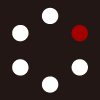
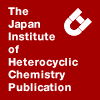
HETEROCYCLES
An International Journal for Reviews and Communications in Heterocyclic ChemistryWeb Edition ISSN: 1881-0942
Published online by The Japan Institute of Heterocyclic Chemistry
e-Journal
Full Text HTML
Received, 28th August, 2012, Accepted, 25th October, 2012, Published online, 31st October, 2012.
DOI: 10.3987/REV-12-750
■ Syntheses of Bilin Chromophores Toward the Investigation of Structure and Function of Phytochromes
Katsuhiko Inomata*
Division of Material Sciences, Graduate School of Natural Science and Technology, Kanazawa University, Kakuma, Kanazawa, Ishikawa 920-1192, Japan
Abstract
We studied on the syntheses of linear tetrapyrrole (bilin) chromophores including the sterically locked derivatives toward the investigation of structure and function of phytochromes as photoreceptors. The synthesized chromophores were successfully assembled with bacterial and plant apophytochromes in vitro and/or in vivo to demonstrate that they are valuable tools for studies on phytochromes.CONTENTS
1. Introduction
2. Development of General Synthetic Method of Bilin Chromophores
2-1. Preparation of the A- and D-Ring Components
2-2. Preparation of the B- and C-Ring Components
2-3. Preparation of Pyrromethenone Derivatives as the AB- and CD-Ring Components
2-4. Preparation of Linear Tetrapyrrole (Bilin) Chromophores
3. In Vitro and In Vivo Assembly of the Synthesized Bilin Chromophores with Apophytochromes
4. Development of General Synthetic Method of Sterically Locked Bilin Chromophores
5. In Vitro and In Vivo Assembly of the Synthesized Locked Bilin Chromophores with Apophytochromes
6. Development of General Synthetic Method of Doubly Locked Bilin Chromophores
7. Conclusion
1. INTRODUCTION
Phytochromes are bilin-containing photoreceptors1–5 in plants, 6,7 bacteria,8–10 and fungi,11 which regulate numerous photoresponses such as phototaxis and pigmentation in bacteria, seed germination, chloroplast development, modulation of gravitropism, inhibition of hypocotyl elongation, induction of cotyledon opening and side branches, flowering, and gene regulation in plants through the photointerconversion between a red-light-absorbing form (Pr) and a far-red-light-absorbing form (Pfr).1,7 All phytochromes have a covalently attached bilin chromophore that absorbs light in red and far-red region.5,12–18 Land plants use phytochromobilin (PΦB),1 cyanobacteria use phycocyanobilin (PCB),19,20 and other bacteria use biliverdin (BV) (Figure 1).11,21,22
The natural chromophores PΦB and PCB are coupled by a thioether bond between the C31 position of the A-ring ethylidene side chain and a conserved cysteine residue within the GAF domain of the proteins. Many bacterial phytochromes carry BV as a natural chromophore, which is coupled at the C32 position in a different manner to the protein.
For many years, it has been assumed that the primary event of the photoconversion from Pr to Pfr is a Z to E isomerization of the C15=C16 methine bridge between the C- and D-rings of the bilin chromophores.23 However, this view was recently challenged by the hypothesis formulated in the context of NMR data on a phytochrome-like protein from a thermophilic cyanobacterium SyB-Cph1, i.e., light-induced rotation of the A-ring but not the D-ring is the primary motion of the chromophore during photoconversion.24
Furthermore, even in the traditional view based on the Z to E photoconversion around the C15=C16 double bond, the chromophore can also move around the exocyclic single bonds. Each single bond can adopt either a syn or anti conformation.12 Vibrational spectroscopy have provided indirect insight into the conformation of the phytochrome chromophore in the Pr, Pfr, and intermediate states, but the data have been interpreted in different ways.25–28 For example, it has been proposed that the formation of Pfr is accompanied by a syn/anti rotation around the C14–C15 single bond.27 More recently, interpretation of resonance Raman spectra of plant phytochromes by density functional theory (DFT) calculations indicated that the C14–C15 single bond is in an anti conformation throughout the entire photocycle and that the C5–C6 single bond rotates from anti to syn upon photoconversion from Pr to Pfr (Figure 2).28
By photoconverting between Pr and Pfr, phytochromes act as unique light-regulated switches in various signal transduction cascades. Despite intensive physico-chemical analysis of various phytochromes, we do not yet understand how contacts between polypeptide and the bilin enable photoconversion between Pr and Pfr, how this transformation reversibly alters the activity of the photoreceptor, or how the holoprotein dimerizes.18,29
The development of yeast and bacterial system for the expression of recombinant phytochrome apoproteins has allowed investigation of the biochemical and spectroscopic properties of the reconstituted phytochromes.30 Moreover, the photophysical and photochemical properties of wild type phytochrome are quite similar to those of the reconstituted chromoproteins.31,32 Other strategies, including systematic N- and C-terminal truncations and site-directed mutagenesis of the apoprotein, have been used to study the structural requirements of the chromophore-apoprotein interaction in terms of photochromism.33–35
Even though such bilin chromophores could be isolated from natural sources, knowledge of the relationship between the structure of synthetic bilin chromophores and the biochemical properties of the holoproteins assembled with apophytochromes is quite interesting and important to determine the precise function of the bilin chromophores. However, little had been done to examine the relationships among chromophore structure, its assembly to apoprotein, and photochromism of the holoprotein, because of the difficulty of synthesizing the natural bilin chromophores and their structural analogs.
Although Gossauer and his co-workers reported total syntheses of dimethyl ester derivatives of PΦB and PCB around 1980, they could not assemble these analogs with phytochrome apoprotein.36–38 Since then, there was no report regarding the synthesis of the free acid form of PΦB or PCB applicable to assemble with the phytochrome apoproteins for a decade.
Therefore, we studied on the syntheses of natural and unnatural bilin chromophores,39–49 and have succeeded in synthesizing PΦB,44 PCB,42,43,48 BV and their analogs including sterically locked derivatives45,46,49 in free acid forms by developing efficient synthetic methods of each pyrrole ring, a new coupling reaction, and palladium-catalyzed deprotection of the allyl propanoate side chains of the B- and C-rings under mild conditions. Finally, assembly experiments of the synthesized chromophores with phytochrome apoproteins in vitro and/or in vivo have provided us new insights into the structure and function of phytochromes.
The background of our studies, including assembly experiments with apophytochromes, have been described in the previous review.50 Thus, this paper focuses on our recent studies on the syntheses of various bilin chromophores.
2. DEVELOPMENT OF GENERAL SYNTHETIC METHOD OF BILIN CHROMOPHORES
Toward establishment of an efficient method for the preparation of bilin chromophores of phytochromes, we initially tried to improve the method employed by Gossauer and his co-workers for the preparation of dimethyl esters of PΦB and PCB,36–38 according to a retrosynthetic analysis shown in Figure 3. To prepare free acid forms of bilin chromophores as final products, we replaced the methyl esters of propanoic acid side chains at the C8 and C12 positions and the benzyl ester at the C5 meso position, which were employed by Gossauer’s group, to allyl esters, respectively.50 Furthermore, we developed a new coupling reaction between the C- and D-rings, instead of the classical method using a strong base, to avoid the hydrolysis of the ester groups.50
This method was useful for the preparation of PCB and its analogs modified at the A-ring (1–4 in Figure 4), B- and/or C-ring(s) (5–12), and D-ring (13–20) as well as the PCB derivatives bearing a photoreactive 4-[3-(trifluoromethyl)diaziren-3-yl]phenyl group51,52 on the D-ring for a photoaffinity study.42,43,48,53 However, it was found to be difficult to apply this method to the synthesis of PΦB bearing an acid labile vinyl group at the C18 position (R5 = vinyl), since this method required the treatment with trifluoroacetic acid (TFA) for decarboxylation at the C5 meso position at the final step of the synthesis.
Therefore, we developed an alternative more flexible method (Scheme 1), which does not require the decarboxylation at the C5 position different from the above method. It was applicable not only for the preparation of PCB, PΦB and their derivatives, but also for the preparation of BV and its derivatives.
2-1. Preparation of the A- and D-Ring Components
3,4-Disubstituted 5-tosylpyrrolinones 22 as the A- or D-ring precursor of bilin chromophores were derived from the corresponding 2-bromo-5-tosylpyrroles 21 by the redox reaction shown in Scheme 2.
The starting 3,4-disubstituted 2-bromo-5-tosylpyrroles 21 were readily available by bromination of the corresponding 2-tosypyrroles 27, which were prepared from nitroalkane and aldehyde via nitro-acetate 25 and/or nitroolefin 26, followed by the reaction with tosylmethyl isocyanide54 by applying the Barton’s method55 as shown in Scheme 3.
In the course of the investigation of the synthesis of 2-tosylpyrroles 27, the isolated 27 was found to be contaminated with its regioisomer 27’ from NMR spectrum taken in CDCl3, even though it was carefully purified on TLC. Furthermore, such phenomenon was not observed when CDCl3 treated with basic alumina was used for NMR measurement. This fact strongly suggested that the tosyl group of 3,4-disubstituted 2-tosylpyrrole 27 rearranged from the C2 position to the C5 position under the mild acidic conditions. Actually, the tosyl group of 27 could be readily rearranged in CDCl3 containing trifluoroacetic acid (TFA) (TFA/CDCl3 = 1/9). The ratio of the regioisomers (27 and 27’) at equilibrium was definitely influenced by the bulkiness of the substituent R’ (marked with a grey circle in Scheme 3) at the C3 position of the starting 4-methyl-3-substituted 2-tosylpyrroles 27 (R = Me). When the substituent R’ was t-butyl group, 27 was completely transformed to 27’ (R = Me, R’ = tBu).56
From the fact that addition of a catalytic amount of p-toluenesulfinic acid (p-TolSO2H = TsH) remarkably accelerated the reaction,57 the rearrangement of a tosyl group from the C2 position to the C5 position seemed to proceed through the mechanism shown in Scheme 4. Namely, initial protonation takes place selectively at the C2 position bearing a tosyl group due to the strong inductive effect of the tosyl group to afford the cationic intermediate 28, just like in the case of reversible sulfonation of aromatic compounds under acidic conditions. Then addition of p-toluenesulfinate at the C5 position takes place to afford the intermediate 29, which has two tosyl groups at both α-positions. From the intermediate 29, p-toluenesulfinic acid must be selectively eliminated to release the unfavorable steric repulsion between a bulkier substituent R’ and a tosyl group found in the starting 2-tosylpyrrole 27a (R = Me < R’). The ratio of 27/27’ at equilibrium was proven to be dependent only on the steric requirement between a tosyl group and the substituent (R’ or R) at the C3 or C4 position of 27.
