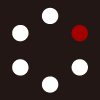
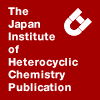
HETEROCYCLES
An International Journal for Reviews and Communications in Heterocyclic ChemistryWeb Edition ISSN: 1881-0942
Published online by The Japan Institute of Heterocyclic Chemistry
e-Journal
Full Text HTML
Received, 3rd August, 2012, Accepted, 31st August, 2012, Published online, 5th September, 2012.
DOI: 10.3987/REV-12-748
■ Aziridine-2-carboxylates: Preparation, Nucleophilic Ring Opening, and Ring Expansion
Tsutomu Ishikawa*
Graduate School of Pharmaceutical Science, Chiba University, 1-8-1 Inohana, Chuo, Chiba, Japan
Abstract
Preparation methods of aziridine-2-carboxylates are discussed. The chemical reactivities, focusing on ring opening by various nucleophiles and ring expansion to larger heterocycles, are also surveyed.INTRODUCTION
Aziridines are the smallest nitrogen-containing heterocycles and are susceptible to ring-opening reactions by the inherent strain (26-27 kcal/mol) due to three-membered ring system. Thus, aziridines play an important role as versatile synthetic intermediates in the synthesis of biologically active nitrogen-containing compounds. In particular, nucleophilic ring opening of aziridine-2-carboxylates (aziridinecarboxylates) has been a common strategy used for the synthesis of α- and β-amino acids. Aziridines, the ring nitrogen of which is activated by an electron-withdrawing group (EWG) such as benzyloxycarbonyl (Cbz) and p-toluenesulfonyl (Ts), react more rapidly than non-activated, N-alkylated or N–unsubstituted aziridines.1 It is well known that heteroatom nucleophiles attack the β-carbon (C3) giving rise to α-amino acids.2 With the exception of nucleophilic Wittig reagents3 and indoles,4 which attack regioselectively at the β-carbon, carbon nucleophiles generally attack in a non-regioselective manner.5 An additional problem with carbon nucleophiles is that the ester functionality is also subject to nucleophilic attack, thereby limiting this method further. Hydrolysis of the ester to the corresponding acid has been shown to correct this problem, and gives rise to regioselective attack at the β-carbon of the aziridine.6 When enantiopure aziridines are required as synthetic intermediates, asymmetric synthesis could, as expected, be applied for the preparation of aziridines. They are also prepared from chiral pool available materials (amino acids,5b,7 sugars8) or from other readily available enantiopure starting materials, especially epoxides.9 Furthermore, lipase-mediated stereoselective transesterification10 of aziridinecarboxylates has been developed. Excellent and exhaustive reviews2,11 have discussed on the syntheses and ring opening reactions of aziridine derivatives. Here, we will survey the reports for the preparation of aziridinecarboxylates and their ring manipulation such as nucleophilic ring opening and ring expansion to 5-membered heterocycles, including literatures appeared after 2004. However, the syntheses by the use of natural source-derived materials, enzymatic transformations, and the hydrogenation of azirine, in addition to chemical modification of the ester function12 and cis-trans ring isomerization,13 are excluded because of limited pages. Reaction examples with asymmetric version are basically cited in schemes of this review, and in non-asymmetric cases the scheme caption is marked with asterisk.
I. PREPARATION
Synthetic approaches to aziridinecarboxylates based on bond connection for the construction of aziridine ring system are schematically illustrated in Scheme 1, in which each three types of intramolecular nucleophilic displacement reactions and addition reactions could be nominated.
The former displacement reaction involves an attack of a nucleophilic nitrogen atom on an adjacent carbon atom bearing a leaving group (X) to afford the aziridine ring system. The cyclization of hydroxyamino acids (method I or II) and the well-known Gabriel-Cromwell method (method II)14 are examples of this approach. In the asymmetric version, it is modified with the use of chiral auxiliaries such as camphorsultam14c or imidazolidin-2-one.14d The aza-Darzens reaction (method II) of N-bromoacetylcamphorsultam has also been used and gives high stereoselectivities.15 The steps of the formation of a stabilized carbanion and of ring closure on an electrophilic nitrogen carrying a good leaving group (X) are involved as an alternative intramolecular nucleophilic displacement via C2-N bond connection, known as aza-Michael reaction (method III). Insertion of an electron-deficient sextet atom (nitrene or carbene) to a double bond is nominated as the latter addition reaction (methods IV - VI) and, in the asymmetric version, nitrene addition to α,β-unsaturated acid derivatives (method IV) bearing a chiral auxiliary16 has been explored.
I-1. By Intramolecular Nucleophilic Displacement Reaction
I-1-1. From Amino Alcohols (Method I or II): A conceptually obvious synthesis of aziridines utilizes 1,2-amino alcohol precursors: the reaction can be readily achieved when the hydroxyl functional group is converted to a good leaving group. Intramolecular nucleophilic displacement reaction by either the amide anion or the amine lone pair provides the aziridine ring. The reaction is generally stereospecific and relies upon the ability of the amine and leaving group to adopt a trans coplanar relationship. Treatment of an amino alcohol with triphenylphosphine (Ph3P) and either bromine (Br2), carbon tetrachloride (CCl4), or diethyl diazocarboxylate (DEAD) has widely been employed to effect aziridine ring closure.2
Optically active, non-activated, benzyl (Bn) aziridinecarboxylates with N-trityl (Tr) group was prepared by treatment of the Bn-esters of N-Tr-(S)-serine or N-Tr-(S)-threonine with sulfuryl chloride and triethylamine (Et3N) at -50 °C.17 It has been concluded that a bulky substituent on nitrogen and a non-sterically hindered carboxylate protecting group are essential for favorable cyclization to occur, affording the required aziridines in good yield (Scheme 2).
Activated N-Ts-aziridinecarboxylates were also prepared from N-Ts-(S)-serinate or N-Ts-(S)-threoninate, p-toluenesulfonyl chloride (TsCl), and potassium carbonate (K2CO3), under phase-transfer catalyst conditions. The same methodology, when applied to the N-Ts-(S)-serine amide, afforded the corresponding newly prepared aziridinecarboxamide, as an enantiopure compound.18
Unique non-activated 3-arylaziridinecarboxylate formation from guanidinium salts involving an ester function and aromatic aldehydes under basic conditions has been discovered by our group, in which tetramethylguanidine (TMG) or sodium hydride (NaH) serve effectively as a base.19 In the reaction urea derivatives are recovered as re-usable precursors for the starting guanidinium salts. This aziridination reaction is applicable to asymmetric synthesis when chiral guanidinium salt derived from 1,2-diphenylethylenediamine is used and, generally, high diastereo- and enantioselectivity are allowed in the products derived from electron-rich aromatic aldehydes. (2R)-Excess aziridinecarboxylate is formed from the (S,S)-guanidinium salt. For example, trans-N-Bn-3-(3,4-methylenedioxyphenyl)-aziridinecarboxylate was afforded in 82% yield with 97% ee when piperonal was used as an electrophile (Scheme 3). α,β-Unsaturated aldehydes can also be used as electrophiles.20 This aziridination may be composed of three steps: (i) nucleophilic addition of guanidinium ylides, derivable from guanidinium salts by treatment with base, to aldehydes, (ii) formation of an imidazolidine-oxazolidine spiro intermediate by attack of the alkoxide ion formed to the guanidinium carbon, and (iii) fragmentation of the spiro intermediate to aziridine and urea components. Plausible mechanisms, dependent upon the electronic character of aldehyde electrophiles, have been proposed based on systematic reactions using a series of p-substituted benzaldehydes.21 This aziridination can be classified to method I because the spiro intermediate is equivalent to an activated form of amino alcohol.
I-1-2. From Epoxides (Azido Alcohol) (Method I or II): The regiospecific ring opening of epoxides by azide ion could lead to the synthesis of aziridines.22 Reduction of the azide moiety in azido alcohol formed, for example with Ph3P in the Staudinger reaction,1c,8b,23 yields first an imino phosphorane and then an oxazaphosphorine intermediate which are normally not isolated prior to thermally induced cyclization to yield an aziridine. The method was applied to the synthesis of possible isomers of aziridine-2,3-dicarboxylate as a chiral version24 (Scheme 4).
In the asymmetric synthesis of oseltamivir phosphate (Tamiflu) from (-)-shikimic acid, aziridination by intramolecular nucleophilic displacement of azido alcohol derivative was applied25 (Scheme 5). Aziridine was prepared in 88% yield by successive treatment of 2-azidocyclohexenyl methanesulfonate with Ph3P, Et3N, and some water in tetrahydrofuran (THF). In this transformation, reduction of the diazo group and formation of the aziridine via an intramolecular nucleophilic substitution of the neighboring methanesulfonyloxy (MsO) group took place simultaneously, and meanwhile the (R)-configuration is inverted to the (S)-one.
