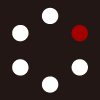
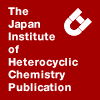
HETEROCYCLES
An International Journal for Reviews and Communications in Heterocyclic ChemistryWeb Edition ISSN: 1881-0942
Published online by The Japan Institute of Heterocyclic Chemistry
e-Journal
Full Text HTML
Received, 23rd July, 2012, Accepted, 26th September, 2012, Published online, 1st October, 2012.
DOI: 10.3987/COM-12-12553
■ Preparation of D-Cycloserine and 13C-Labeled D-Cycloserine
Nathan C. Thacker, Judit Molnár-Tóth, Judy L. Miska, Raul G. Barletta, and James M. Takacs*
Department of Chemistry, University of Nebraska-Lincoln, Lincoln, Nebraska 68588-0304, U.S.A.
Abstract
Tuberculosis (TB) was declared a global emergency in 1993 when an estimated 7–8 million cases were occurring annually causing 1.3–1.6 million deaths.1 More recently, extensively drug-resistant TB strains of Mycobacterium tuberculosis have emerged that are resistant to many of the current treatments.2 M. tuberculosis is characterized by a robust cell wall comprised of a lipid rich structure with a highly cross-linked peptidoglycan backbone.3 D-Alanine enters the pathway of peptidoglycan biosynthesis through the action of D-alanine-D-alanine ligase forming the D-alanyl-D-alanine dipeptide that is subsequently incorporated into a peptidoglycan precursor by the Mur F enzyme. This precursor is incorporated into nascent peptidoglycan by translocation to a lipid intermediate followed by transglycosylation reactions. D-Alanine subunits in the backbone are cross-linked by transpeptidation to meso-D-aminopimelate residues to form the mature peptidoglycan backbone. This backbone gives the cell wall high tensile strength, and thus, inhibiting its formation leads to weaker cell walls and less virulent cells. Finding therapeutic targets that inhibit the D-alanine pathway of peptidoglycan biosynthesis is a highly sought after goal in drug discovery research.4
D-Cycloserine (DCS) is a second stage drug for the treatment of tuberculosis, and while its mechanism of action is not fully understood, DCS is thought to serve as a D-alanine mimic and competitive inhibitor.5 Both D-alanine racemase and D-alanine-D-alanine ligase activities have been shown to be inhibited by DCS in a concentration-dependent manner,6 but it is unclear whether these effects fully account for the bactericidal action of DCS on M. Tuberculosis and other mycobacterial pathogens. In conjunction with studies directed toward gaining a better understanding DCS’s mechanism of action, the synthesis of a specific 13C-labeled DCS, via a route amenable to the preparation of sufficient quantities for metabolic experiments, was needed. DCS has been synthesized a number of times in unlabeled7,8 and isotopically labeled form.9 At the time we initiated our studies, the existing routes were not suitable for our needs, and we embarked on a new synthesis of DCS and 13C-labeled DCS from DL-serine methyl ester. In the interim, Kim published elegant communications of their efforts in which DCS is prepared via a similar route.10 Herein, we report the full details of our synthesis, which unlike that of Kim’s route, starts from racemic serine and gives both enantiomers of cycloserine via a corrected and improved one pot resolution procedure using D- and L-tartaric acids.
DL-Serine methyl ester hydrochloride (1) was treated with trityl chloride to afford the N-trityl-protected amino acid 2 in 99% yield (Scheme 1).11 Cherney and Wang12 reported that a bulky nitrogen protecting group is required for efficient reaction of serine and related derivatives under Mitsunobu conditions. The reaction of 2 with diisopropyl azodicarboxylate (DIAD) and N-hydroxysuccinimide proceeds smoothly, forming the protected hydroxylamine derivative 3 in good yield (80%). The remaining transformations of 3 to (±)-cycloserine, that is, double deprotection followed by cyclization, proved challenging and required careful optimization of the reaction conditions at each step.