This rearrangement is synthetically very useful. Barton and his coworkers have reported a convenient method for the preparation of substituted pyrroles through the reaction of isonitrile with a nitroolefin or its equivalent available from nitroalkane and aldehyde.55 However, we were sometimes confronted with the difficulty of introducing arbitrary substituents at the C3 and C4 positions of pyrroles according to the Barton’s method. The synthesis of the sterically unfavorable 4-methyl-3-substituted 2-tosylpyrrole 27a (R = Me < R’, Eq. 1 in Scheme 5) is much easier than that of its regioisomer, 3-methyl-4-substituted 2-tosylpyrrole 27a’ (R = Me < R’, Eq. 2 in Scheme 5), because substituent R’ at the C3 position of pyrrole 27a is originated from commercially available aldehydes 30 in Eq. 1, whereas the substituent R’ at the C4 position of the pyrrole 27a’ in Eq. 2 is originated from nitroalkanes 31, which are not commercially available except simple ones.
Consequently, this rearrangement allowed us to exchange the substituents (R and R’) at the C3 and C4 positions of 5-tosylpyrrolinones 22 as precursors of the A- or D-ring of bilin chromophores through the intermediary pyrroles 27 and 27’.
2-2. Preparation of the B- and C-Ring Components
The formylpyrrole 33 (R’ = CH2CH2CO2Allyl) as the B- or C-ring precursor of bilin chromophores was readily available by applying the Barton’s method,55 followed by the Vilsmeier–Haack reaction, as shown in Scheme 6.
2-3. Preparation of Pyrromethenone Derivatives as the AB- and CD-Ring Components
Now, various kinds of the A-, B-, C-, and D-rings as the starting compounds shown in Scheme 1 have become available.
On the other hand, 5-tosylpyrrolinone 22 was found to undergo the Wittig-type coupling reaction with aldehydes (R”CHO) in the presence of DBU and tributylphosphine to give the corresponding 5-exomethylene derivatives 34, including a pyrromethenone derivative (R” = 2-pyrrolyl). This reaction seems to proceed through the mechanism shown in Scheme 7.39–41
Applying this Wittig-type coupling reaction, we could develop an alternative method for the synthesis of the AB-ring components, 41 of BV and 43 of PΦB/PCB, without an ester group at the C5 meso-position.
When 5-tosylpyrrolinone 38 as an A-ring precursor was coupled with formylpyrrole 33 in the presence of tributylphosphine and DBU in THF at room temperature, a mixture of (E)- and (Z)-pyrromethenone intermediate 39 was obtained in 80% yield. The E-isomer of 39 was readily converted to the thermodynamically more stable Z-isomer by treating with a catalytic amount of iodine in CH2Cl2 at room temperature in quantitative yield. A 2-(tolylthio)ethyl group of the coupling product 39 was oxidized to the corresponding sulfoxide 40 with mCPBA in CH2Cl2 and converted to a vinyl group by heating in xylene to afford 41, which can be regarded as the AB-ring component of BV. The pyrromethenone derivative 41 having a vinyl group on the A-ring could be further converted to the AB-ring component 43 of PΦB and PCB, i.e., compound 41 was treated with Al(Hg) to the reduced intermediate 42,58 followed by acid treatment without purification to obtain 43 via rearrangement of a proton at the C5 meso-position as a single Z-isomer (confirmed by NOE measurement) in good yield as shown in Scheme 8.43,44,59
Decarboxylated AB-ring component 44, which has an acid labile vinyl group, was prepared from (E/Z)-39 via oxidation to the sulfoxide (E/Z)-40 and subsequent treatment with a mixture of formic acid and TFA for decarboxylation, followed by refluxing in DMF containing pyridine to introduce a vinyl group under basic conditions resulting in the formation of 44 as only Z-isomer in 57% yield in three steps from 39 (Scheme 8).60 Compound 44 was used for the synthesis of the locked BV derivatives as will be described later.
In a similar manner, the CD-ring components of PΦB/BV (51) and PCB (50) were prepared from 5-tosylpyrrolinones 38’, which is a regioisomer of the A-ring precursor 38, and 45 as the D-ring precursor of PCB, by coupling with formylpyrrole 33 through our original Wittig-type reaction. The resulting E/Z-mixtures of 46 and 48 were treated with a catalytic amount of iodine to give only Z-isomers in good yields, respectively. A thioether bond of the coupling product 46 was oxidized with mCPBA to afford sulfoxide 47. Pyrromethenone derivatives 47 and 48 were treated with TFA for decarboxylation, followed by formylation with methyl orthoformate to afford the corresponding formylated products 49 and 50, the latter of which is the CD-ring component of PCB. The CD-ring component 51 of PΦB and BV was obtained by heating 49 in xylene in the presence of pyridine to introduce a vinyl group on the D-ring as shown in Scheme 9.44
2-4. Preparation of Linear Tetrapyrrole (Bilin) Chromophores
As described above, the AB- and CD-ring components were in hand. They were next coupled in alcohol in the presence of an acid catalyst to construct the corresponding linear tetrapyrrole (bilin) derivatives. Scheme 10 shows the coupling in the case of PΦB between the CD-ring component 51 and the AB-ring component 52, which was obtained from 43 by decarboxylation in TFA. The two allyl ester groups of the coupling product 53 were deprotected all at once by treating with excess amounts of morpholine in the presence of Pd-catalyst,61 avoiding migration of the exocyclic olefin of the A-ring to the endocyclic position, to afford PΦB in free acid form.44
Finally, PΦB was successfully prepared starting from only two pyrrole derivatives 27b (R = Me, R’ = CH2CH2STol) and 33 as depicted in Scheme 11, by developing novel synthetic reactions described above: (1) rearrangement of the tosyl group of 2-tosylpyrroles to the 5-position under mild acidic conditions (Schemes 3 and 4), (2) efficient transformation of 2-bromo-5-tosylpyrroles to the corresponding 5-tosylpyrrolinones by a redox reaction (Scheme 2; NaI was initially used instead of Zn as a reducing agent47), (3) the Wittig-type coupling reaction between 5-tosylpyrrolinone and 2-formylpyrrole for the preparation of the AB- and CD-ring components (Schemes 7–9), (4) reductive transformation of the AB-ring component of BV to the AB-ring component of PΦB and BV (Scheme 8), and (5) protection and palladium-catalyzed deprotection of propanoic acid side chains at the C8 and C12 positions via allyl esters (Scheme 10).
Though it is possible to introduce a formyl group to the AB-ring component instead of the CD-ring, it looked better to introduce the electron withdrawing formyl group to the CD-ring component to stabilize an acid labile vinyl group at the D-ring. Furthermore, it was found that PΦB is extremely unstable in free acid form compared with PCB, especially without solidifying after purification.
In a similar manner, we could prepare PCB and various kinds of its derivatives. 3,31-Dihydrogenated PCB derivatives were also synthesized to investigate their non-covalent interaction with apophytochromes toward the development of affinity chromatography to purify the apoproteins.42,46 It was confirmed by NOESY that all of the synthesized bilin chromophores have an all-Z configuration and an all-syn conformation, respectively.
The above synthetic method is applicable not only for the preparation of PCB, PΦB, and their derivatives, but also for the preparation of BV and its derivatives (see Scheme 1). Actually we could prepare BV and its derivatives, 3EtBV, 18EtBV, 3,18EtBV, and BV-4 shown in Figure 5, for assembly experiment with Agrobacterium phytochrome Agp1 as will be described later.
3. IN VITRO AND IN VIVO ASSEMBLY OF THE SYNTHESIZED BILIN CHROMOPHORES WITH APOPHYTOCHROMES
Now PCB, PΦB, BV, and various kinds of their derivatives have become available in free acid forms to study on the reconstituted phytochromes. By photointerconversion between Pr and Pfr, phytochromes act as light-regulated switches for measuring the fluence, direction, duration and color of the ambient light environment.62
Phytochromes A and B (PhyA and PhyB) in the model plant species Arabidopsis thaliana recognize light in essentially different manners.63 PhyB photoreversibly switches responses on or off upon irradiation with red/far-red light, whereas PhyA triggers very low-fluence or high-irradiance responses.64
To analyze the structural requirements of the chromophore for the spectral properties of PhyB, we designed and chemically synthesized more than 20 bilin chromophore derivatives (1–20 in Figure 4) including natural PΦB (Scheme 11) and PCB. These chromophores were assembled with recombinant Arabidopsis PhyB apoprotein (PHYB) through collaborative study with biologists.65
The difference absorption spectra of the in vitro-assembled adducts of the synthetic bilin chromophores (PΦB, PCB, and 1–20) with PHYB were measured on far-red and red light irradiations. The adduct with PCB showed a similar difference spectrum to that of the PΦB adduct, although its peaks were slightly blue shifted due to the difference of the length of π-conjugation system when compared with the PΦB adduct corresponding to the natural phytochrome,66–68 as shown in Figure 6.65
From the results of the difference absorption spectra of PHYB adducts and the biding efficiency (by zinc blot analysis) of the synthesized PCB analogs (1–20) modified at the A-, B-, C-, and D-rings, respectively, we could find valuable insights into phytochromes. 65 Namely, (1) the A-ring acts mainly as the anchor for ligation to PHYB, because the modification of the side chains at the C2 and C3 positions (analogs 1–4) did not significantly influence the biding efficiency and/or difference spectra of adducts. These results indicated that a hydrophobic environment and open space accommodate the A-ring of the bilin chromophore at the binding site of PHYB, and also that stereochemistry at the C2 position was not crucial, since the analog 3, dimethylated at the C2 position, showed similar difference spectra.