I-1-3. From Haloamines (Gabriel-Cromwell Reaction: Method II): Gabriel26 demonstrated the utility of haloamines for aziridine synthesis. 1,2-Dibromoalkanes, prepared via addition of bromine to alkenes, can be elaborated to yield racemic aziridines on treatment with amines.27 trans-Methyl N-Ts-3-arylaziridinecarboxylates and its nosyl (Ns) derivatives were easily synthesized by mixing alkyl cinnamate-derived haloamines with K2CO3 in acetonitrile (MeCN) at room temperature. Good to excellent yields have been achieved (83-97% for the N-Ts- and 75-94% for the N-Ns-derivatives).28 An asymmetric Gabriel-Cromwell reaction of primary amine with enantiopure 2-bromocarboxamides, leading to non-activated aziridines, has been described; best results were obtained utilizing camphorsultam as a stoichiometric chiral controller14c,29 (Scheme 6).
A similar stoichiometric reagent-controlled strategy utilizes a chiral imidazolidin-2-one auxiliary in place of camphorsultam to prepare 1H-aziridinecarboxylates14d,30 (Scheme 7).
The Wittig olefination of α-D-xylo-pentodialdose, derived from D-glucose, using ethyl bromo(triphenylphosphino)acetate in dichloromethane (CH2Cl2) afforded the required bromocarboxylate in 77% yield. The exclusive formation of the E-isomer was confirmed by 1H NMR spectra. Treatment with benzylamine provided aziridinecarboxylate in 70% yield31 (Scheme 8). The trans-geometry of aziridine product was also established by 1H NMR analysis which showed a small vicinal coupling constant between C2-H and C3-H (J5,6 = 2.9 Hz) against the large Jvic (ca 7.0 Hz) known for the cis-aziridine derivative.32 The trans-(2S,3S)-configuration was completely established by the single crystal X-ray analysis and reasonably deduced by the Re-face attack of benzylamine at the prochiral β-carbon of the ester function.
The amino group of alkyl aminoacrylate, derived from the corresponding bromoacrylates by aminolysis, was protected by treatment with aqueous hydrobromic acid in CH2Cl2 to the corresponding hydrobromide salts which were subsequently treated with bromine. After bromination, neutralization of the reaction mixture with sodium hydrogen carbonate (NaHCO3) afforded dibromoaminopropanoates as precursors for Gabriel-Cromwell reaction. Conversion of the dibromo-amines to the aziridines as major products in moderate yields, together with the azetidines as minor compounds, was achieved under conditions with K2CO3 in MeCN at 60 °C33 (Scheme 9). Interestingly, bromination of the tosylaminoacrylate (R2 = Ts in Scheme 9) proceeded uneventfully without pre-treatment with hydrobromic acid and post-treatment with NaHCO3. The dibromopropanoate was efficiently and selectively cyclized to N-Ts-aziridine (95% yield).
An attempt was also made to perform the bromination and cyclization in a one-pot reaction.33 Bromination of 2-[(1-phenylethylamino)methyl]acrylate was subjected to the conditions in Scheme 9, after which CH2Cl2 was replaced by MeCN. Upon adding K2CO3, a selective cyclization of the intermediate hydrobromide salt occurred to yield aziridine in an excellent overall yield (81%) (Scheme 10).
(2S,3R)-3-Arylaziridinecarboxamides and (2S,3R)-3-arylaziridinecarboxylates have asymmetrically prepared from (Rs)-t-butanesulfinamide as a chiral source by the application of Gabriel-Cromwell reaction34 (Scheme 11). Condensation of (Rs)-t-butanesulfinamide and excess of 2-chloro-1,1,1-trimethoxyethane in the presence of a catalytic amount of p-toluenesulfonic acid (TsOH) without solvent at 100 °C for 4 h afforded (Rs)-α-chloro-N-(t-butanesulfinyl) imidate in 84% yield. The addition reaction of the imidate to aldimine was optimized by systematically changing the reaction
conditions in the synthesis of adducts, α-chloro-β-(sulfonylamino)sulfinyl imidates, and finally the use of lithium hexamethyldisilazide (LHMDS) as a base led to the isolation of the optically pure (Rs,R,R)-adduct imidate (Ar = Ph, R1 = Ts) in 86% yield. Analogously (hetero)aromatic chiral imidates (Ar = 4-MeOC6H4, R1 = Ts and Ar = furan-2-yl, R1 = Bs) were prepared with excellent diastereoselectivity. N-Deprotection of t-butanesulfinyl group on the imidate function in the (Rs,R,R)-adduct imidates was simply achieved by treatment with a 4N solution of anhydrous hydrogen chloride (HCl) in dioxane to yield the corresponding (R,R)-imidate hydrochlorides (Ar = Ph or 4-MeOC6H4, R1 = Ts), which were easily transformed to new chiral (R,R)-α-chloro-β-sulfonylamino amides and esters in excellent yields (94-99%), upon simple heating in chloroform (CHCl3) and hydrolysis, respectively. The HCl-promoted N-deprotection of the adduct imidate (Ar = furan-2-yl, R1 = Bs) in dioxane led directly to the corresponding (R,R)-amide in 95% yield, whereas (R,R)-ester was formed in 89% yield by treatment with 3N HCl in methanol (MeOH). (R,R)-Amides and esters could be easily cyclized to the corresponding (2S,3R)-3-arylaziridinecarboxylic amides and esters via addition of K2CO3 in MeCN.
I-1-4. From Imines (Aza-Darzens Reaction: Method II): Aza-Darzens reactions of the chiral enolate derived from bromoacylcamphorsultam with N-(diphenylphosphinyl) imines (R = Ar or t-Bu) were reported as means of asymmetric access to aziridinecarboxylic acid derivatives35 (Scheme 12). Enantiopure cis-aziridinecarboxylates may then be obtained after alcoholysis of these sultams.
Condensation of enantiopure sulfinimines with the lithium enolate of methyl bromoacetate36 or of methyl α-bromopropanoate37 allows entry to cis-N-(p-toluenesulfinyl)aziridinecarboxylates in moderate yields (Scheme 13). Diastereoselectivities of up to 98% in favor of the cis-diastereoisomer are achieved. A chair-like transition state is proposed in which the sulfinyl nitrogen is also coordinated to the lithium cation. Both the enolate and the N-sulfinimine are required to have the E-configuration in the transition state. However, sulfinimines are known to possess relatively low barriers to inversion,38 and it has, therefore, been argued that the lithium cation may coordinate to both the nitrogen and oxygen of the transition state to lock the imine in the necessary E-geometry. Oxidation of the N-sulfinylaziridines with m-chloroperbenzoic acid (mCPBA) readily afforded the N-Ts analogues and, alternatively, the sulfinyl group could be removed under acidic or basic conditions, yielding 1H-aziridines.32c
A chiral auxiliary based approach using tartrate-derived auxiliaries attached to imines has also been reported and a range of cis-aziridines was allowed to prepare on consideration with lithium or zinc enolates.39 It was observed that lithium enolates afford the (2R,3S)-aziridine whereas zinc enolates afford the (2S,3R)-diastereoisomer, presumably as a consequence of the different coordinating abilities of these metals39b (Scheme 14).
The nucleophilic addition of ethyl diazoacetate to imines is one of the most versatile methods for the synthesis of aziridinecarboxylates40 and represents a complementary alternative to nitrene addition to alkenes (method IV) for the synthesis of aziridines. Copper (Cu) salts41 and transition metal salts42 are typically employed to promote the reactions of ethyl diazoacetate and imines. Lewis acids such as methylruthenium trioxide, boron trifluoride etherate (BF3·Et2O), indium chloride (InCl3), and metal triflates, including zinc triflate [Zn(OTf)2] and yttribium triflate [Y(OTf)3], can also be used for the addition ractions.43 In most cases, the products are obtained as a mixture of cis- and trans-aziridines and also the yields and selectivities reported are far from satisfactory. For example, Cu-catalyzed diazoacetate decomposition in the presence of an imine has indicated that the efficiency of the reaction is strongly dependent upon the nature of the imine nitrogen substituent and favor N-aryl substituents.41a A bisoxazoline Cu(I) complex has been known to act as a useful catalyst for the control of stereochemistry in this process;41b however, effectiveness in the aziridine formation was lower than for the racemic process (Scheme 15).