N-Deprotection of succinimide derivatives is frequently carried out by treating with hydrazine in protic solvents13 or by reaction with methylamine14 at elevated temperature. Treatment of 3 with hydrazine in refluxing EtOH led to competing reaction of the methyl ester; carrying out the reaction at room temperature gives 4 in only 35% yield.15 In contrast, changing the solvent to CH2Cl2 (ambient temperature, 12 h) gives 4 in high yield (98%). The N-trityl group is readily removed by treatment with HCl in diethyl ether; the dihydrochloride salt 5, an intermediate in the original 1957 synthesis of DCS,7 precipitates from solution in quantitative yield. Attempts to cyclize 5 under a much scaled down version of the original 1957 conditions (dissolution in 5.85 M aqueous KOH, 0 °C to room temperature, 1 h) affords little of the desired product (ca. 10%); the high solubility of DCS in water makes isolation difficult. However, limiting the amount of KOH to 3.0 equivalents and running the reaction in aqueous MeOH (2:1 MeOH/H2O, 0 °C to ambient temperature,1.5 h) and then carefully adjusting to pH = 6 with glacial HOAc affords (±)-cycloserine (6) in 51% yield.
In the original 1955 and 1957 reports,7 DCS is said to have been resolved by treating the racemate with an equivalent of D-tartaric acid (aqueous solution, 0 °C). However, in our hands, treating commercial DCS with an equivalent of D-tartaric acid failed to yield crystals. Contrary to the original report, adding a cold aqueous solution of L-tartaric acid to a cold aqueous solution of D-cycloserine results in almost immediate precipitation of the 1:1 salt 7. The salt is partially soluble in cold water, but by carefully controlling the concentration, both the L-tartaric acid-DCS salt (7) and the D-tartaric acid-L-cycloserine salt (8) from (±)-cycloserine (6) can be isolated by the sequential addition of L-tartaric acid, separating the crystals by filtration, and then adding D-tartaric acid to the mother liquor (Scheme 2). Using this protocol, 7 and 8 are obtained in 82% and 63% of the theoretical yields, respectively. Tartaric acid is removed by ion exchange chromatography (Amberlite© resin, basic form) eluting with 2% aqueous NH4OH. The aqueous eluents were lyophilized to give D- and L-cycloserine (9 and 10), which were recrystallized from 1:1 EtOH/i-PrOH (adjusted to pH 6.0 with glacial HOAc, 0 °C) in 65 and 64% yield, respectively.
In summary, D-cycloserine (DCS, 9) was prepared in overall 19.8% yield and L-cycloserine (10) in 15.2% yield from the hydrochloride salt of (±)-serine methyl ester. Key features of the synthesis include the use of DIAD for the Mitsunobu reaction of the trityl-protected serine methyl ester with N-hydroxysuccinimide and the use of mild conditions for N-deprotection of the succinimide moiety in the presence of the methyl ester. The formation of the L-tartaric acid-D-cycloserine salt (7) and the D-tartaric acid-L-cycloserine salt (9) from (±)-cycloserine (6) via a one-pot procedure corrects an apparent long-standing error in the literature which indicates that the resolution of DCS is accomplished using D-tartaric acid. Via the route described, 250 mg of commercially available DL-serine-1-13C afforded DCS 13C-labeled in the 1-position (16.4% overall yield); yields for each step are comparable to those obtained for the unlabeled material (See SI for characterization).
EXPERIMENTAL
Reactions were carried out under a dry nitrogen atmosphere. Dichloromethane (CH2Cl2), tetrahydrofuran (THF), and methanol (MeOH) were freshly distilled under the following conditions: DCM from calcium hydride, THF from sodium metal and benzophenone, and MeOH from Mg. All synthesized compounds were purified using flash chromatography with the indicated solvents on Silica Gel 60 Geduran®. Thin layer chromatography analyses were performed on Silica Gel HLF (250 microns) precoated analytical plates and visualized with use of handheld short wavelength UV light, vanillin stain (ethanol, H2SO4, and vanillin), or ninhydrin stain (ethanol, acetic acid, and ninhydrin). NMR spectra were recorded on a 400 MHz spectrometer using CHCl3 (δ 7.27 ppm), CDCl3 (δ 77.0 ppm), DMSO (δ 2.54 ppm), or d6-DMSO (δ 40.45 ppm). Peaks are expressed as m (unresolved multiplet), q (quartet), t (triplet), d (doublet), s (singlet), bs (broad singlet). IR spectra were recorded by FT-IR using the ATR technique (ZnSe). Optical rotations were measured in solutions, 1.0 g/100 mL H2O unless indicated otherwise.