In contrast, (2) the side chains of the B- and C-rings were found to be crucial to position the chromophore properly in the chromophore pocket of PHYB and for photoreversible spectral changes by using analogs 5–12. Exchanging the methyl groups and the propanoic acid side chains of the B- and C-rings decreased the binding efficiency remarkably,65 suggesting that free-acid elements are required for chromophore binding, probably dictated by the electrostatic interaction between propanoic acid side chains and basic amino acid residues of the phytochrome apoprotein. When PHYB adducts retained a propanoic acid side chain at the C8 position of the B-ring, they displayed photochromism. Thus, propanoic acid side chain at the C8 position was found to be more crucial for photoreversible conversion of phytochrome than that at the C12 position.
(3) The side-chain structure of the D-ring was required for the photoreversible spectral change of the adducts.65 When methyl and ethyl groups at the C17 and C18 positions of PCB were replaced with a propyl or octyl group (analogs 13–18), respectively, the photoreversible spectral change of the adducts depended on the length of the side chains. Comparison of the difference absorption spectra of the adducts replaced with an octyl group (analogs 15 and 18) suggested that the environment around the C18 position of the chromophore within the chromophore-biding pocket of PHYB is hydrophobic and more flexible than that of the C17 position. This suggestion was supported by the results from other two analogs 19 and 20, which have the 2-(acetoxy)ethyl or 2-(tolylthio)ethyl groups at the C18 position.65
From these studies, we proposed that each pyrrole ring of the linear tetrapyrrole (bilin) chromophore plays a different role in chromophores assembly and the photochromic properties of PhyB.
Furthermore, these results allowed us to outline the order of events during in vitro assembly of PHYB with its chromophore as shown in Figure 7.
The ligation of chromophore to PHYB is initiated by an electrostatic interaction between the propanoic acid side chains of the B- and C-rings and basic amino acid residues such as Arg or Lys, probably accompanied by an additional interaction between an acidic amino acid residue and the basic nitrogen of the pyrrole (the ring-B in Figure 7). Rotation around the C14–C15 single bond of the original all-Z and all-syn bilin chromophore would occur at this initial stage to form an extended anti conformation of the chromophore in PHYB, as will be discussed later. The A-ring falls into the hydrophobic site close to the Cys within the GAF domain of PHYB, followed by nucleophilic attack of a thiol group of the Cys onto the C31 position of the ethylidene substituent of the A-ring to form a thioether bond through a sort of the Michael-type addition reaction. The D-ring also falls into a hydrophobic pocket where open space exists particularly around the C18 position of the chromophore to afford Pr form, which acts as the photoreceptor for red/far-red reversible reaction (Figure 7).
It was possible to replace a side chain at the C17 or C18 position of the D-ring with a bulky substituent without loss of photoconversion. Thus, these positions were labeled with a photoreactive group for photoaffinity studies. PCB analogs carrying a photoreactive 4-[3-(trifluoromethyl)diaziren-3-yl]phenyl group at the C17 or C18 position of the D-ring, however, were not incorporated into PHYB, probably due to the bulkiness and the lack of flexibility of the employed photoreactive group.
Phytochromes are encoded by a small gene family, and five distinct phytochromes (A to E) have been identified in the model plant species Arabidopsis thaliana,69,70 in which phytochromes A (PhyA) and B (PhyB) are most abundant, principally working throughout the life cycle. Photobiological analyses have established that, under continuous irradiation, PhyA is primarily responsible for plant’s sensitivity to far-red light, whereas the other phytochromes respond mainly to red light, using various phytochrome-deficient mutants.4 To elucidate a question whether different bilin structures affect the photobiological activities of phytochromes in vivo, we have collaborated with biologists to incorporate synthetic bilin chromophores into apopytochromes A (PHYA) and B (PHYB) in Arabidopsis hy1 and hy2 mutants, which are deficient in PΦB biosynthesis.71–73 Parks and Quail have restored photomorphogenesis in these mutants with exogenously supplied biliverdin IXα (BV), a direct precursor of PΦB.74 By using an analogous approach, synthetic bilins were fed exogenously to hy1 and hy2 seedlings to test whether they restore the photobiological functions of PhyA and PhyB in vivo.75
The far-red light sensitivity of PhyA was found to depend on the structure of the bilin chromophore, i.e., a double bond on the D-ring of PΦB was required for restoring PhyA function, whereas PhyB function was restored with both PΦB and PCB with a saturated D-ring substituent.75
This collaborative work with biologists demonstrated a quite suggestive discovery that a double bond in the vinyl side chain at the C18 position of D-ring of PΦB is crucial for the photosensory function of PhyA, and has opened an avenue of research toward understanding the nature of holophytochrome assembly and the chromophore pocket in vitro and in vivo.
Different from plant phytochromes, many bacterial phytochromes carry biliverdin (BV) as a natural chromophore, which is coupled in a different manner to the protein, because BV has a vinyl side chain on the A-ring instead of an ethylidene side chain of PΦB and PCB. In phytochrome Agp1 of Agrobacterium tumefaciens, BV is covalently attached to a cysteine residue close to the N-terminus (position 20). It had been proposed that BV binds via its D-ring to the protein,76 but the assumption had not been confirmed by chemical studies.
Therefore, we used different natural and synthetic BV analogs prepared above (Figure 5), three BV-derivatives with a reduced A-ring and/or D-ring side chains, namely 3EtBV, 18EtBV, 3,18EtBV, and BV-4, in which the D-ring vinyl group of the C18 position was exchanged with the methyl group of the C17 position, to test whether the A-ring or D-ring vinyl side chain is used for covalent BV attachment in Agp1.77 Chromophore assembly was carried out by German biologists as our collaborative work, and it was found that all tested chromophores formed adducts with the Agp1 apoprotein. In addition, all adducts showed typical photoconversion into Pfr form upon irradiation with red light.77
Covalent attachment of the chromophores was further tested by SDS-denaturation/dissociation followed by separation of protein and the free bilin on desalting columns. If the chromophore is covalently bound, it elutes together with the protein from the desalting column, whereas noncovalently bound chromophore is separated from the protein by treating with SDS and remains almost entirely inside the column. The assay showed that BV, 18EtBV and BV-4 adducts were covalently attached. All three covalently bound chromophores have an A-ring vinyl side chain (marked with a grey circle in Figure 5) but differ by their D-ring side chains. Those chromophores that contain an A-ring ethyl or ethylidene side chain, namely 3EtBV, 3,18EtBV, PΦB, PCB, were noncovalently bound to the protein.
From these results, we could conclude that a vinyl group of the A-ring of BV chromophore forms a thioether bond with the cysteine 20 residue of Agp1.77 This result was then confirmed by X-ray crystallography of a truncated phytochrome from Deinococcus radiodurans consisting of the N-terminal 321 amino acids.78
4. DEVELOPMENT OF GENERAL SYNTHETIC METHOD OF STERICALLY LOCKED BILIN CHROMOPHORES
As mentioned above, we could establish an efficient and flexible synthetic method of various kinds of bilin chromophores,41–46 including PΦB,44 PCB, 42,43 and various kinds of PCB derivatives,45,46 in free acid forms, which made it possible to assemble them with the apoproteins not only in vitro to analyze the spectral properties of the resulting holoproteins,65 but also in vivo to observe their physiological functions.75
On the other hand, it has been shown for plant phytochrome that the first step of the Pr to Pfr photoconversion is a Z to E isomerization of the chromophore around the C15=C16 double bond,23 which occurs on the picosecond time scale.79–81 Isomerization is followed by spectral changes in the microsecond and millisecond time scale.82–84 During photoconversion, the chromophore also undergoes movement around the exocyclic single bonds. In principle, each exocyclic single bond can adopt either a syn or anti conformation during photoconversion.12 Vibrational spectroscopy gained indirect insight into the conformation of the phytochrome chromophore in the Pr, Pfr, and intermediate states, but the data were ambiguous and have been interpreted in different ways.25–28 For example, it has been proposed that the formation of Pfr is accompanied by a syn/anti rotation around the C14–C15 single bond.27 More recently, interpretation of resonance Raman spectra by using density functional theory (DFT) calculations indicated that the C14–C15 single bond is in an anti conformation throughout the entire photocycle and that the C5–C6 single bond rotates from anti to syn upon conversion from Pr to Pfr as shown in Figure 2.28 Therefore, to directly confirm the stereochemistry of the chromophore in phytochrome around the C15 position for both Pr- and Pfr-forms, we first prepared four different types of BV derivatives (62–65), in which the C- and D-rings are sterically locked by cyclizing with an additional carbon chain, by applying the above general synthetic method of bilin chromophores.
The AB-ring component 44 common to all of the locked BV derivatives (62–65) were prepared as shown in Scheme 8 from (E/Z)-39.60 Thus, total syntheses of the four different types of BV derivatives (62–65), in which the stereochemistry between the C- and D-rings are locked in Z-syn, Z-anti, E-syn, and E-anti configuration and conformation, respectively, were accomplished via establishment of new and efficient methods for the construction of the sterically locked CD-ring components (58–61) based on the retrosynthetic analyses depicted in Figure 8.
The Z-syn CD-ring precursor 66 was prepared by treating (Z)-48, which is available by the Wittig-type coupling of 45 and 33 (Scheme 9), with 1,2-dibromoethane in the presence of NaH in 84% yield. The t-butoxycarbonyl group of 66 was converted to a formyl group by treating with TFA, followed by addition of methyl orthoformate at room temperature to give the formylated CD-ring component 58 in quantitative yield (Scheme 12). Coupling reaction between the CD-ring component 58 and the AB-ring component 44 was carried out in methanol under acidic conditions to afford 15Zs-18Et-BV diallyl ester 67 in 69% yield. The two allyl ester groups were deprotected by a Pd(0)-catalyzed reaction using sodium p-toluenesulfinate (NaTs) as a nucleophile in THF/MeOH to give locked chromophore 62 (15Zs-18Et-BV) in free acid form in 80% yield.49
The Z-anti CD-ring component 59 was prepared from the D-ring 4547 and the C-ring 54 bearing a leaving group for cyclization.60 As shown in Scheme 13, commercially available 3-bromo-1-propanol (68) was acetylated, followed by nitration using sodium nitrite in the presence of phloroglucinol in DMF to give 3-nitropropyl acetate (69) in 60% yield. Compound 69 was coupled with oxoester 70 in a similar manner for the preparation of the B- and C-rings of bilin chromophore.41,45 Subsequent acetylation of the resulting alcohol gave nitro-acetate 71 in 66% yield, along with a small amount of nitroolefin. When compound 71 was treated with t-butyl isocyanoacetate and DBU in acetonitrile according to the Barton’s method,55 pyrrole derivative 72 was obtained in 55% yield. Hydrolysis of 72 with KOH in MeOH, followed by allylation with allyl bromide in the presence of DBU in THF/DMF, gave pyrrole 73 in 60% yield. When compound 73 was formylated by the Vilsmeier reaction to give the formylated product, chlorination of the hydroxy group proceeded simultaneously to afford formylpyrrole 54 in 94% yield.