Furthermore, many of these reactions cannot be carried out in a one-pot operation with an aldehyde, amine, and ethyl diazoacetate, because the amines and water that exist during imine formation can decompose or deactivate the Lewis acids. A one-pot procedure was developed for this conversion using lanthanide triflate as a novel catalyst.44 Aldimine (generated in situ from aldehydes and amines) undergo ready addition with ethyl diazoacetate in the presence of a catalytic amount of lithium perchlorate (LiClO4) in MeCN to afford the corresponding cis-aziridinecarboxylates in high yields with high diastereoselectivity45 (Scheme 16).
Wulff’s group has developed a protocol for the 3,3’-diphenyl-2,2’-bi(naphthol) (VANOL)-triphenoxyborane [B(OPh)3]-catalyzed asymmetric aziridination between benzhydryl imines, prepared from aminodiphenylmethane and an aldehyde, and ethyl diazoacetate.46 Scheme 17 illustrates the generality of this protocol over a broad spectrum of benzhydryl imines derived from not only aromatic but also aliphatic aldehydes. The 2,2’-biphenyl-3,3’-(4-biphenanthrol) (VAPOL) catalyst gives essentially the same asymmetric induction. The exemplified benzhydryl aziridines can be readily crystallized up to almost optical purity by single crystallization with good to excellent recovery. Reduction of the amount of catalyst did not result on less than complete conversion for the VANOL catalyst until the loading was reduced to 0.25 mol% and raising the concentration of imine to 1.0 M increased the conversion to 95%.
N-Ts-Imino ester was reacted with (trimethylsilyl)diazomethane (TMSCHN2) in the presence of catalytic 2,2-bis(diphenylphosphino)-1,1’-binaphthalene (BINAP) and bisoxazoline-Cu salts to give cis- and trans-aziridines with moderate enantiomeric excess.47
The proton is arguably the most abundant and least expensive of the Lewis acids. Brønstead acids are also readily removed from an organic reaction-an attractive feature with substantial strategic and practical implications. Brønsted acid catalysis was turned to extend the scope of Lewis acid-catalyzed aza-Darzens reactions48 and to facilitate construction of the more Lewis basic and functionally diverse N-alkylaziridines.49 Screening of various Brønsted acids revealed to be triflic acid (TfOH) as the most effective catalyst for the aza-Darzene reaction: in the addition of diazoacetate to α-imino glyoxalates in the presence of 25 mol% TfOH, cis-aziridinecarboxylates were provided in good yield with excellent selectivity. Aldimines derived from simple aliphatic aldehydes afforded low isolated yields (41-45%) of the cis-aziridine. A dramatic improvement came by employing pyridine-2-carbaldehyde imine, which provided the desired cis-aziridine (R = 2-Py) (90 : 10 ds) in 73% isolated yield49 (Scheme 18).
Aza-Darzens reaction of ethyl diazoacetate with aldimines, derived from phenyl glyoxal, has furnished cis-aziridinecarboxylates with excellent enantioselectivities by means of a chiral phosphoric acid.50
I-1-5. From α,β-Unsaturated Esters (Aza-Michael reaction: Method III): Direct conversion of (E)-2-cyanoacrylates into the aziridine products was achieved by aza-Michael addition of N-protected O-sulfonylhydroxylamine derivatives51 (Scheme 19). 2-Cyanoaziridinecarboxylates were prepared in good yields and with high purity by reaction of the easily obtainable corresponding cyanoacrylates with sulfonyloxycarbamates in the presence of calcium oxide, after only a fast filtration of crude mixtures through plugs of silica gel. The reactivity was found to be influenced by steric hindrance of the R substituents on acrylates.
I-2. By Addition Reaction
I-2-1. From Alkenes (Nitrene Addition: Method IV): Direct aziridination of alkenes by nitrenes is a well-studied reaction particularly using alkoxycarbonylnitrenes. However, there are severe limitations to this method in synthesis arising from competitive insertion into C-H bonds and from conversion of the initially formed singlet state of the nitrene into the triplet state which reacts non-stereospecifically with alkenes. Thus, the addition of free nitrenes to an alkene is generally not well stereochemically-controlled to provide a mixtures of cis- and trans-aziridines52 (Scheme 20).
There are few general methods for highly diastereoselective aziridination of alkenes with the chiral element (chiral center) present in the alkene (substrate-controlled diastereoselectivity)2 and even fewer with the chiral center contained in the reagent (reagent-controlled diastereoselectivity). However, both the α,β-unsaturated ketone bearing Oppolzer’s chiral auxiliary53 and sugar-derived α,β-unsaturated ester54 are aziridinated by oxidative addition of N-aminophthalimide (Pht-NH2) mediated by lead tetraacetate (LTA) with excellent diastereoselectivity (Scheme 21a, b). A similar oxidative addition of the N-aminobenzimidazole (BIM-NH2) to an α-methylene-γ-butyrolactone gave only the aziridine diastereoisomer (reagent-controlled diastereoselectivity) (Scheme 21c). Atkinson and co-workers have explored 3-acetoxyaminoquinazolinone and its analogs as a nitrogen source in asymmetric aziridination through these nitrene additions.11b
It was reported that (N-Ts-imino)phenyliodinane (PhI=NTs) could aziridinate alkenes in the presence of catalytic quantity of low-valent metal complexes.55 A variety of catalysts capable of effecting asymmetric aziridination was also reported.56 These include benzylidne derivative of 1,2-diaminocyclohexane,56a chiral 4,4’-disubstituted bisoxazolines,56b,c and binaphthyl salen-manganese (III) complexes.57 Examples using methyl cinnamate are shown in Scheme 22.
The synthesis of trans-N-Ts-3-arylaziridinecarboxylates from cinnamate was conducted via a one-pot procedure consisting of aminohalogenation and in situ intramolecular SN2 substitution58 (Scheme 23). Et3N was found to be an effective base for the in situ cyclization for most substrates. Moderate to good yields of products and excellent anti-stereoselectivity for trans-aziridine formation were achieved. This method is applicable to only phenyl and EWG-substituted cinnamates, but α,β-unsaturated ketones worked.
Aziridination with good to excellent enantioselectivity was achieved by using 2-(trimethylsilyl)ethanesulfonyl azide as a nitrene precursor in the presence of newly prepared ruthenium salen complex [Ru(salen)(CO)] of high durability59 (Scheme 24). Aziridiantion of less reactive α,β-unsaturated esters (and amides) proceeded with excellent enantioselectivities.
I-2-2. From Imines (Carbenoid Addition: Method V or VI): The alternative [2+1] cycloaddition route to aziridines involves carbenoid addition to imine double bond. Aza-Darzens reaction of imines with diazoesters discussed in I-1-4 section may be categorized to method VI; however, to the best of our knowledge, no reports by methods V and VI are basically available.
II. NUCLEOPHILIC RING OPENING
The nucleophilic ring opening of a 2,3-disubstitued aziridine can deliver two regioisomers, from which, in principle, four products are possible with inversion or retention of configuration2,11 (Scheme 25). In particular, completely stereoselective ring opening almost always arises from inversion of configuration.
The conformational stability and reactivity of the aziridine ring toward nucleophiles are dependent on the nature of the substituent on nitrogen, according to which aziridines can be classified to two groups. The first “non-activated” aziridines contain a basic nitrogen atom and their aziridine ring conformation is more stable and less reactive toward nucleophiles. Thus, ring opening reactions usually occur after protonation, quaternization, or formation of a Lewis acid adduct. The second “activated” aziridines contain a substituent which can conjugatively stabilize the negative charge that develops on the nitrogen atom in the transition state for ring opening by a nucleophile, which is consistent with conformational destabilization of the aziridine ring. Most of ring opening reactions have focused on N-activated aziridines, whereas there have been few reports on the ring opening reactions of N-alkylaziridines.2,11
A general synthesis of α-1-C-substituted derivatives of fagomine (2-deoxynojirimycin-α-C-glycoside) by ring opening reactions of an iminosugar-derived non-activated aziridine with various heteroatom nucleophiles, including alcohol, amine, carboxylate, thiol, and phosphate, has been achieved 60 (Scheme 26).
In this chapter, nucleophilic ring openings by various nucleophiles will be discussed in the order of carbon, hydrogen, and heteroatom nucleophiles.