DL-Serine methyl ester hydrochloride (1).16 To a cooled (0 °C) solution of DL-serine (250 mg, 2.38 mmol) in MeOH (2 mL) was added dropwise SOCl2 (0.17 mL, 2.4 mmol). The solution was stirred for 16 h at room temperature, at which point it was concentrated with rotary evaporation. Hexanes (3 mL) were added to the resulting oil and the solution was concentrated. The resulting solid was then recrystallized from MeOH/Et2O to afford 1 (352 mg, 95%) as a white crystalline solid: mp 124–126 °C (decomp.); 1H NMR (400 MHz, DMSO-d6) δ 8.64 (3H, bs), 5.64 (1H, s), 4.08 (1H, t, J = 7 Hz), 3.83 (2H, s), 3.74 (3H, s); 13C NMR (100 MHz, DMSO-d6) δ 168.9, 59.9, 54.8, 53.2; IR (ATR) 3381 (OH stretch), 3023 (N-H stretch), 1736 (CO2CH3 stretch), 1499 (C-H bend), 1245 (C-N stretch) cm-1
DL-Methyl 3-hydroxy-2-(tritylamino)propanoate (2).17 To a cooled (0 °C) solution of 1 (350 mg, 2.25 mmol) in CH2Cl2 (10 mL) was added triethylamine (0.69 mL, 4.9 mmol) dropwise over the course of 5 min. Triphenylmethyl chloride (659 mg, 2.36 mmol) in CH2Cl2 (2.5 mL) was then added over the course of 15 min. The resulting cold reaction mixture was stirred (2 h), and then warmed to room temperature overnight. The resulting mixture was washed (1 x 10 mL aq. NaHCO3) and the latter back-extracted with CH2Cl2 (3 x 15 mL). The combined organic extracts were washed with brine (1 x 5 mL) and then dried (MgSO4), filtered and concentrated yielding a white solid. This solid was recrystallized from EtOAc/hexanes and dried under high vacuum affording 2 (804 mg, 99%) as a white powder-like solid: mp 135–137 °C; TLC analysis (30:70 EtOAc/hexanes) showed one spot at Rf 0.3; 1H NMR (400 MHz, CDCl3) δ 7.56–7.53 (6H, m), 7.34–7.25 (6H, m), 7.24–7.22 (3H, m), 3.79–3.75 (1H, m), 3.65–3.57 (2H, m), 3.33 (3H, s); 13C NMR (100 MHz, CDCl3) δ 174.0, 145.7, 128.8, 128.0, 126.7, 71.00, 65.0, 57.9, 52.0; IR (ATR) 3465 (OH stretch), 3309 (N-H stretch), 1720 (CO2CH3 stretch) cm-1.
DL-O-(1,3-Dihydro-2,5-dioxo-1-pyrolidinyl)-N-(triphenylmethyl) methyl ester (3). A solution containing PPh3 (580 mg, 2.22 mmol), N-hydroxysuccinimide (255 mg, 2.22 mmol), and 2 (800 mg, 2.22 mmol) in THF (10 mL) was prepared. The reaction mixture was purged with N2 three times. The reactants were stirred for 10 min to dissolve most of the solid (some solid was not dissolved). The reaction was cooled to 0 °C, and DIAD (0.46 mL, 2.22 mmol) was added dropwise over 3 min. The reaction was allowed to warm to room temperature and stirred overnight. The crude reaction mixture was concentrated under reduced pressure. Flash chromatography on silica gel (20:80 acetone/hexanes) afforded 3 (813 mg, 80%) as a white powder-like solid: mp 156–159 °C; TLC analysis (30:70 acetone/hexanes) showed one spot at Rf 0.30; 1H NMR (400 MHz, CDCl3) δ 7.56–7.55 (6H, m), 7.31–7.27 (6H, m), 7.23–7.22 (3H, m), 4.42 and 4.01 (2H, dd, J1 = 9.1 Hz, 4.0 Hz, J2 = 9.1 Hz, 5.8 Hz), 3.70–3.65 (1H, m), 3.36 (3H, s), 3.12 (1H, d, J = 10 Hz), 2.64 (4H, s); 13C NMR (100 MHz, CDCl3) 172.5, 170. 6, 145.6, 128.8, 128.0, 126.6, 79.0, 71.2, 55.7, 52.2, 25.4; IR (ATR) 3658 (N-H stretch), 2977 (C-H aromatic stretch), 1722 (CO2CH3 stretch), cm-1; HRMS (ESI) calcd. for C27H26N2O5 (M+H): 459.1920, found 459.1914 m/z.