The Wittig-type coupling reaction between 5-tosylpyrrolinone 45 and formylpyrrole 54 proceeded satisfactorily in the presence of tributylphosphine and DBU in THF at 0 °C to room temperature to afford the CD-ring components 74 as a mixture of E- and Z-isomers in 98% yield (Scheme 14). It turned out that compound (E)-74 had to be converted to the Z-isomer by treating with a catalytic amount of iodine in CH2Cl2 prior to the cyclization. Compound (Z)-74 was then cyclized at 50 °C in the presence of DBU in THF affording the desired cyclized product 75 in 76% yield. Subsequent formylation was accomplished by treating with methyl orthoformate in TFA to give the formylated CD-ring component 59 in quantitative yield.49,60
Coupling reaction between the CD-ring 59 and the AB-ring 44 was carried out under acidic conditions to afford the sterically locked 15Za-18Et-BV diallyl ester derivative 76 in 87% yield. Finally, the deprotection of the allyl ester moiety of 76 was achieved by a Pd(0)-catalyzed reaction42,44 using sodium p-toluenesulfinate as a nucleophile in THF/MeOH to give the desired locked chromophore 63 (15Za-18Et-BV) in 90% yield.49,60
Toward the construction of 15Es-18Et-BV (64), 5-tosylpyrrolinone derivative 55 was prepared starting from propenal (77) as shown in Scheme 15. Treatment of 77 with acetic acid in the presence of a catalytic amount of zinc acetate afforded 3-acetoxypropanal (78) in 40% yield.85,86 Compound 78 was then coupled with 1-nitropropane according to the Henry reaction in the presence of a catalytic amount of KOH in MeOH. The resulting nitro-alcohol was then acetylated using acetic anhydride in the presence of a catalytic amount of 4-(dimethylamino)pyridine (DMAP) in THF to give the nitro-diacetate 79 in 85% yield in two steps. Compound 79 was reacted with tosylmethyl isocyanide in the presence of DBU to afford 2-tosylpyrrole derivative 80 in 59% yield. Compound 80 was then brominated with trimethylphenylammonium tribromide in CH2Cl2, followed by hydrolysis of the acetoxy group with aqueous NaOH in MeOH to give compound 81 in 72% yield in two steps. Mesylation and subsequent redox-type reaction39,47 using DMSO and zinc in TFA afforded the 5-tosylpyrrolinone derivative 55 in 55% yield in two steps.49
Compounds 55 and 33 were coupled by the Wittig-type reaction using tributylphosphine and DBU in THF to afford the cyclized E-syn CD-ring component 82 in 65% yield. Formylation of 82 with TFA and methyl orthoformate accompanied by decarboxylation gave the CD-ring component 60 in 85% yield.
Coupling reaction between the CD-ring 60 and the AB-ring 44 was carried out under acidic conditions in a similar manner as in the case of other locked chromophores described above to afford the sterically locked 15Es-18Et-BV diallyl ester derivative 83 in 45% yield. The deprotection of the allyl ester moiety of 83 by a Pd(0)-catalyzed reaction42,44 with sodium p-toluenesulfinate as a nucleophile in THF/MeOH gave locked chromophore 64 (15Es-18Et-BV) in 42% yield (Scheme 16).49,60
Locked BV derivative 65 (15Ea-18Et-BV) bearing an E-anti CD-ring component was synthesized through cyclization with an 8-membered ring as shown in Scheme 17.87
The CD-ring component 61 was prepared starting from commercially available 3,4-dihydro-2H-pyran (84). 5-Hydroxypentanal (86) obtained by acid hydrolysis of 84 was reacted with 1-nitropropane in the presence of a base according to the Henry reaction to afford nitro-alcohol 87, followed by acetylation in the presence of DMAP to give nitro-diacetate 88 along with nitro-olefin 89 in three steps. A mixture of compounds 88 and 89 was reacted with t-butyl isocyanoacetate in the presence of DBU in THF applying the Barton’s method55 to give pyrrole derivative 56 in 26% yield from 84 in four steps.
Iodination of α-position of pyrrole 56 with N-iodosuccinimide (NIS) to 90 (99% yield) and subsequent oxidation utilizing Pb(OAc)4 in toluene gave pyrrolinone derivative 91 in 91% yield. The α-acetoxy group of the pyrrolinone derivative 91 was replaced by a Ts group to afford 92 in 94% yield using anhydrous sodium p-toluenesulfinate (TsNa) in refluxing THF, followed by protection of the pyrrolinone-NH group using di-t-butyl dicarbonate (Boc2O) in MeCN to give compound 93 in 86% yield. Then, hydrolysis of the acetate group of 93 in 0.5 M methanolic HCl afforded N-protected tosylpyrrolinone derivative 94a and its regioisomer 94b, which was formed by allylic rearrangement of a tosyl group, in 97% total yield (94a/94b = ca. 1/2). Iodination of the resulting alcohol using iodine and triphenylphosphine in the presence of imidazole in MeCN afforded 95 in 89% total yield. The product 95 was converted to a mixture of tosylpyrrolinone derivatives 96a and 96b bearing a nitro group in its side chain in 60% yield by treating with sodium nitrite in the presence of phloroglucinol in DMF. The resulting nitro compound 96 was reacted with allyl 4-oxobutanoate (97) that was readily available from γ-butyrolactone (101) (Scheme 18),45,88 followed by acetylation and reaction with t-butyl isocyanoacetate to give a mixture of dipyrrole derivatives, 57a and its regioisomer 57b, in 28% total yield from 96. Compound 57 was subjected to the Vilsmeier reaction to afford a mixture of formylated dipyrrole derivatives 98a and 98b in 67% yield. Compound 98 was then treated with 99% formic acid to cleave the Boc and t-butyl esters giving dicarboxylic acid derivative, which was further subjected to the Wittig-type intramolecular coupling using tributylphosphine in the presence of DBU in THF/DMF to give the pyrromethenone derivative 99 in 49% yield. It was confirmed that isolated both isomers 98a and 98b could be converted to the same product 99, respectively, under the same reaction conditions. Compound 99 was formylated by treating with methyl orthoformate in TFA to give the desired formylated E-anti CD-ring component 61 in 63% yield.
Coupling reaction between the CD-ring 61 and the AB-ring 44 was carried out under acidic conditions to afford 15Ea-18Et-BV diallyl ester derivative 100 in 71% yield. Finally, deprotection of the allyl esters was achieved by a Pd(0)-catalyzed reaction in the presence of sodium p-toluenesulfinate (TsNa) as a nucleophile in THF/MeOH to give 65 (15Ea-18Et-BV) in 70% yield.87,89
Now, we could synthesize four different types of locked BV derivatives, simply termed 15Zs (62), 15Za (63), 15Es (64), and 15Ea (65) hereafter, in which the C15=C16 double bond is locked in either the Z or E configuration and the C14–C15 single bond in either the syn or anti conformation by cyclizing with an additional carbon chain as described above.
5. IN VITRO AND IN VIVO ASSEMBLY OF THE SYNTHESIZED LOCKED BILIN CHROMOPHORES WITH APOPHYTOCHROMES
To understand how the structure of chromophore is linked with the conformation of the protein, the synthesized locked BV derivatives 62–65 were assembled in vitro with Agrobacterium phytochrome
Agp1 by German biologists as collaborative work. Biliverdin (BV), the natural chromophore of Agp1, and 18EtBV (Figure 5) were used as controls. Both BV and 18EtBV chromophores completely formed a covalent bond between 1 and 5 min and produced adducts that show typical red/far-red reversible photoconversion (Figure 9a, b).90
All locked BV derivatives also bound covalently to the protein, which was confirmed by using SDS-denaturation/dissociation, followed by separation of protein and the free bilin on desalting columns, and the binding was completed between 1 and 5 min except 15Ea that required about 140 min, producing adducts with characteristic spectral properties. The 15Za adduct is spectrally similar to the Pr form (Figure 9c), and the 15Ea adduct is similar to the Pfr form (Figure 9e) of the BV adduct. Thus, the chromophore of Agp1 adopts a C15=C16 Z configuration and a C14–C15 anti conformation in the Pr form and a C15=C16 E configuration and a C14–C15 anti conformation in the Pfr form. Both the 15Zs and the 15Es adducts absorbed only in the blue region of the visible spectra (Figure 9d, f).90
The results with the 15Zs and 15Es chromophores show that the Agp1 protein is rather tolerant for bilins that have the “wrong” conformation. Both locked syn bilins might adopt a helical conformation within the Agp1 chromophores pocket as compared with the stretched conformation of other bilins. Another possibility could be that the conjugation system of tetrapyrrole chromophore is broken by a nucleophilic attack of an amino acid side chain to the C10 position. However, the addition reaction must be reversible, because the red absorption band appeared again upon SDS treatment of the holoprotein, as found during our tests for covalent binding. We observed a related phenomenon using doubly locked BV derivatives.88
The covalent bond with 15Ea formed rather slowly as compared with the other chromophores. It could be that after the rapid incorporation of 15Ea into the Agp1 chromophore pocket, the distance between the Cys-20 thiol group and the A-ring vinyl side chain of 15Ea is rather large and that the likelihood for covalent bond formation is thereby decreased. In this model, thermal conformational motions of the protein and/or the chromophore would be required for covalent bond formation. For the chromophore, a syn/anti rotation around the C5–C6 single bond is the most likely option. Once the E-isomer is present, the protein adopts its conformation in a proper manner.
It has been shown that the first step in the Pr to Pfr photoconversion of plant phytochromes is a Z to E isomerization around the C15=C16 double bond.23 Because of the spectral similarities, this mechanism was most likely universal for all phytochromes, although direct experimental evidence was lacking. If Agp1 follows the same mechanism, all adducts with locked chromophores should remain stable in the light. Indeed, we did not observe any spectral changes upon irradiation with either red or white light (Figure 9c–f) under conditions that induced saturating conversions of the BV and 18EtBV adducts.