II-1. With Carbon Nucleophiles
Nucleophilic ring opening of aziridines by organometallic reagents has been known for over four decades.61 However, the application of the carbanion addition was not significantly accelerated until a more efficient method developed in the BF3·Et2O-catalyzed opening of non-activated aziridines by organocuprates was reported.62 A subsequent report for the ring opening of N-sulfonated aziridines with requirement of no catalysis further enhanced its broad use.5a The use of organometallics to open aziridinecarboxylates appears an excellent route for the synthesis of α-amino acids,3 but the competing reaction at the ester functionality is problematic. While organocuprate reagents have obviated this problem, regioselectivity in ring opening was poor.63 Hydrolysis of the ester to an acid, then treatment with higher order cuprates overcome this regioselectivity problem for 3-unsubstituted aziridinecarboxylic acid64 (Scheme 27). In accordance with the expected steric effect of methyl substitution at C2 in aziridine, in situ-prepared cuprates attack the C3 of the t-butyl aziridinecarboxylate with complete control of regioselectivity.
Indoles, containing enamine functionality embedded in the heteroaryl ring, have been found to be good substrates for the Lewis acid-catalyzed ring opening of aziridines.4 2-Substituted indole readily underwent nucleophilic addition to activated aziridinecarboxylate, when facilitated by scandium perchlorate [Sc(ClO4)3], to give 2-substituted tryptophan in good yield65 (Scheme 28), whereas a mixture of regioisomers were obtained when catalyzed by tin triflate [Sn(OTf)3]. Other Lewis acids such as BF3·Et2O, metal triflates [Zn(OTf)2, Yb(OTf)3, and In(OTf)3] and InCl3 were less effective catalysts than Sc(ClO4)3 in the ring opening of the aziridine with respect to regioselectivity and reproducibility.66
We have reported Lewis acid-catalyzed ring opening of non-activated trans-N-Bn-3-arylaziridinecarboxylate using electron-rich aromatic nucleophiles, including indole, which preferentially attack at the benzylic C3 of the starting aziridine with inversion to give 2-amino-3,3-diarylpropanoates13 (Scheme 29).
We also examined ring opening of non-activated 3-arylaziridinecarboxylates using non-aromatic carbon nucleophiles67 (Scheme 30). Effective cyanation (92% yield) with high regio- and stereoselectivity was observed when trans-3-arylaziridinecarboxylate carrying an electron-rich aryl substituent was treated with a freshly prepared diethylaluminum cyanide [Nagata reagent: Et2AlCN] as a cyanide source. Treatment with ketene silyl acetal as an alternative carbon nucleophile in the presence of aluminum chloride (AlCl3) successfully provided the corresponding ring cleaved product, even a pyrrolidone derivative was formed after cyclization. It was found that aluminum-containing Lewis acid catalysts were effective in these ring opening reactions; however, less effective ring opening and no reaction were observed when used the electron-deficient aryl-substituted trans-derivatives and the cis-aziridines, respectively.
II-2. With Hydrogen Nucleophiles
Although hydrogen is not characterized as a nucleophile, it serves the purpose of aziridine ring cleaving.11 Catalytic hydrogenolysis of both activated and non-activated aziridines produces amines as useful building blocks for other synthesis and synthon for the synthesis of biologically active products.36,37,56b,68 Hydrides could undergo ring opening of aziridines; however, hydride reduction requires activation of aziridines for the completion. In addition, hydrogenolysis of aziridines provides the corresponding amines with high regiocontrol, whereas hydride reduction is less appreciable in terms of site of the ring cleavage.
II-2-1. Hydrogenolysis: With 3-arylaziridinecarboxylates, regiospecific hydrogenolysis at the benzylic carbon was observed to give α-amino acid products and such ring opening does not affect the C2 stereochemistry.13,19,69 In the case of (R)-N-[(S)-1-phenylethyl]aziridinecarboxylate, lacking benzylic
aziridine carbon center, hydrogenolysis occurred at C2 to give β-amino ester.70 However, the corresponding (2R,1S’)-aziridine alcohol gave a chiral (2R,1S’)-amino alcohol with C3 opening70,71 (Scheme 31). No reaction occurred with the sterically crowded trityl group on nitrogen.
The catalyst and solvent effects on hydrogenolysis of 3-alkylaziridinecarboxylates have been examined72 (Scheme 32). The optimum results with nearly quantitative yield was obtained by the use of Raney nickel (Ni) in ethanol (EtOH). On the other hand, the 2-methyl derivative yielded two diastereoisomers and the diastereoselectivity was dependent upon the alcohol solvent used. The (2S)-isomer was yielded as a major isomer with retention of configuration in the ring opening reaction of the (2R)-methylaziridine when the more hindered t-butanol (t-BuOH) was used as solvent.
II-2-2. Hydride: The reductive cleavage of 3-phenylaziridinecarboxylate containing peptidemimics using sodium cyanoborohydride (NaCNBH3) under acidic conditions was reported73 (Scheme 33). Ring opened product was regioselectively derived by hydride attack at the benzylic carbon.
Sodium borohydride (NaBH4) was also used for regioselective ring cleavage of N-Ts-aziridinecarboxylates; however, no selectivity was observed in the presence of additional substituent at the C2, resulting in ca 1 : 1 mixture of α- and β-aminopropanoates74 (Scheme 34).
The regiochemistry of ring opening can also be directed towards C2 through chelation of the nucleophile.75 Treatment of (2R,3S)-2-methylaziridinecarboxylate with lithium aluminum hydride (LiAlH4) afforded alkoxide by reduction of the ester function, which directed the delivery of hydride to open the aziridine at C2. (2R,3S)-β-Amino acids were obtained by re-oxidation of the alcoholic group75b (Scheme 35).
II-2-3. Reductive Ring Opening through Electron Transfer: Samarium (II) iodide (SmI2) is subjected to reduction of a number of functional groups due to its single-electron transfer capability. Application to ring opening of carbonyl functionalized aziridine derivatives provides β-amino carbonyl derivatives not only in high yields, but in excellent regioselectivity.76 Use of N,N-dimethylethanolamine (DMEA) as the proton source was recommended for effective ring opening reactions. Reduction of the 2-ketoaziridine required no DMEA as a proton source, whereas reduction of the aziridinecarboxylic esters and amides involved the DMEA additive, a possible ligand to the Sm(II) reductant for the reactivity and rectifier for regioselectivity (Scheme 36). A mechanism, in which a ketyl or a radical species is initially formed and the adjacent C-N bond is cleaved, has been proposed. Although stereochemistry was preserved β to the carbonyl, the stereochemistry at the α position cannot be controlled in this process.
Magnesium in MeOH was employed as the electron transfer reagent in the reduction of 2-cyano-, 2-halomethyl-, and 2-(phenylsulfonylmethyl)aziridines; however, elimination, not ring opening, products were given on the latter two aziridines.77
II-3. With Heteroatom Nucleophiles
The regioselective introduction of a heteroatom nucleophile into enantiopure 2-substituted aziridines makes it possible to synthesize polyfunctional chiral compounds. The reaction of heteroatom nucleophiles with aziridinecarboxylates generally proceeded at C3.
II-3-1. Halogen: Regioselectivity in the ring opening of aziridines by halogen nucleophiles is dependent on the nucleophilic reagent rather than aziridine substrate. Regioselective ring opening at C3 was proceeded for a variety of activated (2S,3R)-3-alkylaziridinecarboxylates by treatment with magnesium bromide (MgBr2), but C2 opening generally predominated when used sodium bromide (NaBr) [or sodium iodide (NaI)] as a halogen source78 (Scheme 37). However, reaction of phenylaziridines under the latter conditions gave a 1 : 1 regiochemical mixture. Removal of the halogen using radical reduction allowed the preparation of either α- or β-amino acid derivatives.
HCl [or hydrogen bromide (HBr)] itself is a source of a proton for activation and of a chloride anion for the ring opening of aziridines. Ring cleavage of a non-activated aziridinecarboxylate smoothly proceed with dry HCl in diethyl ether (Et2O) to give a β-amino ester in excellent yield with exclusive regioselectivity79 (Scheme 38). The use of an aqueous HCl solution in the ring opening of non-activated aziridines was also reported.80
Reaction of N-Boc-2-[(2-ethoxycarbonyl)vinyl]aziridines with lithium chloride (LiCl) or lithium bromide (LiBr) in the presence of Amberlyst-15 led to the effective production of ring cleaved products with high regio- and stereoselectivity.81 Amberlyst-15 can also catalyze the same reaction with lithium iodide (LiI). The reaction proceeded at very mild conditions, but somehow reduced chemical yields of iodo adduct and the formation of oxazolidinone, due to the activity of the iodide anion as a leaving group, were observed (Scheme 39).