DL-Methyl-γ-Aminooxy-α-amino(triphenylmethyl)butyrate (4). To a cooled (0 °C) solution of 3 (810 mg, 1.77 mmol) in CH2Cl2 (5 mL) was slowly added anhydrous hydrazine (0.16 mL, 5.09 mmol). The resulting solution was stirred at 0 °C for an additional 30 min and then allowed to warm to room temperature overnight. The reaction mixture was filtered through Celite® and concentrated. Flash chromatography on silica gel (3:97 MeOH/CH2Cl2) afforded 4 (664 mg, 98%) as a colorless oil: TLC analysis (8:92 MeOH/CH2Cl2) showed one spot at Rf 0.35; 1H NMR (400 MHz, CDCl3) δ 7.64–7.62 (6H, m), 7.36–7.33 (6H, m), 7.27–7.24 (3H, m), 5.50 (2H, s), 4.11 (1H, dd, J = 10.2 Hz, 4.8 Hz), 3.84–3.77 (1H, d overlapping with 1H), 3.29 (3H, s), 2.89 (1H, d, J = 10.1 Hz); 13C NMR (100 MHz, CDCl3) δ 174.4, 146.0, 128.9, 128.0, 126.7, 78.1, 71.0, 55.8, 51.8; IR (ATR) 3313 (N-H stretch), 3056 (C-H aromatic stretch), 1726 (CO2CH3 stretch) cm-1; HRMS (ESI) calcd. for C23H24N2O3 (M+Na):399.1685, found 399.1695 m/z.
DL-β-Aminoxyalanine methyl ester dihydrochloride (5). To a cooled (0 °C) solution of 4 (658 mg, 1.75 mmol) in THF (5 mL) was added dropwise 3.0 M HCl in Et2O (2.62 mL, 7.87 mmol). The resulting mixture was allowed to warm to room temperature and stirred overnight. The reaction mixture was filtered and the precipitate was washed with THF (4 x 10 mL THF) and then dried under vacuum affording 5 (358 mg, 99%) as a white fluffy solid: mp 144–146 °C (decomp.); 1H NMR (400 MHz, DMSO-d6) δ 11.80–7.80 (5H, bs), 4.61–4.59 (1H, m), 4.56–4.47 (2H, m), 3.76 (3H, s); 13C NMR (100 MHz, DMSO-d6) δ 164.3, 71.7, 53.7, 51.3; IR (ATR) 2848 (N-H stretch), 2639 (ON-H stretch), 1740 (CO2CH3 stretch) cm-1; HRMS (ESI) calcd. for C4H11N2O3 (M+H): 135.0770, found 135.0769 m/z.
DL-Cycloserine (6). To a cooled (0 °C), stirred solution of 5 (350 mg, 1.69 mmol) in MeOH/water (0.5 mL/0.1 mL) was added KOH (332 mg, 5.92 mmol) in water (0.4 mL) dropwise over a period of 10 min. The pH was carefully monitored and kept between 11.0-11.5. The solution was stirred for 30 min at 0 °C followed by 30 min at room temperature. The reaction solution was re-cooled (0 °C) and 2 mL of a mixture of i-PrOH/EtOH (50:50) was added. After approximately 10 minutes the resulting mixtures was filtered and the separated solids washed with a mixture of cold i-PrOH/EtOH (1:1, 0.5 mL). The filtrate was cooled (0 °C) and the pH of the solution was adjusted to 6.0 by the addition of glacial HOAc. Precipitation occurred over 30 min at 0 °C once the pH reached 6.0. The supernatant was removed and the remaining precipitate was washed sequentially with cold i-PrOH/EtOH (50:50, 1 x 0.5 mL) and cold Et2O (1 x 1 mL). The solid was dried under high vacuum yielding 6 (88.0 mg, 51%) as a white powder: mp 146–149 °C (decomp.); 1H NMR (400 MHz, DMSO-d6) δ 5.50–4.75 (3H, bs), 4.46–4.39 (1H, m), 3.79–3.72 (2H, m); 13C NMR (100 MHz, DMSO-d6) δ 174. 9, 75.5, 54.1; IR (ATR) 3399 (NH3 stretch), 1536 (C-O stretch) cm-1.