The Pr/Pfr photocycle is associated with conformational change of the protein that have been measured by using size exclusion chromatography (SEC), CD spectroscopy, or limited proteolysis.91–93 Most bacterial phytochromes are histidine kinases, in which kinase activity is modulated by light-induced conformational changes of the protein. Kinase activity is generally stronger in the Pr and weaker in the Pfr form, although other phosphorylation patterns have also been found.8,11,76,94
Mobility of proteins subjected to SEC was dependent on the shape and quaternary structure of the molecules. All chromophore adducts with Agp1-M15 (the N-terminal 504 amino acids of Agp1) apoprotein were analyzed by using SEC, and also histidine kinase activity to probe for protein conformation.90 In either case, the 15Za adduct behaved like the Pr, and the 15Ea adduct behaved like the Pfr form of Agp1. Replacing the natural chromophore with a locked 15Ea derivative can thus bring phytochrome holoprotein in the Pfr form in the dark. In this way, physiological action of Pfr could be studied in vivo and separated from Pr/Pfr cycling and other light effects by using locked PCB derivatives as will be described later.
Agp1 and Agp2 have antagonistic properties: in the dark, Agp1 converts slowly from Pfr to Pr, whereas Agp2 converts slowly from Pr to Pfr. To see whether and how locked chromophores are incorporated into Agp2, the set of the above mentioned chromophores was also tested with purified recombinant Agp2.95 Interesting differences between Agp1 and Agp2 and between different chromophores were found for the assembly rates. The slowest assembly with Agp1 was found for the 15Ea chromophore. In the case of Agp2, the 15Ea assembly rate is faster than that of 15Za. The assembly rates for 15Ea/15Za for both phytochromes are in line with the finding that Agp1 adopts a Pr form and Agp2 adopts a Pfr form in the dark.95
For analysis of stereochemistry of the C5–C6 single bond, new locked chromophores 5Zs and 5Za, in which C4=C5 double bond was fixed in a Z configuration and the C5–C6 single bond was fixed in either a syn or anti conformation (Figure 10), were synthesized in a similar manner described above for the chromophores locked at the C15 position. They were also used for assembly experiments with Agp1 and Agp2 and provided deeper insight into the similarities and differences between Agp1 and Agp2, giving further clues for the understanding of chromophore stereochemistry in the ground state and during photoconversion. 95
Results of the assembly experiments with the 5Zs and 5Za chromophores are summarized as follows:95 (1) Both chromophores form covalent bonds with either Agp1 or Agp2. (2) The spectra and the absorption maxima of the 5Zs adducts are similar to those of the Pr form of the 18EtBV control. (3) The absorption spectra of the 5Za adducts are also similar to Pr, but their maxima are shifted to shorter wavelengths. (4) Photoconversion shifted the absorption maxima of the 5Zs adducts to shorter wavelengths, whereas the 5Za adducts were shifted to longer wavelengths. Thus, the C5–C6 single bond of the Pfr chromophore is in an anti conformation, supporting the previous suggestion that during photoconversion of phytochromes, a rotation around the C5–C6 single bond connecting the A- and B-rings occurs,28 though direction of the rotation was opposite, and the 5Za photoproducts are spectrally not exactly identical with Pfr. All of the data that were obtained with these chromophores lead to the conclusion that a flexibility around the A- and B-rings connecting methine bridge is essential for proper photoconversion from Pr to Pfr and for Pr to Pfr dark conversion of Agp2, although the role of C15=C16 Z to E isomerization as the initial step is beyond doubt from our results.
The spectral analogy between Pr and the 5Zs adducts was in line with the data from the crystal structure of phytochrome reported.78,96 In their structures, the configuration and conformation of the chromophores were found to be 5Zs/10Zs/15Za, i.e., the single bond between the A- and B-rings, is in the syn conformation.
Furthermore, in 5Zs-Agp1 adduct the photoconversion proceeded via the Lumi-R intermediate to Meta-RA, but the following millisecond-transition to Meta-RC was blocked. Consistently, no transient proton release was detected. The photoconversion of 5Zs-Agp1 is apparently arrested in a Meta-RA-like intermediate, since the subsequent syn to anti rotation around the C5–C6 single bond is prevented by the lock. The Meta-RA-like photoproduct was characterized by its distinctive CD spectrum suggesting a reorientation of the ring D.97
Based on the above results, we proposed that the stereochemistry of the Agp1 and Agp2 chromophores is 5Zs/10Zs/15Za in the Pr and 5Za/10Zs/15Ea or 5Ea/10Zs/15Ea in the Pfr form.95 A schematic presentation of the proposed stereochemistry of Pr and Pfr is given in Figure 11,95 taking into account the data from the crystal structure of the chromophore-binding domain of the bacteriophytochromes.78,96,98
As mentioned above, assembly of the sterically locked chromophores with apophytochrome could afford photostable Pr- and Pfr-like adducts. This fact prompted us to use the adducts of the locked chromophores with Agp1 for crystallization analysis carried out with German collaborators.100
Because of the difficulty to grow X-ray suitable crystals of full-length Agp1, our studies were focused on the truncated N-terminal photosensory chromophore module of Agp1, termed Agp1-M15 for the first crystallization experiments and preliminary X-ray analysis.100 This 54 kDa-fragment consists of the N-terminal 504 amino acids of Agp1 and contains the PAS-like domain (PLD), GAF domain and PHY domain but lacks the C-terminal histidine-kinase module.
The protein Agp1-M15 was either assembled with the natural chromophore biliverdin (BV) or a sterically locked synthetic BV derivative 15Za. Both adducts could be crystallized, but the resolution was largely improved by the use of 15Za.
In general, for crystal inspection under the microscope, light was passed through an interference filter with a maximum transmission at 520 nm. To obtain information about the color of the crystals and to test for stability against light, some crystals were inspected under white light. The crystals of BV-Agp1-M15, which were obtained by assembling natural BV chromophore with Agp1-M15, cracked during irradiation with white light at 293 K, and the green color gradually disappeared over time, showing that the holoprotein inside the crystal still undergoes light-induced conformational changes.
On the other hand, a locked chromophore 15Za adduct of Agp1-M15 did not undergo light-induced conformational changes, because the first step of the photocycle, the Z/E isomerization around the C15=C16 double bond, is not possible. The sharper bands of the Vis spectrum of 15Za-Agp1-M15 than that of BV-Agp1-M15 suggested that 15Za is less flexible within the chromophore pocket as compared with BV. Thus, by using 15Za-Agp1-M15 for crystallization, we could both improve the quality of the crystals and allow for continuous work under laboratory light. The rigidity of the chromophore pocket might be one reason for the improved resolution that was obtained for these crystals. Interestingly, crystals of both the BV adduct and the 15Za adduct were obtained under similar conditions, belong to the same space group, and have similar unit cell parameters, indicating similar crystal packing and comparable overall conformations of the different adducts. However, we cannot yet determine the detailed structure of the chromophore in the holophytochrome.101
Most information on the configuration and conformation of the chromophore is now available for the Pr and Pfr forms from the crystal structures of other phytochromes,78,96,98,102–105 however, detailed knowledge of configurational and conformational changes of the chromophore that are reflected by spectral changes is still lacking.
For studies with plant phytochromes, the BV-based chromophores are inappropriate. Therefore, we recently established the syntheses of locked phycocyanobilin (PCB) derivatives, termed 15Z-anti PCB (15Za-PCB) and 15E-anti PCB (15Ea-PCB) according to a retrosynthetic analysis shown in Figure 12, which is similar to the method described for locked BV derivatives.89 Such chromophores might be covalently integrated into the chromophore pocket of plant phytochromes, because of their ethylidene side chain, and thus fulfill the required properties.
We assembled the locked 15Za-PCB (103) and 15Ea-PCB (104) in vitro and in vivo with phytochrome in Ceratodon purpureus and Arabidopsis thaliana.106
Recombinant bacterial and plant phytochromes incorporated either chromophore in a noncovalent or covalent manner. All adducts were photoinactive as in the case of 15Za-18Et-BV and 15Ea-18Et-BV. The absorption spectra of the 15Za-PCB and 15Ea-PCB adducts were comparable with those of the Pr and Pfr form, respectively. Feeding of 15Ea-PCB, but not 15Za-PCB, to protonemal filaments of the moss Ceratodon purpureus resulted in increased chlorophyll accumulation, modulation of gravitropism, and induction of side branches in darkness. The effect of locked chromophores on phytochrome responses, such as induction of seed germination, inhibition of hypocotyl elongation, induction of cotyledon opening, randomization of gravitropism, and gene regulation, were investigated in wild-type Arabidopsis thaliana and the phytochrome-chromophore-deficient long hypocotyl mutant hy1. All phytochrome responses were induced in darkness by 15Ea-PCB, not only in the mutant but also in the wild type. These studies show that the 15Ea stereochemistry of the chromophore results in the formation of active Pfr-like phytochrome in the cell.106
Based on these results, the locked PCB chromophores might be used to investigate phytochrome responses in many other organisms without the need to isolate mutants. A further goal could be to use the locked PCB chromophores in agriculture and horticulture to induce phytochrome responses in crop plants.
6. DEVELOPMENT OF GENERAL SYNTHETIC METHOD OF DOUBLY LOCKED BILIN CHROMOPHORES
To confirm the structure of a chromophore in Pr form of bacteriophytochromes, we synthesized a doubly locked 5Zs15Za-BV (105) according to the retrosynthetic analysis shown in Figure 13.88
The Z-anti AB-ring component 106 was obtained from 39 by cyclizing with BrCH2CH2Br in the presence of nBu4NBr, followed by introducing a vinyl group via oxidation and elimination of the tolylthio group in 107, and subsequent decarboxylation of the resulting compound 108 with TFA (Scheme 19).88
Coupling between the CD-ring component 59 and the AB-ring component 106 was accomplished under acidic conditions to afford 5Zs15Za-BV diallyl ester derivative 109 in 90% yield. Final deprotection of the allyl esters was achieved by a Pd(0)-catalyzed reaction in the presence of sodium p-toluenesulfinate (TsNa) as a nucleophile in THF/MeOH to give 105 (5Zs15Za-BV) in 70% yield (Scheme 20).88
To our knowledge, there is as yet no evidence of a C4=C5 E configuration of the Pfr chromophore. However, such configuration might have been overlooked for chemical or technical reasons. Therefore, four different types of doubly locked biliverdin (BV) derivatives, 5Zs15Ea-BV (110), 5Za15Ea-BV (111), 5Es15Ea-BV (112), and 5Ea15Ea-BV (113), were synthesized for the first time in free acid forms to elucidate the stereochemistry of phytochrome chromophore in Pfr form according to the retrosynthetic analyses shown in Figure 14.107 The CD-ring component 114 was readily available by decarboxylation of an E-anti CD-ring component 99 with TFA (Scheme 21).