Iodo-amines could be produced as ring opened products when non-activated aziridines were treated with trimethylsilyl iodide (TMSI), in which the ring nitrogen was initially activated with the silyl reagent. In contrast to other halide nucleophiles, fewer reports for the ring opening of aziridine with a fluoride anion have been documented.
II-3-2. Nitrogen: Increased activities in methods for ring opening of aziridines with azides have been developed. Most of the reported methodologies are practically useful, and all these provide multiple options with consideration of substrate and product criteria. Sodium azide (NaN3) has traditionally been used as a nitrogen nucleophile in the ring opening of activated aziridines. Trimethylsilyl azide (TMSN3) serves a dual function like TMSI: it activates the basic ring nitrogen and provides a nitrogen source (N3-), which attacks the less substituted C3 of 3-unsubstituted aziridines to give a ring opened product.82 In contrast, a different regioselectivity is observed in the AlCl3-catalyzed reaction of enantiopure aziridinecarboxylate with NaN3 in aqueous EtOH (pH 4), in which N3- selectively attacks the more electron-deficient C2 to regioselectively yield 3-amino-2-azidopropanoate in high yield83 (Scheme 40).
The stereospecific preparation of α,β-diamino-β-(trifluoromethyl)propanoates could be achieved from the N-Ts- and N-Ns-3-(trifluoromethyl)aziridinecarboxylates using amines or NaN3 as nucleophiles without a Lewis acid catalyst.84 Examples of N-Ts-aziridines with amines are shown in Scheme 41.
N-Ts-Aziridines react efficiently with amines in the presence of Montmorillonite K-10 as catalyst under microwave irradiation in solvent-free conditions to yield the corresponding achiral and chiral diamines in a few minutes and in high yields with regio- and stereoselectivity.85 In the case of the 2-methylaziridinecarboxylate, 3-(N-Ts-amino)-2-methyl-2-(phenylamino)propanoate was afforded in 85% yield when treated with aniline for 3 min (Scheme 42).
II-3-3. Oxygen: Aziridines show lower reactivity toward oxygen nucleophiles compared to epoxides. The activation at the ring nitrogen either by attaching EWG and / or on the use of appropriate Lewis acids in oxygen nucleophilic addition is normally needed for the ring opening of aziridines. The ring opening of both activated and non-activated aziridines with a water molecule can be achieved similarly to that with alcohols. The stereochemistry of the addition is generally controlled by anti-attack and the regiochemistry is largely dependent on the reaction conditions used. Steric effects and effects from the functional group in substrates also govern the site of the addition. Proton-promoting ring opening of 3-phenylaziridinecarboxylates preferentially proceeded at the C3 to give β-hydroxyamino acid derivatives. Thus, β-hydroxyphenylalanine was provided in 72% yield as a single stereoisomer by addition of water to the aziridine in the presence of TsOH86 (Scheme 43).
Aziridine ring openings virtually proceed via SN2 mechanism. Treatment of N-Bn-3-(1-pentadecenyl)aziridinecarboxylate with oxygen nucleophiles under conventional conditions, as expected, afforded the ring opened α-amino-β-hydroxy esters with regio- and stereoselectivity.20a Reduction of (2S,3S)-aziridinecarboxylate derived from D-glucose with diisobutylaluminum hydride (DIBAL-H) resulted in the chemoselective formation of aziridine aldehyde. Its two-carbon homologation using a Wittig reagent afforded an inseparable E / Z mixture of (4R,5S)-γ,δ-aziridino-α,β-unsaturated ester in a ratio of 8.5 : 1.5, which was treated with trifluoroacetic acid (TFA) in aqueous MeCN to, as expected, afford a ring opened product, (4S,5S)-δ-amino-γ-hydroxy-α,β-conjugated ester, with regio- and stereoselectivity31b (Scheme 44a). One notable exception was the trifluoroacetic anhydride (TFAA)-induced ring opening of an enantiopure (SR,2R,3R)-N-toluenesulfinyl-3-(1-pentadecenyl)-aziridinecarboxylate.87 Treatment with aqueous TFA gave the expected C3-ring opening product, L-threo-(2R,3S)-sphingosine precursor, but TFAA predominantly affords (2R,3R)-isomer, in the formation of which a [3,3]-stereospecific rearrangement of the activated sulfoxide complex was proposed (Scheme 44b).
In the asymmetric synthesis of oseltamivir phosphate (Tamiflu) from (-)-shikimic acid, aziridination by intramolecular nucleophilic displacement and ring opening reaction of the formed aziridine system with an oxygen nucleophile were applied.25 The aziridine prepared in Scheme 5 was exposed to acetic anhydride and Et3N in ethyl acetate (EtOAc) to form N-acetylaziridine almost quantitatively, which was regio- and stereoselectively ring opened by treatment with BF3·Et2O using pentan-3-ol as solvent to give (3R,4S)-3-(1-ethylpropoxy)-4-acetamidocyclohexene-1-carboxylate in 92% yield (Scheme 45). The reaction temperature is crucial here, it should be kept in the range of -5 °C to 0 °C, and the slow addition of BF3·Et2O was necessary because the reaction is exothermic. In this aziridine ring opening reaction, the nucleophile (pentan-3-ol) approaches the face of the cyclohexene ring opposite to the benzoyloxy (BzO) group at the C5 position. The allylic position (C1) of the aziridine is much more reactive than the non-allylic position (C6), so the Lewis acid-catalyzed nucleophilic attack of pentan-3-ol at allylic position was completely regiospecific.
Other oxygen containing acids such as TsOH88 and phosphoric acid9b themselves were also reported as effective nucleophiles to undergo ring opening of aziridines.
II-3-4. Sulfur: The ring opening reaction of aziridines by thiols can readily proceed in either an activated or a non-activated form and, in general, not only high chemical yields but also high regioselectivity have been observed.89 The ring nitrogen in the non-activated aziridine can serve as a base to abstract a proton from the thiols to form a reactive aziridinium intermediate. The aziridine ring carbon at a less hindered site is then attacked by the deprotonated thiol anion to provide 2-amino sulfide products. On the other hand, activated aziridines, lacking basic nitrogen, often require a Lewis acid for further activation. The highly regioselective ring opening of non-activated 3-(trifluoromethyl)aziridinecarboxylate at the α-carbon of the carboxylate was observed in the thiol addition and the stereoselective anti-attack to the ring yielded the corresponding adducts in high chemical yields79 (Scheme 46).
BF3·Et2O has been widely used as a catalyst in the ring opening of aziridines with thiol nucleophiles; however, at least a stoichiometric amount of BF3·Et2O and excess thiol were requested for practical achievements. Thus, (2S,3S)-β-phenylcysteine derivative was obtained from (2S,3R)-phenylaziridinecarboxylate in 67% yield by treatment with p-methoxybenzyl thiol (3 eq) in the presence of BF3·Et2O (1.5 eq) 90 (Scheme 47).
Although stereoselectivity on the ring opening of activated 3-(trifluoromethyl)aziridinecarboxylate with nitrogen nucleophiles was observed (see, Scheme 41), a 58 : 42 mixture of both diastereoisomers was obtained in 66% yield in the reaction with potassium ethyl xanthate84 (Scheme 48). Lack of diastereoselectivity is probably the result of an isomerization due to the higher acidity of proton, vicinal to the carboxylate and xanthate functions.
II-3-5. Miscellaneous: Phosphites, organosilane anions, and selenols could serve as heteroatom nucleophiles in ring opening of aziridines; however, only limited reports were found in the literature. Direct conversion of aziridines to β-lactams has been realized by carbonylations catalyzed with transition metal cobalt.11f
III. Ring Expansion
The aziridine ring is a valuable synthon for the preparation of larger heterocycles via ring expansion. For aziridinecarboxylates, ring enlargement via the scission of either of the C-N bonds [paths (a) and (b)] or the C-C bond [path (c)] can be programmed (Scheme 49). Examples, including our case,67 of intermolecular [3+2] cycloaddition of azomethine ylide, derived from the path (c) bond scission, with electron-deficient olefins yielding pyrrolidine derivatives have been studied; however, no reports on ring expansions through path (c) have appeared to the best of our knowledge.
III-1. Through Path (a)
Lewis acids promote the rearrangement of acylaziridines into oxazolines and BF3·Et2O is the most widely used Lewis acid catalyst. Both chemical evidence and ab initio calculations have shown that this reaction occurs with retention of configuration at the stereogenic centers.91 N-Acylaziridine-2-carboximides undergo completely regioselective ring expansion to afford oxazolines as the only products92 (Scheme 50).