D-Cycloserine-L-Tartrate (7) and L-Cycloserine-D-Tartrate (8). To a cooled (0 °C) solution of 6 (80.0 mg, 0.784 mmol) in H2O (0.3 mL) was added L-tartaric acid (118 mg, 0.784 mmol) in H2O (0.2 mL) resulting in the immediate precipitation of a white solid. After stirring for 30 min (0 °C) the supernatant liquid was transferred to a clean vial and the crystalline precipitate washed sequentially with cold water (1 x 1 mL) followed by cold acetone (1 x 1 mL). The solid was dried under high vacuum affording D-cycloserine-L-tartrate 7 (82.0 mg, 82%) as a white crystalline powder: mp 158–160 °C (decomp.); [α]D20 +43.2 (c 0.7, H2O); 1H NMR (400 MHz, DMSO-d6) δ 8.48–6.83 (7H, bs), 4.49 (1H, t, J = 8.08 Hz), 4.17 (2H, s), 3.99 and 3.90 (2H, dd, J1 = 9.7 Hz, 7.9 Hz, J2 = 9.7 Hz, 8.3 Hz); 13C NMR (100 MHz, DMSO-d6) δ 174.34, 170.95, 73.38, 52.73; IR (ATR) 3322 (N-H stretch), 3036 (OH stretch), 2786 (CO2H stretch), 1683 (C=O stretch) cm-1.
The supernatant was cooled (0 °C) and D-tartaric acid (80.0 mg, 0.784 mmol) added. The resulting mixture was stirred for 30 min (0 °C), and then cold acetone (1 x 1 mL) was added resulting in the slow formation of a white precipitate. The precipitate was washed sequentially with cold water (1 x 1 mL) followed by cold acetone (1 x 1 mL) and then dried under vacuum affording L-cycloserine-D-tartrate 8 (62.2 mg, 63%) as a white crystalline solid: mp 158–160 °C (decomp.); [α]D20 - 42.8 (c 0.7, H2O); 1H NMR (400 MHz, DMSO-d6) δ 8.48–6.83 (7H, bs), 4.49 (1H, t, J = 8.08 Hz), 4.17 (1H, s), 3.99 and 3.90 (2H, dd, J1 = 9.7 Hz, 7.9 Hz, J2 = 9.7 Hz, 8.3 Hz); 13C NMR (100 MHz, DMSO-d6) δ 174.3, 171.0, 73.4, 72.5, 52.7; IR (ATR) 3322 (N-H stretch), 3036 (OH stretch), 2786 (CO2H stretch), 1683 (C=O stretch) cm-1.
D-Cycloserine (9). L-Tartrate salt 7 (75.0 mg, 0.297 mmol) was dissolved in water (0.5 mL) and filtered through a short column of Amberlite® IR-120 PLUS ion exchange resin (ca 1.0 g, sodium form). The column was washed with water (ca. 2.0 mL) until the effluent tested negative to ninhydrin stain. D-Cycloserine 9 was then eluted with 2% aqueous NH4OH (ca 10 mL) until the effluent tested negative to ninhydrin stain. The latter eluent was lyophilized, and the resulting white flaky residue dissolved in 4% aqueous NH4OH (0.1 mL) and a mixture of i-PrOH/EtOH (50:50, 1.0 mL). The resulting solution was cooled (0 °C) and glacial HOAc added dropwise until the solution reached pH 6; a precipitate formed over the course of ca. 30 min. The precipitate was washed with Et2O and dried affording D-Cycloserine (9) (19.7 mg, 65%) as a white powder: mp 146–149 °C (decomp.); [α]D20 +120.3° (c 1.0, H2O) (literature7 α]D20 +112 (c 1.0, H2O)); 1H NMR (400 MHz, DMSO-d6) δ 5.50–4.75 (3H, bs), 4.46–4.39 (1H, m), 3.79–3.72 (2H, m); 13C NMR (100 MHz, DMSO-d6) δ 174. 9, 75.5, 54.1; IR (ATR) 3040 (NH3 stretch), 1528 (C-O stretch) cm-1.
L-Cycloserine (10). D-Tartrate salt 8 (58.0 mg, 0.23 mmol) was converted to L-cycloserine (10) using the procedure described above giving 10 (15.3 mg, 64%) as a white powder: mp 146–149 °C (decomp.); [α]D20 -118.4 (c 1.0, H2O).
ACKNOWLEDGEMENTS
Support for this work was from a seed pilot grant (RGB, PI) from the University of Nebraska-Lincoln Redox Biology Center (parent grant #NCRR 2P2ORR 017675, D. Becker, P.I.) and the Nebraska Research Initiative is gratefully acknowledged. We thank R. Powers and S. Halouska (UNL Chemistry) for helpful discussions and the NSF (CHE-0091975, MRI-0079750) and NIH (SIG-1-510-RR-06307) for the NMR spectrometers used in these studies carried out in facilities renovated under NIH RR016544.
References
1. WHO. 2011: Global Tuberculosis Control. WHO/HTM/TB/2011.16. www.who.int.tb/publications/global_report/2011/gtbr11_full.pdf (5 December 2011).