The synthesized doubly locked bilin chromophores, 105 corresponding to Pr form and 110–113 as candidates for the chromophore corresponding to Pfr form, were assembled with Agp1 and Agp2 apophytochromes. The absorption spectra of doubly locked 5Zs15Za-BV (105) adducts resembled the spectrum of natural BV-binding phytochrome in Pr form as expected.88,95,108
Furthermore, we found that the absorption spectra of 5Za15Ea-BV (111) or 5Ea15Ea-BV (113) adducts resembled the spectrum of Pfr form of natural Agp1 and Agp2, though the synthesized doubly locked chromophores 111 and 113 have no vinyl group on the A-ring necessary to form a covalent bond with the apoproteins.95,109
To investigate the structure and function of the BV chromophores in Pfr form, we further synthesized a new doubly locked BV derivative, 5Za15Ea(7)-BV (116), which carries a vinyl group at the A-ring necessary to form a covalent bond with a Cys residue of apophytochrome. The CD-ring component was locked in E-anti stereochemistry by a 7-membered ring, different from the previous one locked by an 8-membered ring, according to the retrosynthetic analysis shown in Figure 15.110
The locked Z-anti AB-ring component 118 was synthesized in a similar manner described above for the locked Z-anti CD-ring component 59 according to Scheme 22.49,60 Tosylpyrrolinone 38 as the A-ring precursor and formylpyrrole 54 as the B-ring precursor here were coupled by our original Wittig-type reaction to give dipyrrole 119 as a mixture of E- and Z-isomers. The E-isomer was converted to the Z-isomer by treating with a catalytic amount of iodine in CH2Cl2. (Z)-119 thus obtained was treated with DBU in THF at 50 ˚C to afford the desired cyclized product 120 in 80% yield. After oxidation of the tolythio group on the A-ring, a vinyl group was introduced by refluxing the resulting sulfoxide in DMF under basic conditions to afford the locked Z-anti AB-ring component 121. By treating with TFA, the decarboxylated AB-ring component 118 was obtained in situ (Scheme 22).110
On the other hand, though we have synthesized an E-anti CD-ring component locked with an 8-membered ring,87,89 this method still remained some unsatisfactory points due to the linear synthetic manner. Therefore, a new convergent method was established for the synthesis of the E-anti CD-ring component 117 locked with a 7-membered ring.110
We recently developed the synthesis of 4-formylpyrrole 122 as the C-ring precursor via selective oxidation of pyrrole 32a (R’ = CH2CH2CO2Allyl) with DDQ in the presence of MeOH (Scheme 23).111
4-(Acetoxymethyl)pyrrolinone 127 as the D-ring precursor was prepared starting from ethyl glyoxalate (123), which was coupled with 1-nitropropane via the Henry reaction. After acetylation of the resulting nitro-alcohol, the obtained mixture of nitro-acetate and nitro-olefin was converted to the corresponding pyrrole-3-carboxylate 124 by applying the Barton’s method55 with tosylmethyl isocyanide.54 Pyrrole-3- carboxylate 124 was then reduced to the corresponding alcohol with lithium aluminum hydride (LAH), followed by acetylation with acetic anhydride in the presence of a catalytic amount of DMAP to afford 125. After α-bromination of 125, it was tried to convert to the corresponding tosylpyrrolinone by our previous redox reaction using TFA, DMSO and Zn metal. However, it was failed. Therefore, we directly oxidized 125 with mCPBA to 5-tosylpyrrolinone 126 in 70% yield, followed by reductive elimination of the tosyl group with NaBH4 to afford the 5-unsubstituted pyrrolinone 127 as the D-ring precursor (Scheme 24).110
The D-ring precursor 127 bearing an acetoxyl group at the allylic position was activated by a catalytic amount of Pd(0)112 to react with tributylphosphine generating the phosphonium intermediate, which was further converted to the corresponding ylide by treatment with butyl lithium at low temperature to couple with 4-formylpyrrole 122 via the Wittig olefination reaction to afford the product 128 as a mixture of E- and Z-isomers. At this stage, an allyl ester group in the C-ring precursor 122 was changed to a methyl ester group under basic conditions in MeOH. The E/Z mixture of olefin 128 was reduced by hydrogenation to afford 129 in 90% yield. After treating with TFA and methyl orthoformate, the resulting α,α’-diformylated product 131 was cyclized with DBU to afford an E-anti CD-ring component 117 locked with a 7-membered ring in 50% yield (Scheme 25).
The locked Z-anti AB-ring 118 and E-anti CD-ring 117 were coupled with TFA in MeOH to construct the doubly locked 5Za15Ea(7)-BV allyl methyl ester 132, which was further hydrolyzed under acidic conditions to give the doubly locked 5Za15Ea(7)-BV (116) in free acid form (Scheme 25).
As described above, we succeeded to prepare the doubly locked 5Za15Ea(7)-BV (116) corresponding to the chromophore in Pfr form of Agp1 by a new convergent synthetic method. Development of a convenient method for the synthesis of the locked E-anti CD-ring component such as 117 is essential for the synthesis of the chromophore corresponding to the Pfr form. We are now studying on assembly of the doubly locked bilin chromophores synthesized above with phytochrome apoproteins in vitro and in vivo.
7. CONCLUSION
We could establish an efficient and flexible general method for the preparation of the linear tetrapyrrole (bilin) chromophores to investigate the structure and function of phytochrome chromophores by developing several new reactions, i.e., (1) rearrangement of the tosyl group of 2-tosylpyrroles to the 5-position under mild acidic conditions, (2) efficient transformation of 2-bromo-5-tosylpyrroles to the corresponding 5-tosylpyrrolinones by a redox reaction, (3) the Wittig-type coupling reaction between 5-tosylpyrrolinone and 2-formylpyrrole for the preparation of the AB- and CD-ring components, (4) reductive transformation of the AB-ring component of BV to the AB-ring component of PΦB and PCB, and (5) protection and palladium-catalyzed deprotection of propanoic acid side chains at the C8 and C12 positions via allyl esters.
This general method made it possible to synthesize not only PΦB and PCB as natural chromophores used in plants and cyanobacteria, but also BV used in other bacteria. Furthermore, various unnatural types of bilin chromophores bearing modified side chain(s) and sterically locked BV and PCB derivatives could be prepared in free acid forms by applying the present synthetic method.
Assembly experiments of the synthesized bilin chromophores with recombinant Arabidopsis phytochrome PhyB apoprotein (PHYB) in vitro provided insights into the structure and function of phytochromes, i.e., the different role of each substituents on four pyrrole rings of the bilin chromophore in plant phytochrome: (1) the A-ring acts mainly as the anchor for ligation to an apophytochrome, (2) hydrophobic environment and open space accommodate the A- and D-rings of the bilin chromophore at the binding site, (3) stereochemistry at the C2 position of the chromophore of plant phytochrome is not crucial, (4) the side chains of the B- and C-rings are crucial to position the chromophore properly in the chromophore pocket and for photoreversible spectral changes, (5) a propanoic acid side chain at the C8 position of the bilin chromophore is more crucial for photoreversible conversion of phytochrome than that at the C12 position, (6) the side-chain structure of the D-ring is required for the photoreversible spectral change of adducts, and the environment around the C18 position of the chromophore within the chromophore-biding pocket is more flexible than that around the C17 position.
Based on these results, we could outline the order of events during in vitro assembly of PHYB with its chromophore as shown in Figure 7.
In vitro assembly with Agrobacterium phytochrome Agp1 and/or Agp2 revealed the following: (1) BV chromophore in Agp1 covalently binds to the apoprotein via the A-ring vinyl side chain, (2) the stereochemistry of the Agp1 and Agp2 chromophores is most likely to be 5Zs/10Zs/15Za in the Pr and 5Za/10Zs/15Ea or 5Ea/10Zs/15Ea in the Pfr form, respectively, (3) photoinsensitive single crystals of Pr with a 15Za-locked chromophore were obtained for X-ray crystallographic analysis of the N-terminal photosensory module of phytochrome Agp1.
On the other hand, by feeding synthetic bilins exogenously to Arabidopsis hy1 and hy2 mutant seedlings in vivo, a double bond in the vinyl side chain at the C18 position of the D-ring of PΦB was found to be crucial for the photosensory function of PhyA, whereas PhyB function was restored with both PΦB and PCB with a saturated D-ring substituent.
Furthermore, in vivo assembly experiments with the locked PCB derivatives provided very important new insights into the structure and function of phytochromes, i.e., phytochrome responses are induced in darkness by 15EaPCB, not only in the mutant but also in the wild type: (1) feeding of 15EaPCB, but not 15ZaPCB, to protonemal filaments of the moss Ceratodon purpureus resulted in increased chlorophyll accumulation, modulation of gravitropism, and induction of side branches in darkness, (2) the effect of locked chromophores on phytochrome responses, such as induction of seed germination, inhibition of hypocotyl elongation, induction of cotyledon opening, randomization of gravitropism, and gene regulation, were also observed in wild-type Arabidopsis thaliana and the phytochrome-chromophore-deficient long hypocotyl mutant hy1.
These studies show that the 15Ea stereochemistry of the chromophore results in the formation of active Pfr-like phytochrome in the cell.
We have recently succeeded to synthesize the doubly locked BV and PCB chromophores. Such locked PCB chromophores would allow us to investigate phytochrome responses in vivo in detail. A further goal could be to use the locked PCB chromophores in agriculture and horticulture to induce phytochrome responses in crop plants.