Semi-empirical calculations suggest that the imidazolidin-2-one chiral auxiliary could be responsible for the completion of reaction and for the regiochemistry.93 The incipient positive charge would presumably be stabilized by a preferred conformation, in which the endocyclic carbonyl oxygen is in proximity of the aziridine C3. Ring expansion and ring opening of aziridine derivatives are complementary. Ring opening of trans-acylaziridines by an oxygen nucleophile affords the anti-amino acids, whereas ring expansion followed by hydrolysis leads to the syn-isomers. Furthermore, (R)- or (S)-amino acids may be isolated dependent on the chiral imidazolidin-2-one auxiliary used.
Optically active N-Boc-aziridinecarboxylate was converted to the corresponding oxazolidin-2-one in quantitative yield with complete regio- and stereoselectivity94 (Scheme 51). Both of the bond breaking and forming processes proceed with the retention of the C3 configuration.
Incorporation of MeCN into an imidazoline skeleton was observed when a solution of N-acetyl (Ac)-aziridinecarboxylate in MeCN was treated with BF3·Et2O88 (Scheme 52).
A new protocol for generating aza-ortho-xylylenes via acid-catalyzed (or fluoride-promoted) ring opening of 3-(2-acylaminophenyl)aziridinecarboxylates, which were prepared by Gabriel-Cromwell method, is explored95 (Scheme 53). The aza-ortho-xylylenes then intercepted with indoles in intramolecular hetero Diels-Alder reactions to give pentacyclic ring systems.
III-2. Through Path (b)
When non-activated aziridinecarboxylate was treated with chloroformates, the ring opening could be initiated by the formation of the aziridinium ion intermediates, which then underwent double nucleophilic addition to form oxazolidinones with high chemical yields96 (Scheme 54). Retention of the configuration was observed because double nucleophilic additions occur at the C2 chiral center of the starting aziridine.
Isocyanates can also serve ring expansion of non-activated aziridinecarboxylates to form imidazolidin-2-ones.97 The treatment of N-[(R)-1-phenylethyl]aziridinecarboxylate with isocyanates afforded 4-functionalized imidazolidin-2-ones in high yields. A plausible mechanism with retention of the configuration at C2 of the aziridine via a double inversion process at the C2 position of the chiral aziridine was proposed97 (see, Scheme 54).
Exposure of N-unsubstituted 3,3-dimethylaziridinecarboxylate to potassium thiocyanate in hot formic acid led to cleanly crystalline thiazoline (94%), indicating that aziridine cleavage in this case followed path (b).98 On the other hand, treatment with benzyl isothiocyanate in benzene at reflux gave the hydantoin derivative in 88% yield. The latter is believed to be formed via transient N-substituted aziridine which undergoes initial acylation by the carboxylate. Aziridine fragmentation through path (a) then generates the isopropylidene substituent (Scheme 55). The analogous reaction of N-benzylaziridinecarboxylate with potassium thiocyanate produced a mixture of regioisomeric thiazolidines, the ratio of which depended upon polarity of the solvent. In a neutral solvent such as THF, the major was a product through path (b), but as the acidity of the reaction medium was increased the proportion of path (a) product also increased. This suggests that opening of the protonated aziridine increasingly favors path (a), as would be expected on the basis of relative carbocation stabilization at C2 vs C3.
CONCLUDING REMARKS
Aziridines have proven to be versatile building blocks for the synthesis of bioactive compounds and the increasing interests in amine-containing molecules and their broad utility in organic and medicinal chemistry have made aziridines, in particular aziridinecarboxylates, more important and attractive substrates in contemporary synthetic chemistry. Therefore, the development of new methodologies for the construction and ring manipulation of aziridine skeleton, focusing on issues of cost efficiency and environment friendliness for potential manufacture, will be further requested in the future.
References
1. (a) D. Tanner and P. Somfai, Tetrahedron Lett., 1987, 28, 1211; CrossRef (b) K. Fugami, K. Miura, Y. Morizawa, K. Oshima, K. Utimoto, and H. Nozaki, Tetrahedron, 1989, 45, 3089; CrossRef (c) I. Funaki, P. L. Bell, L. Thijs, and B. Zwanenburg, Tetrahedron, 1996, 52, 12253. CrossRef
2. D. Tanner, Angew. Chem., Int. Ed. Engl., 1994, 33, 599. CrossRef
3. J. E. Baldwin, R. M. Adlington, and N. G. Robinson, J. Chem. Soc., Chem. Commun., 1987, 153. CrossRef
4. (a) K. Sato and A. P. Kozikowski, Tetrahedron Lett., 1989, 30, 4073; CrossRef (b) I. Shima, N. Shimazaki, K. Imai, K. Hemmi, and M. Hashimoto, Chem. Pharm. Bull., 1990, 38, 564. CrossRef
5. (a) J. E. Baldwin, R. M. Adlington, I. A. O’Neil, C. J. Schofield, A. C. Spivey, and J. B. Sweeney, J. Chem. Soc., Chem. Commun., 1989, 1852; (b) J. E. Baldwin, A. C. Spivey, C. J. Schofield, and J. B. Sweeney, Tetrahedron, 1993, 49, 6309. CrossRef
6. N. J. Church and D. W. Young, Tetrahedron Lett., 1995, 36, 151. CrossRef
7. J. E. Baldwin, C. N. Farthing, A. T. Russell, C. J. Schofield, and A. C. Spivey, Tetrahedron Lett., 1996, 37, 3761. CrossRef
8. (a) J. S. Brimacombe and K. M. M. Rahman, Carbohydr. Res., 1984, 30, 103; (b) A. Duréault, C. Greck, and J. C. Depezay, Tetrahedron Lett., 1986, 27, 4157; CrossRef (c) L. Dubois and R. H. Dodd, Tetrahedron, 1993, 49, 901. CrossRef
9. (a) J. Legters, L. Thijs, and B. Zwanenburg, Tetrahedron Lett., 1989, 30, 4881; CrossRef (b) N. A. J. M. Sommerdijk, P. J. J. A. Buynsters, H. Akdemir, D. G. Guerts, R. J. M. Nolte, and B. Zwanenburg, J. Org. Chem., 1997, 62, 4955. CrossRef
10. M. Martres, G. Gil, and A. Méou, Tetrahedron Lett., 1994, 35, 8787. CrossRef
11. (a) H. M. I. Osborn and J. Sweeney, Tetrahedron: Asymmetry, 1997, 8, 1693; CrossRef (b) R. S. Atkinson, Tetrahedron, 1999, 55, 1519; CrossRef (c) W. McCoull and F. A. Davis, Synthesis, 2000, 1347; CrossRef (d) G. Cardillo, L. Gentilucci, and A. Tolomelli, Aldrichimica Acta, 2003, 36, 39; (e) W. K. Lee and H.-J. Ha, Aldrichimica Acta, 2003, 36, 57; (f) X. E. Hu, Tetrahedron, 2004, 60, 2701. CrossRef
12. (a) A. Singh, H.-J. Ha, J. Park, J. H. Kim, and W. K. Lee, Bioorg. Med. Chem., 2011, 19, 6174; CrossRef (b) A. Singh, B. Kim, W. K. Lee, and H.-J. Ha, Org. Biomol. Chem., 2011, 9, 1372; CrossRef (c) K. M. Lee, J. C. Kim, P. Kang, W. K. Lee, H. Eum, and H.-J. Ha, Tetrahedron, 2012, 68, 883. CrossRef
13. T. Manaka, S.-I. Nagayama, W. Disadee, N. Yajima, T. Kumamoto, T. Watanabe, T. Ishikawa, M. Kawahata, and K. Yamaguchi, Helv. Chem. Acta, 2007, 90, 128. CrossRef
14. (a) D. L. Nagel, P. B. Woller, and N. H. Cromwell, J. Org. Chem., 1971, 36, 3911; CrossRef (b) P. Tarburton, P. B. Woller, R. C. Badger, E. Doomers, and N. H. Cromwell, J. Heterocycl. Chem., 1977, 14, 459; CrossRef (c) P. Garner, O. Dogan, and S. Pillai, Tetrahedron Lett., 1994, 35, 1653; CrossRef (d) G. Cardillo, L. Gentilucci, C. Tomasini, and M. P. Visa Castajon-Bordas, Tetrahedron: Asymmetry, 1996, 7, 755; CrossRef (e) S. N. Filigheddu and M. Taddei, Tetrahedron Lett., 1998, 39, 3857. CrossRef
15. A. B. McLaren and J. B. Sweeney, Org. Lett., 1999, 1, 1339. CrossRef
16. K.-S. Yang and K. Chen, J. Org. Chem., 2001, 66, 1676. CrossRef
17. E. Kuyl-Yeheskiely, M. Lodder, G. A. van der Marel, and J. H. van Boom, Tetrahedron Lett., 1992, 33, 3013. CrossRef
18. L. Marzorati, G. C. Barazzone, M. A. B. Filho, B. Wladislaw, and C. D. Vitta, Tetrahedron Lett., 2007, 48, 6509. CrossRef
19. (a) K. Hada, T. Watanabe, T. Isobe, and T. Ishikawa, J. Am. Chem. Soc., 2001, 123, 7705 (C&E News, August 13); (b) T. Ishikawa, Chem. Pharm. Bull., 2010, 58, 1555. CrossRef
20. (a) W. Disadee and T. Ishikawa, J. Org. Chem., 2005, 70, 9399; CrossRef (b) W. Disadee, T. Ishikawa, M. Kawahata, and K. Yamaguchi, J. Org. Chem., 2006, 71, 6600; CrossRef (c) T. Kumamoto, K. Suzuki, S.-K. Kim, K. Hoshino, M. Takahashi, H. Sato, H. Iwata, K. Ueno, M. Fukuzumi, and T. Ishikawa, Helv. Chem. Acta, 2010, 93, 2109. CrossRef
21. T. Haga and T. Ishikawa, Tetrahedron, 2005, 61, 2857. CrossRef
22. (a) C. Blandy, R. Choukroun, and D. Gervais, Tetrahedron Lett., 1983, 24, 4189; CrossRef (b) K. Maruoka, H. Sano, and H. Yamamoto, Chem. Lett., 1985, 599; CrossRef (c) D. Sinou and M. Emziane, Tetrahedron Lett., 1986, 27, 4423. CrossRef
23. (a) R. Zamboni and J. Rokach, Tetrahedron Lett., 1983, 24, 331; CrossRef (b) D. Tanner and P. Somfai, Tetrahedron, 1988, 44, 619. CrossRef
24. (a) D. Tanner, C. Birgesson, and H. K. Dhaliwal, Tetrahedron Lett., 1990, 31, 1903; CrossRef (b) J. Legters, L. Thijs, and B. Zwanenburg, Tetrahedron, 1991, 47, 5287. CrossRef
25. L.-D. Nie and X.-X. Shi, Tetrahedron: Asymmetry, 2009, 20, 124. CrossRef
26. (a) S. Gabriel, Chem. Ber., 1988, 21, 1049; (b) S. Gabriel, Chem. Ber., 1988, 21, 2664.
