nat. Prod., 1997, 60, 719. CrossRef
16. M. Sakaitani and Y. Ohfune, J. Org. Chem., 1990, 55, 870; CrossRef M. Sakaitani and Y. Ohfune, Tetrahedron Lett., 1985, 26, 5543. CrossRef
17. K. Namba, M. Kawasaki, S. Iwama, I. Takada, M. Izumida. T. Shinada, and Y. Ohfune, Tetrahedron Lett., 2001, 42, 3733. CrossRef
18. A. Goti and L. Nannelli, Tetrahedron Lett., 1996, 37, 6025. CrossRef
19. K. Oe, T. Shinada, and Y. Ohfune, Tetrahedron Lett., 2008, 49, 7426. CrossRef
20. U. Schmidt, A. Lieverknecht, and J. Wild, Synthesis, 1984, 53. CrossRef
21. M. J. Burk, J. E. Feaster, W. A. Nugent, and R. L. Harlow, J. Am. Chem. Soc., 1993, 115, 10125; CrossRef M. J. Burk, Acc. Chem. Res., 2000, 33, 363. CrossRef
22. M. Shoji, N. Akiyama, K. Tsubone, L. L. Lash, J. M. Sanders, G. T. Swanson, R. Sasaki, K. Shimamoto, M. Oikawa, and M. Sasaki, J. Org. Chem., 2006, 71, 5208. CrossRef
23. S. Masamune, W. Choy, J. S. Petersen, and L. R. Sita, Angew. Chem., Int. Ed. Engl., 1985, 24, 1. CrossRef
24. C. G. Espino, P. M. When, J. Chow, and J. Du Bois, J. Am. Chem. Soc., 2001, 123, 6935. CrossRef
25. P. M. When and J. Du Bois, J. Am. Chem. Soc., 2002, 124, 12950. CrossRef
26. J. A. Ellman, T. D. Owens, and T. P. Tang, Acc. Chem. Res., 2002, 35, 984. CrossRef
27. J. C. Lanter, H. Chen, X. Zhang, and Z. Sui, Org. Lett., 2005, 7, 5905. CrossRef
28. H. Hanawa, T. Hashimoto, and K. Maruoka, J. Am. Chem. Soc., 2003, 125, 1708; CrossRef H. Hanawa, D. Uraguchi, S. Konishi, T. Hashimoto, and K. Maruoka, Chem. Eur. J., 2003, 9, 4405; CrossRef T. Kano, T. Hashimoto, and K. Maruoka, J. Am. Chem. Soc., 2005, 127, 11926. CrossRef
29. K. Namba, Ph. D. Dissertation, Osaka City University, 2000.
30. T. Kano, T. Hashimoto, and K. Maruoka, J. Am. Chem. Soc., 2006, 128, 2174. CrossRef
31. M. P. Sibi, L. M. Stanley, and T. Soeta, Org. Lett., 2007, 9, 1553. CrossRef
32. Y. Wang, X. Liu, and L. Deng, J. Am. Chem. Soc., 2006, 128, 3928. CrossRef
33. B. Wang, F. Wu, Y. Wang, X. Liu, and L. Deng, J. Am. Chem. Soc., 2007, 129, 768. CrossRef
34. S. Shirakawa, P. J. Lombardi, and J. L. Leighton, J. Am. Chem. Soc., 2005, 127, 9974. CrossRef
35. K. Tran, P. J. Lombardi, and J. L. Leighton, Org. Lett., 2008, 10, 3165. CrossRef
36. Y. Ichikawa, K. Tsuboi, and M. Isobe, J. Chem. Soc., Perkin Trans. 1, 1994, 2791. CrossRef
37. Y. Ichikawa, K. Okumura, Y. Matsuda, T. Hasegawa, M. Nakamura, A. Fujimoto, T. Masuda, K. Nakano, and H. Kotsuki, Org. Biomol. Chem., 2012, 10, 614. CrossRef
38. J. C. S. Woo and D. B. MacKay, Tetrahedron Lett., 2003, 44, 2881. CrossRef
39. C. Drouin, J. C. S. Woo, D. B. MacKay, and R. M. A. Lavigne, Tetrahedron Lett., 2004, 45, 7197. CrossRef
40. K. Namba, T. Shinada, and Y. Ohfune, unpublished result.
41. Y. Ito, T. Matsuura, and T. Saegusa, Tetrahedron Lett., 1985, 26, 5781; CrossRef Y. Ito, M. Sawamura, and T. Hayashi, J. Am. Chem. Soc., 1986, 108, 6405; CrossRef A. Togni and S. D. Pastor, Helv. Chim. Acta, 1989, 72, 1038. CrossRef
42. W. Oppolzer, Tetrahedron, 1987, 43, 1969; CrossRef W. Oppolzer, Pure Appl. Chem., 1990, 62, 1241. CrossRef
43. S. Hatakeyama, H. Matsumoto, H. Fukuyama, Y. Mukugi, and H. Irie, J. Org. Chem., 1997, 62, 2275. CrossRef
44. K. Ando, J. Org. Chem., 1997, 62, 1934; CrossRef K. Ando, J. Org. Chem., 1998, 63, 8411. CrossRef
45. K. Sankar, H. Rahman, P. P. Das, E. Bhimireddy, B. Sridhar, and D. K. Mohapatra, Org. Lett., 2012, 14, 1082. CrossRef
46. Non-stereoselective construction of 4,6-diaminolactone intermediate focusing on the synthesis of racemic manzacidins A and C was reported: G. Xiong, M. Wei, Y. Zhou, Y. Li, F. Zang, and Y. Gong, Synthesis, 2011, 3439. CrossRef
47. J. B. Hendrickson, J. Am. Chem. Soc., 1975, 97, 5784; CrossRef T. Gaich and P. S. Baran, J. Org. Chem., 2010, 75, 4657. CrossRef
48. B. M. Trost, Science, 1991, 254, 1471; CrossRef B. M. Trost, Angew. Chem., Int. Ed. Engl., 1995, 34, 259. CrossRef
49. P. A. Wender, M. P. Croatt, and B. Witulski, Tetrahedron, 2006, 62, 7505; CrossRef P. A. Wender, V. A. Verma, T. J. Paxton, and T. H. Pillow, Acc. Chem. Res., 2008, 41, 40; CrossRef P. A. Wender and B. L. Miller, Nature, 2009, 460, 197. CrossRef
50. J. M. Richter, Y. Ishihara, T. Masuda, B. W. Whitefield, T. Llamas, A. Pohajakalio, and P. S. Baran, J. Am. Chem. Soc., 2008, 130, 17938; CrossRef N. Z. Burns, P. S. Baran, and R. W. Hoffmann, Angew. Chem. Int. Ed., 2009, 48, 2854. CrossRef