From the results described above, it is obvious that an approach based on the synthetic organic chemistry toward the elucidation of the structure and function of phytochromes and also toward the related studies113–115 is very effective and necessary. Especially, sterically locked chromophores will further open the new avenues for investigation of phytochrome chromophores both in vitro and in vivo in future.
ACKNOWLEDGEMENTS
Author is very grateful to Professors H. Kinoshita, Y. Ukaji, M. Furuya, T. Lamparter, and N. Krauß, Drs. H. Hanzawa and Y. Murata, and other colleagues and students whose names are in the references, for the synthetic, biological, and crystallographic experiments and discussions.
The present work was financially supported in part by Grant-in-Aid for Scientific Research (Nos. 06640685, 07640715, 11440187) and Grant-in-Aid for Scientific Research on Priority Areas (13029041, 14044033) from The Ministry of Education, Culture, Sports, Science and Technology (MEXT), Japan, and Grand-in-Aid for Scientific Research (Nos. 15350021, 19350082) from Japan Society for the Promotion of Science (JSPS).
References
1. W. Rüdiger and F. Thümmler, ‘Photomorphogenesis in Plants,’ 2nd ed., ed. by R. E. Kendrick and G. H. M. Kronenberg, Kluwer Academic Publishers, Dordrecht, The Netherlands, 1994.
2. M. M. Neff, C. Frankhauser, and J. Chory, Genes Dev., 2000, 14, 257.
3. C. Fankhauser, J. Biol. Chem., 2001, 276, 11453. CrossRef
4. M. Wada, K. Shimazaki, and M. Iino, ‘Light Sensing in Plants,’ Springer-Verlag, Tokyo, 2005. CrossRef
5. N. C. Rockwell, Y.-S. Su, and J. C. Lagarias, Annu. Rev. Plant Biol., 2006, 57, 837. CrossRef
6. H. A. Borthwick, S. B. Hendricks, M. W. Parker, E. H. Toole, and V. K. Toole, Proc. Natl. Acad. Sci. U.S.A., 1952, 38, 662. CrossRef
7. W. L. Butler, K. H. Norris, H. W. Siegelman, and S. B. Hendricks, Proc. Natl. Acad. Sci. U.S.A., 1959, 45, 1703. CrossRef
8. K. C. Yeh, S. H. Wu, J. T. Murphy, and J. C. Lagarias, Science, 1997, 277, 1505. CrossRef
9. T. Lamparter, F. Mittmann, W. Gärtner, T. Börner, E. Hartmann, and J. Hughes, Proc. Natl. Acad. Sci. U.S.A., 1997, 94, 11792. CrossRef
10. S. J. Davis, A. V. Vener, and R. D. Vierstra, Science, 1999, 286, 2517. CrossRef
11. S. H. Bhoo, S. J. Davis, J. Walker, B. Karniol, and R. D. Vierstra, Nature, 2001, 414, 776. CrossRef
12. H. Falk, ‘The Chemistry of Linear Oligopyrrole and Bile Pigment,’ Springer Verlag, New York, 1989. CrossRef
13. W. Rüdiger and F. Thümmler, Angew. Chem., Int. Ed. Engl., 1991, 30, 1216. CrossRef
14. M. Furuya and P.-S. Song, ‘Assembly and Properties of Holophytochrome in Photomorphogenesis in Plants,’ 2nd ed., ed. by R. E. Kendrick and G. H. M. Kronenberg, Kluwer Academic Publishers, Dordrecht/Boston/London, 1994, Cap. 4.3, pp. 105–140.
15. M. Stanek and K. Grubmayr, Chem. Eur. J., 1998, 4, 1653; CrossRef 1998, 4, 1660. CrossRef
16. T. Lamparter, FEBS Lett., 2004, 573, 1. CrossRef
17. N. C. Rockwell and J. C. Lagarias, Plant Cell, 2006, 18, 4. CrossRef
18. J. C. Lagarias and H. Rapoport, J. Am. Chem. Soc., 1980, 102, 4821. CrossRef
19. N. Frankenberg, K. Mukougawa, T. Kohchi, and J. C. Lagarias, Plant Cell, 2001, 13, 965.
20. T. Hübschmann, T. Börner, E. Hartmann, and T. Lamparter, Eur. J. Biochem., 2001, 268, 2055. CrossRef
21. A. Blumenstein, K. Vienken, R. Tasler, J. Purschwitz, D. Veith, N. Frankenberg-Dinkel, and R. Fischer, Curr. Biol., 2005, 15, 1833. CrossRef
22. I. Oberpichler, I. Molina, O. Neubauer, and T. Lamparter, FEBS Lett., 2006, 580, 437. CrossRef
23. W. Rüdiger, F. Thümmler, E. Cmiel, and S. Schneider, Proc. Natl. Acad. Sci. U.S.A., 1983, 80, 6244. CrossRef
24. A. T. Ulijasz, G. Cornilescu, C. C. Cornilescu, J.-R. Zhang, M. Rivera, J. L. Markley, and R. D. Vierstra, Nature, 2010, 463, 250. CrossRef
25. Y. Mizutani, S. Tokutomi, and T. Kitagawa, Biochemistry, 1994, 33, 153. CrossRef
26. C. Kneip, P. Hildebrandt, W. Schlamann, S. E. Braslavsky, F. Mark, and K. Schaffner, Biochemistry, 1999, 38, 15185. CrossRef
27. F. Andel, III, J. T. Murphy, J. A. Haas, M. T. McDowell, I. van der Hoef, J. Lugtenburg, J. C. Lagarias, and R. A. Mathies, Biochemistry, 2000, 39, 2667. CrossRef
28. M. A. Mroginski, D. H. Murgida, D. von Stetten, C. Kneip, F. Mark, and P. Hildebrandt, J. Am. Chem. Soc., 2004, 126, 16734. CrossRef
29. J. R. Wagner, J. Zhang, J. S. Brunzelle, R. D. Vierstra, and K. T. Forest, J. Biol. Chem., 2007, 282, 12298. CrossRef
30. L. Li and J. C. Lagarias, J. Biol. Chem., 1992, 267, 19204.
31. P. Schmidt, U. H. Westphal, K. Worm, S. E. Braslavsky, W. Gärtner, and K. Schaffner, J. Photochem. Photobiol. B, 1996, 34, 73, and references cited therein. CrossRef
32. J. T. Murphy and J. C. Lagarias, Photochem. Photobiol., 1997, 65, 750. CrossRef
33. L. Deforce, M. Furuya, and P.-S. Song, Biochemistry, 1993, 32, 14165. CrossRef
34. S. H. Bhoo, T. Hirano, H.-Y. Jeong, J.-G. Lee, M. Furuya, and P.-S. Song, J. Am. Chem. Soc., 1997, 119, 11717. CrossRef
35. A. Remberg, P. Schmidt, S. E. Braslavsky, W. Gärtner, and K. Schaffner, Eur. J. Biochem., 1999, 266, 201. CrossRef
36. A. Gossauer and W. Hirsch, Justus Liebigs Ann. Chem., 1974, 1496. CrossRef
37. A. Gossauer and R.-P. Hinze, J. Org. Chem., 1978, 43, 283. CrossRef
38. J.-P. Weller and A. Gossauer, C hem. Ber., 1980, 113, 1603. CrossRef
39. H. Kinoshita, Y. Hayashi, Y. Murata, and K. Inomata, Chem. Lett., 1993, 1437. CrossRef
40. H. Kinoshita, H. Ngwe, K. Kohori, and K. Inomata, Chem. Lett., 1993, 1441. CrossRef
41. K. Kohori, M. Hashimoto, H. Kinoshita, and K. Inomata, Bull. Chem. Soc. Jpn., 1994, 67, 3088. CrossRef
42. T. Kakiuchi, H. Kato, K. P. Jayasundera, T. Higashi, K. Watabe, D. Sawamoto, H. Kinoshita, and K. Inomata, Chem. Lett., 1998, 1001. CrossRef
43. K. P. Jayasundera, H. Kinoshita, and K. Inomata, Chem. Lett., 1998, 1227. CrossRef
44. T. Kakiuchi, H. Kinoshita, and K. Inomata, Synlett, 1999, 901. CrossRef
45. A. Ohta, D. Sawamoto, K. P. Jayasundera, H. Kinoshita, and K. Inomata, Chem. Lett., 2000, 492. CrossRef
46. D. Sawamoto, H. Nakamura, H. Kinoshita, S. Fujinami, and K. Inomata, Chem. Lett., 2000, 1398, see also the references cited therein. CrossRef
47. S. Takeda, K. P. Jayasundera, T. Kakiuchi, H. Kinoshita, and K. Inomata, Chem. Lett., 2001, 590. CrossRef
48. K. P. Jayasundera, H. Kinoshita, and K. Inomata, Bull. Chem. Soc. Jpn., 2000, 73, 497. CrossRef
49. M. A. S. Hammam, H. Nakamura, Y. Hirata, H. Khawn, Y. Murata, H. Kinoshita, and K. Inomata, Bull. Chem. Soc. Jpn., 2006, 79, 1561. CrossRef
50. K. Inomata, Bull. Chem. Soc. Jpn., 2008, 81, 25, and references cited therein. CrossRef
51. M. Nasal, Liebigs Ann. Chem., 1983, 1510. CrossRef
52. M. Nasal, J. Am. Chem. Soc., 1984, 106, 7540. CrossRef
53. T. Masukawa, H. Kato, T. Kakiuchi, K. P. Jayasundera, H. Kinoshita, and K. Inomata, Chem. Lett., 1998, 27, 455. CrossRef