27. (a) G. V. Shustov, O. N. Krutius, V. N. Voznesensky, L. I. Chervin, A. V. Eremeev, R. G. Krostyanovsky, and F. D. Polyak, Tetrahedron, 1990, 46, 6741; CrossRef (b) S. D. Sharma, S. Kanwar, and S. Rajpool, J. Heterocycl. Chem., 2006, 43, 11. CrossRef
28. D. Chen, S. H. Kim, B. Hodges, and G. Li, ARKIVOC, 2003, xii, 56.
29. O. Ploux, M. Caruso, G. Chassaing, and A. Marquet, J. Org. Chem., 1988, 53, 3154. CrossRef
30. G. Cardillo, S. Casolari, L. Gentilucci, and C. Tomasini, Angew. Chem., Int. Ed. Engl., 1996, 35, 1848. CrossRef
31. (a) D. D. Dhavale, K. S. A. Kumar, V. D. Chaudhari, T. Sharma, S. G. Sabharwal, and J. P. Reddy, Org. Biomol. Chem., 2005, 3, 3720; CrossRef (b) K. S. A. Kumar, V. D. Chaudhari, and D. D. Dhavale, Org. Biomol. Chem., 2008, 6, 703. CrossRef
32. (a) H.-D. Ambrosi, W. Duczek, M. Ramm, E. Grundemann, B. Schulz, and K. Jahnisch, Liebigs Ann. Chem., 1994, 1013; CrossRef (b) A. E. Rubin and K. B. Sharpless, Angew. Chem., Int. Ed. Engl., 1997, 36, 2637; CrossRef (c) E. A. Davis, H. Liu, P. Zhou, T. Fang, G. V. Reddy, and Y. Zhang, J. Org. Chem., 1999, 64, 7559. CrossRef
33. S. Mangelinckx, A. Žukauskaitė, V. Buinauskaitė, A. Šačkus, and N. D. Kimpe, Tetrahedron Lett., 2008, 49, 6896. CrossRef
34. F. Colpaert, S. Mangelinckx, S. D. Brabandere, and N. D. Kimpe, J. Org. Chem., 2011, 76, 2204. CrossRef
35. A. A. Cantrill, L. D. Hall, A. N. Jarvis, H. M. I. Osborn, J. Raphy, and J. B. Sweeney, J. Chem. Soc., Chem. Commun., 1996, 2631.
36. F. A. Davis, P. Zhou, and G. V. Reddy, J. Org. Chem., 1994, 59, 3243. CrossRef
37. F. A. Davis, H. Liu, and G. V. Reddy, Tetrahedron Lett., 1996, 37, 5473. CrossRef
38. M. Ho, J. K. K. Chung, and N. Tang, Tetrahedron Lett., 1993, 34, 6513. CrossRef
39. (a) M. Bucciarelli, A. Forni, I. Moretti, and G. Torre, J. Org. Chem., 1983, 48, 2640; CrossRef (b) T. Fujiwara, R. Hayakawa, and M. Shimizu, Tetrahedron Lett., 1992, 33, 7903. CrossRef
40. (a) P. Baret, H. Buffet, and J. L. Pierre, Bull. Soc. Chim. Fr., 1972, 2493; (b) A. J. Hubert, A. Feron, R. Warin, and P. Teyssi, Tetrahedron Lett., 1976, 17, 1317; CrossRef (c) R. Bartnik and G. Mloston, Synthesis, 1983, 924. CrossRef
41. (a) K. B. Hansen, N. S. Finney, and E. N. Jacobsen, Angew. Chem., Int. Ed. Engl., 1995, 34, 676; CrossRef (b) K. G. Rasmussen and K. A. Jorgensen, J. Chem. Soc., Chem. Commun., 1995, 1401. CrossRef
42. J. M. Mohan, B. S. Uphade, V. R. Choudhary, T. Ravindranathan, and A. Sudalai, Chem. Commun., 1997, 1429. CrossRef
43. A. Mezumdar, Z. Xue, and M. F. Mayer, Synlett, 2007, 2025. CrossRef
44. S. Nagayama and S. Kobayashi, Chem. Lett., 1998, 685. CrossRef
45. J. S. Yadav, B. V. S. Reddy, M. Shesha Rao, and P. N. Reddy, Tetrahedron Lett., 2003, 44, 5275. CrossRef
46. (a) A. P. Patwardhan, V. R. Pulgam, Y. Zhang, and W. D. Wulff, Angew. Chem. Int. Ed., 2005, 44, 6169; CrossRef (b) A. A. Desai, R. Moran-Ramallal, and W. D. Wullf, Org. Synth., 2011, 88, 224 and references therein.