2. WHO. Multidrug and Extensively Drug Resistant TB (M/XDR-TB) 2010 Global Report on Surveillance and Response. 2010. WHO/HTM/TB/2010.3. http://whiqlibdoc.who.int/publications/ 2010/9789241599191_eng.pdf (5 December 2011).
3. C. F. Neuahus, J. Biol. Chem., 1962, 237, 3128.
4. P. E. A. Da Silva and J. C. Palomino, J. Antimicrob. Chemother., 2011, 66, 1417. CrossRef
5. J. B. Bruning, A. C. Murillo, O. Chacon, R. G. Barletta, and J. C. Sacchettini, Antimicrob. Agents Chemother., 2011, 55, 291. CrossRef
6. N. E. Caceres, N. B. Harris, J. F. Wellehan, Z. Feng, V. Kapur, and R. G. Barletta, J. Bacteriol., 1997, 179, 5046; O. Chacon, L. E. Bermudez, D. K. Zinniel, H. K. Chahel, R. J. Fenton, Z. Fent, K. Hanfor, L. G. Adams, and R. G. Barletta, Microbiology, 2009, 155, 1440; CrossRef Z. Feng and R. G. Barletta, Antimicrob. Agents Chemother., 2003, 47, 283; CrossRef S. Halouska, O. Chacon, R. J. Fenton, D. Zinniel, R. G. Barletta, and R. Powers, J. Proteome Res., 2007, 6, 4608; CrossRef U. Strych, R. L. Penland, M. Jimenez, K. L. Krause, and M. J. Benedik, FEMS Microbiol. Lett., 2001, 196, 93. CrossRef
7. C. H. Stammer, N. H. Wilson, F. W. Holly, and K. Folkers, J. Am. Chem. Soc., 1955, 77, 2346; CrossRef C. H. Stammer, A. N. Wilson, C. F. Spencer, F. W. Bachelor, F. W. Holly, and K. Folkers, J. Am. Chem. Soc., 1957, 79, 3236. CrossRef
8. X. Li, X. Meng, H. Duan, L. Wang, S. Wang, Y. Zhang, and D. Qin, Arch. Parm. Chem. Life. Sci., 2010, 343, 473; CrossRef H. Bretschneider and W. Vetter, Monatsh. Chem., 1959, 90, 799; CrossRef P. A. Platter, A. Boller, H. Frick, A. Furst, B. Hegedus, H. Kirchensteiner, S. Majnoni, R. Schlapfer, and H. Spiegelberg, Helv. Chim. Acta, 1957, 40, 1531. CrossRef
9. B. Chutney, A. Habersbergerova, P. Kourim, J. Kucera, J. Moravek, O. Pitak, J. Urban, and J. Zikmund, Jaderna Energie, 1958, 4, 392; P. Kourim and J. Zikmund, Collect. Czech. Chem. Commun., 1961, 26, 717.
10. H. K. Kim and K. J. J. Park, Tetrahedron Lett., 2012, 53, 4090H. K. Kim and K. J. J. Park, Tetrahedron Lett., 2012, 53, 1668; CrossRef H. K. Kim and K. J. J. Park, Tetrahedron Lett., 2012, 53, 4090. CrossRef
11. H. Liu, V. R. Pattabiraman, and J. C. Vederas, Org. Lett., 2007, 9, 4211. CrossRef
12. R. J. Cherney and L. Wang, J. Org. Chem., 1996, 61, 2544. CrossRef
13. S. Wolfe, M. C. Wilson, M. H. Cheng, G. V. Shustov, and C. I. Akuche, Can. J. Chem., 2003, 81, 937; CrossRef K. R. Falck, R. Manne, K. R. Atcha, N. Pulli, N. Dubasi, V. L. Manthati, J. H. Capdevila, X. Y. Yi, D. H. Goldman, C. Morisseau, B. D. Hammock, and W. B. Campbell, J. Med. Chem., 2009, 52, 5069. CrossRef
14. K. A. Winans and C. R. Bertozzi, Chemistry and Biology, 2002, 9, 113. CrossRef
15. The major product from the reaction was the ring closed cycloserine with the trityl group intact. However, we found it difficult to remove the trityl protecting group once the ring was closed.
16. B. M. Trost and M. T. Rudd, Org. Lett., 2003, 5, 4599. CrossRef
17. B. McKeever and G. Pattenden, Tetrahedron, 2003, 59, 2701. CrossRef