54. B. E. Hoogenboon, O. H. Oldenziel, and A. M. van Leusen, Org. Synth., 1988, Coll. Vol. VI, 987.
55. D. H. R. Barton, J. Kervogoret, and S. Z. Zard, Tetrahedron, 1990, 46, 7587. CrossRef
56. K. Kohori, H. Kinoshita, and K. Inomata, Chem. Lett., 1995, 799. CrossRef
57. A. Hiyoshi, K. Kohori, H. Kato, H. Kinoshita, and K. Inomata, 1997, unpublished data.
58. A. Gossauer and M. Blacha-Puller, Liebigs Ann. Chem., 1981, 1492. CrossRef
59. A. Gossauer, F. Nydegger, E. Benedikt, and H.-P. Köst, Helv. Chim. Acta, 1989, 72, 518. CrossRef
60. M. A. S. Hammam, Y. Murata, H. Kinoshita, and K. Inomata, Chem. Lett., 2004, 33, 1258. CrossRef
61. H. Kunz and C. Unverzagt, Angew. Chem., Int. Ed. Engl., 1984, 23, 436. CrossRef
62. P. H. Quail, Nature Rev. Mol. Cell Biol., 2002, 3, 85. CrossRef
63. T. Shinomura, A. Nagatani, H. Hanzawa, M. Kubota, M. Watanabe, and M. Furuya, Proc. Natl. Acad. Sci. U.S.A., 1996, 93, 8129. CrossRef
64. T. Shinomura, K. Uchida, and M. Furuya, Plant Physiol., 2000, 122, 147. CrossRef
65. H. Hanzawa, K. Inomata, H. Kinoshita, T. Kakiuchi, K. P. Jayasundera, D. Sawamoto, A. Ohta, K. Uchida, K. Wada, and M. Furuya, Proc. Natl. Acad. Sci. U.S.A., 2001, 98, 3612; CrossRef a related paper was reported: U. Robben, I. Lindner, W. Gärtner, and K. Schaffner, Angew. Chem. Int. Ed., 2001, 40, 1048. CrossRef
66. T. D. Elich and J. C. Lagarias, J. Biol. Chem., 1989, 264, 12902.
67. J. A. Wahleithner, L. M. Li, and J. C. Lagarias, Proc. Natl. Acad. Sci. U.S.A., 1991, 88, 10387. CrossRef
68. L. Deforce, K. Tomizawa, N. Ito, D. Farrens, P.-S. Song, and M. Furuya, Proc. Natl. Acad. Sci. U.S.A., 1991, 88, 10392. CrossRef
69. R. A. Sharrock and P. H. Quail, Genes Dev., 1989, 3, 1745. CrossRef
70. T. Clack, S. Mathews, and R. A. Sharrock, Plant Mol. Biol., 1994, 25, 413. CrossRef
71. S. J. Davis, J. Kurepa, and R. D. Vierstra, Proc. Natl. Acad. Sci. U.S.A., 1999, 96, 6541. CrossRef
72. T. Muramoto, T. Kohchi, A. Yokota, I. Hwang, and H. M. Goodman, Plant Cell, 1999, 11, 335.
73. T. Kohchi, K. Mukougawa, N. Frankenberg, M. Masuda, A. Yokota, and J. C. Lagarias, Plant Cell, 2001, 13, 425.
74. B. M. Parks and P. H. Quail, Plant Cell, 1991, 3, 1177.
75. H. Hanzawa, T. Shinomura, K. Inomata, T. Kakiuchi, H. Kinoshita, K. Wada, and M. Furuya, Proc. Natl. Acad. Sci. U.S.A., 2002, 99, 4725. CrossRef
76. T. Lamparter, N. Michael, F. Mittmann, and B. Esteban, Proc. Natl. Acad. Sci. U.S.A., 2002, 99, 11628. CrossRef
77. T. Lamparter, N. Michael, O. Caspani, T. Miyata, K. Shirai, and K. Inomata, J. Biol. Chem., 2003, 278, 33786. CrossRef
78. J. R. Wagner, J. S. Brunzelle, K. T. Forest, and R. D. Vierstra, Nature, 2005, 438, 325. CrossRef
79. A. R. Holzwarth, E. Venuti, S. E. Braslavsky, and K. Schaffner, Biochim. Biophys. Acta, 1992, 1140, 59. CrossRef
80. S. Rentsch, G. Hermann, M. Bischoff, D. Strehlow, and M. Rentsch, Photochem. Photobilol., 1997, 66, 585. CrossRef
81. K. Heyne, J. Herbst, D. Stehlik, B. Esteban, T. Lamparter, J. Hughes, and R. Diller, Biophys. J., 2002, 82, 1004. CrossRef
82. C. F. Zhang, D. L. Farrens, S. C. Bjorling, P. S. Song, and D. S. Kliger, J. Am. Chem. Soc., 1992, 114, 4569. CrossRef
83. J. J. van Thor, B. Borucki, W. Crielaard, H. Otto, T. Lamparter, J. Hughes, K. J. Hellingwerf, and M. P. Heyn, Biochemistry, 2001, 40, 11460. CrossRef
84. B. Borucki, D. von Stetten, S. Seibeck, T. Lamparter, N. Michel, M. A. Mroginski, H. Otto, D. H. Murgida, M. P. Heyn, and P. Hildebrandt, J. Biol. Chem., 2005, 280, 34358. CrossRef
85. R. Tsumura, M. Kanemaru, and N. Ishi, Japan Kokai Tokkyo Koho, 7505315, 1975 (Chem. Abstr., 1975, 83, 27573q).
86. T. Urasaki and W. Funakoshi, Japan Kokai Tokkyo Koho, 76131817, 1976 (Chem. Abstr., 1977, 86, 120786h).
87. H. Kinoshita, M. A. S. Hammam, and K. Inomata, Chem. Lett., 2005, 34, 800. CrossRef
88. L.-Y. Chen, H. Kinoshita, and K. Inomata, Chem. Lett., 2009, 38, 602. CrossRef
89. K. Nishiyama, A. Kamiya, M. S. A. Hammam, H. Kinoshita, S. Fujinami, Y. Ukaji, and K. Inomata, Bull. Chem. Soc. Jpn., 2010, 83, 1309. CrossRef
90. K. Inomata, M. A. S. Hammam, H. Kinoshita, Y. Murata, H. Khawn, S. Noack, N. Michael, and T. Lamparter, J. Biol. Chem., 2005, 280, 24491. CrossRef
91. J. C. Lagarias and F. M. Mercurio, J. Biol. Chem., 1985, 260, 2415.
92. E. Chen, V. N. Lapko, P. S. Song, and D. S. Kliger, Biochemistry, 1997, 36, 4903. CrossRef
93. B. Esteban. M. Carrascal, J. Abian, and T. Lamparter, Biochemistry, 2005, 44, 450. CrossRef
94. T. Hübschmann, H. J. M. M. Jorissen, T. Börner, W. Gärtner, and N. Tandeau de Marsac, Eur. J. Biochem., 2001, 268, 3383. CrossRef
95. K. Inomata, S. Noack, M. A. S. Hammam, H. Khawn, H. Kinoshita, Y. Murata, N. Michael, P. Scheerer, N. Krauss, and T. Lamparter, J. Biol. Chem., 2006, 281, 28162. CrossRef
96. J. R. Wagner, J. Zhang, J. S. Brunzelle, R. D. Vierstra, and K. T. Forest, J. Biol. Chem., 2007, 282, 12298. CrossRef
97. S. Seibeck, B. Borucki, H. Otto, K. Inomata, H. Khawn, H. Kinoshita, N. Michael, T. Lamparter, and M. P. Heyn, FEBS Lett., 2007, 581, 5425. CrossRef
98. X. Yang, E. A. Stojkovic, J. Kuk, and K. Moffat, Proc. Natl. Acad. Sci. U.S.A., 2007, 104, 12571. CrossRef
99. C. Dugave and L. Demange, Chem. Rev., 2003, 103, 2475. CrossRef
100. P. Scheerer, N. Michael, J. H. Park, S. Noack, C. Förster, M. A. S. Hammam, K. Inomata, H.-W. Choe, T. Lamparter, and N. Krauß, J. Struct. Biol., 2006, 153, 97. CrossRef
101. P. Scheerer, N. Michael, J. H. Park, S. Nagano, H.-W. Choe, K. Inomata, B. Borucki, N. Krauß, and T. Lamparter, ChemPhysChem, 2010, 11, 1090. CrossRef
102. L.-O. Essen, J. Mailliet, and J. Hughes, Proc. Natl. Acad. Sci. U.S.A., 2008, 105, 14709. CrossRef
103. X. Yang, J. Kuk, and K. Moffat, Proc. Natl. Acad. Sci. U.S.A., 2008, 105, 14715. CrossRef
104. X. Yang, J. Kuk, and K. Moffat, Proc. Natl. Acad. Sci. U.S.A., 2009, 106, 15639. CrossRef
105. X. Yang, Z. Ren, J. Kuk, and K. Moffat, Nature, 2011, 479, 428. CrossRef
106. R. Yang, K. Nishiyama, A. Kamiya, Y. Ukaji, K. Inomata, and T. Lamparter, Plant Cell, 2012, 24, 1936. CrossRef
107. H. Khawn, L.-Y. Chen, H. Kinoshita, and K. Inomata, Chem. Lett., 2008, 37, 198. CrossRef
108. B. Zienicke, L.-Y. Chen, H. Khawn, M. A. S. Hammam, H. Kinoshita, J. Reichert, A. S. Ulrich, K. Inomata, and T. Lamparter, J. Biol. Chem., 2011, 286, 1103. CrossRef
109. K. Inomata, H. Khawn, L.-Y. Chen, H. Kinoshita, B. Zienicke, I. Molina, and T. Lamparter, Biochemistry, 2009, 48, 2817. CrossRef
110. L.-Y. Chen, R. Iwamoto, Y. Ukaji, and K. Inomata, Chem. Lett., 2011, 40, 632. CrossRef
111. R. Iwamoto, Y. Ukaji, and K. Inomata, Chem. Lett., 2010, 39, 176. CrossRef
112. Y. Tsukahara, H. Kinoshita, K. Inomata, and H. Kotake, Bull. Chem. Soc. Jpn., 1984, 57, 3013. CrossRef
113. E. M. Franklin, S. Browne, A.-M. Horan, K. Inomata, M. A. S. Hammam, H. Kinoshita, T. Lamparter, G. Golfis, and T. J. Mantle, FEBS Journal, 2009, 276, 4405. CrossRef
114. Y. Hagiwara, M. Sugishima, H. Khawn, H. Kinoshita, K. Inomata, L. Shang, J. C. Lagarias, Y. Takahashi, and K. Fukuyama, J. Biol. Chem., 2010, 284, 1000. CrossRef
115. T. Ishizuka, A. Kamiya, H. Suzuki, R. Narikawa, T. Noguchi, T. Kohchi, K. Inomata, and M. Ikeuchi, Biochemistry, 2011, 50, 953. CrossRef