47. K. Juhl, R. G. Hazell, and K. A. Jorgensen, J. Chem. Soc., Perkin Trans. 1, 1999, 2293. CrossRef
48. (a) J. C. Antilla and W. D. Wulff, J. Am. Chem. Soc., 1999, 121, 5099; CrossRef (b) J. C. Antilla and W. D. Wulff, Angew. Chem. Int. Ed., 2000, 39, 4518. CrossRef
49. A. L. Williams and J. N. Johnston, J. Am. Chem. Soc., 2004, 126, 1612. CrossRef
50. T. Akiyama, T. Suzuki, and K. Mori, Org. Lett., 2009, 11, 2445. CrossRef
51. S. Fioravanti, A. Morreale, L. Pellacani, and P. A. Tardella, Synlett, 2004, 1083. CrossRef
52. (a) P. Scheiner, J. Am. Chem. Soc., 1966, 88, 4759; CrossRef (b) J. S. McConaghy, Jr. and W. Lwowski, J. Am. Chem. Soc., 1967, 89, 2357; CrossRef (c) A. Mishra, S. N. Rice, and W. Lwowski, J. Org. Chem., 1968, 33, 481. CrossRef
53. J. T. Kapron, B. D. Santarseiro, and J. C. Vederas, J. Chem. Soc., Chem. Commun., 1993, 1074. CrossRef
54. Z. Chilmonczyk, M. Egli, C. Behringer, and A. S. Dreiding, Helv. Chem. Acta, 1989, 72, 1095. CrossRef
55. (a) D. Mansay, J. Mashy, A. Duréault, G. Bedi, and P. Battioni, J. Chem. Soc., Chem. Commun., 1984, 1161; CrossRef (b) D. A. Evans, M. M. Faul, and M. T. Bilodeau, J. Org. Chem., 1991, 56, 6744. CrossRef
56. (a) Z. Li, K. R. Conser, and E. N. Jacobsen, J. Am. Chem. Soc., 1993, 115, 5326; CrossRef (b) D. A. Evans, M. M. Faul, M. T. Bilodeau, B. A. Anderson, and D. M. Barnes, J. Am. Chem. Soc., 1993, 115, 5328; CrossRef (c) D. A. Evans, M. M. Faul, and M. T. Bilodeau, J. Am. Chem. Soc., 1994, 116, 2742. CrossRef
57. H. Nishikawa and T. Katsuki, Tetrahedron Lett., 1996, 37, 9245. CrossRef
58. D. Chen, C. Timmons, L. Guo, X. Xu, and G. Li, Synlett, 2004, 2479. CrossRef
59. H. Kawabata, K. Omura, T. Uchida, and T. Katsuki, Chem. Asian J., 2007, 2, 248. CrossRef
60. J.-Y. Goujon, D. Gueyrard, P. Compain, O. R. Martin, K. Ikeda, A. Kato, and N. Asano, Biorg. Med. Chem. 2005, 13, 2313. CrossRef
61. A. Hassner and A. Kascheres, Tetrahedron Lett., 1970, 4623. CrossRef
62. M. J. Eis and B. Ganem, Tetrahedron Lett., 1985, 26, 1153. CrossRef
63. J. E. Baldwin, A. C. Spivery, C. J. Schofield, and J. B. Sweeney, Tetrahedron, 1993, 49, 6309. CrossRef
64. (a) N. J. Church and D. W. Young, Tetrahedron Lett., 1995, 36, 151; CrossRef (b) B. G. M. Burgaud, D. C. Horwell, A. Padova, and M. C. Pritchard, Tetrahedron, 1996, 52, 13035. CrossRef
65. T. Nishikawa, M. Ishikawa, K. Wada, and M. Isobe, Synlett, 2001, 945. CrossRef
66. T. Nishikawa, S. Kajii, K. Wada, M. Ishikawa, and M. Isobe, Synthesis, 2002, 1658. CrossRef
67. Y. Hayashi, T. Kumamoto, M. Kawahata, K. Yamaguchi, and T. Ishikawa, Tetrahedron, 2010, 66, 3836. CrossRef
68. (a) F. A. Davis, C.-H. Liang, and H. Liu, J. Org. Chem., 1997, 62, 3796; CrossRef (b) S. Chandrasekhar and M. Ahmed, Tetrahedron Lett., 1999, 40, 9325. CrossRef
69. I. Khantikaew, M. Takahashi, T. Kumamoto, N. Suzuki, and T. Ishikawa, Tetrahedron, 2012, 68, 878. CrossRef
70. Y. Lim and W. K. Lee, Tetrahedron Lett., 1995, 36, 8431. CrossRef
71. G.-I. Hwang, J.-H. Chung, and W. K. Lee, J. Org. Chem., 1996, 61, 6183. CrossRef
72. (a) F. A. Davis, Y. Zhang, A. Rao, and Z. Zhang, Tetrahedron, 2001, 57, 6345; CrossRef (b) F. A. Davis, J. Deng, Y. Zhang, and R. C. Haltiwanger, Tetrahedron, 2002, 58, 7135. CrossRef
73. E. N. Prabhakaran, J. P. Nandy, S. Shukla, A. Tewari, S. K. Das, and J. Iqbal, Tetrahedron Lett., 2002, 43, 6461. CrossRef
74. P. Dauban and R. H. Dodd, Tetrahedron Lett., 1998, 39, 5739. CrossRef
75. (a) D. Tanner and O. P. Gautun, Tetrahedron, 1995, 51, 8279; CrossRef (b) F. A. Davis, G. V. Reddy, and C. H. Liang, Tetrahedron Lett., 1997, 38, 5139. CrossRef
76. (a) G. A. Molander and P. J. Stengel, J. Org. Chem., 1995, 60, 6660; CrossRef (b) G. A. Molander and P. J. Stengel, Tetrahedron, 1997, 53, 8887. CrossRef
77. C. S. Pak, T. H. Kim, and S. J. Ha, J. Org. Chem., 1998, 63, 10006. CrossRef
78. G. Right, R. D’Achille, and C. Bonini, Tetrahedron Lett., 1996, 37, 6893. CrossRef
79. B. Crousse, S. Narizuka, D. Bonnet-Delpon, and J.-P. Begue, Synlett, 2001, 679. CrossRef
80. D. Gneeco, O. F. Laure, A. Galindo, R. G. Enriquez, R. A. Toscano, and W. F. Reynolds, Molecules, 2000, 5, 998. CrossRef
81. G. Righi, C. Potini, and P. Bovicelli, Tetrahedron Lett., 2002, 43, 5867. CrossRef
82. S.-H. Shin, E. Y. Han, C. S. Park, W. K. Lee, and H.-J. Ha, Tetrahedron: Asymmetry, 2000, 11, 3293. CrossRef
83. Y. Kim, H.-J. Ha, K. Han, S. W. Ko, H. Yun, H. J. Yoon, M. S. Kim, and W. K. Lee, Tetrahedron Lett., 2005, 46, 4407. CrossRef
84. G. Rinaudo, S. Narizuka, N. Askari, B. Crousse, and D. Bonnet-Delpon, Tetrahedron Lett., 2006, 47, 2065. CrossRef
85. U. K. Nadir and A. Singh, Tetrahedron Lett., 2005, 46, 208. CrossRef
86. B. Saha, J. P. Nandy, S. Shukla, I. Siddiqui, and J. Iqbal, J. Org. Chem., 2002, 67, 7858. CrossRef
87. F. A. Davis and G. V. Reddy, Tetrahedron Lett., 1996, 37, 4349. CrossRef
88. M. Bucciarelli, A. Forni, I. Moretti, F. Prati, and G. Torre, Tetrahedron: Asymmetry, 1995, 6, 2073. CrossRef
89. (a) T. Wakamiya, K. Shimbo, T. Shiba, K. Nakajima, M. Neya, and K. Okawa, Bull. Chem. Soc. Jpn., 1982, 55, 3878; CrossRef (b) O. Ploux, M. Caruso, G. Chassaing, and A. Marquet, J. Org. Chem., 1988, 53, 3154; CrossRef (c) H. Shao, Q. Zhu, and M. Goodman, J. Org. Chem., 1995, 60, 790; CrossRef (d) B. Zwanenburg and L. Thijs, Pure Appl. Chem., 1996, 68, 735; CrossRef (e) H. K. Lee, J. H. Im, and S. H. Jung, Tetrahedron, 2007, 63, 3321. CrossRef
90. C. Xiong, W. Wang, C. Cai, and V. J. Hruby, J. Org. Chem., 2002, 67, 1399. CrossRef
91. (a) K. Hori, T. Nishiguchi, and A. Nabeya, J. Org. Chem., 1997, 62, 3081; CrossRef (b) D. Ferraris, W. J. Drury III, C. Cox, and T. Lectka, J. Org. Chem., 1998, 63, 4568. CrossRef
92. (a) G. Cardillo, L. Gentilucci, A. Tolomelli, and C. Tomasini, Tetrahedron Lett., 1997, 38, 6953; CrossRef (b) G. Cardillo, L. Gentilucci, and A. Tolomelli, Tetrahedron Lett., 1999, 40, 8261; CrossRef (c) G. Cardillo, L. Gentilucci, M. Gianotti, and A. Tolomelli, Tetrahedron: Asymmetry, 2001, 12, 563. CrossRef
93. G. Cardillo, L. Gentilucci, M. Gianotti, and A. Tolomelli, Tetrahedron, 2001, 57, 2807. CrossRef
94. S. Lucarini and C. Tomasini, J. Org. Chem., 2001, 66, 727. CrossRef
95. S. L. Crawley and R. L. Funk, Org. Lett., 2006, 8, 3995. CrossRef
96. T. B. Sim, S. H. Kang, K. S. Lee, and W. K. Lee, J. Org. Chem., 2003, 68, 104. CrossRef
97. M. S. Kim, Y.-W. Kim, H. S. Hahn, J. W. Jang, W. K. Lee, and H.-J. Ha, Chem. Commun., 2005, 3062. CrossRef
98. J. D. White and T. Furuta, Heterocycles, 2009, 79, 347. CrossRef