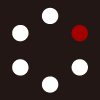
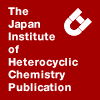
HETEROCYCLES
An International Journal for Reviews and Communications in Heterocyclic ChemistryWeb Edition ISSN: 1881-0942
Published online by The Japan Institute of Heterocyclic Chemistry
e-Journal
Full Text HTML
Received, 9th October, 2013, Accepted, 14th November, 2013, Published online, 28th November, 2013.
DOI: 10.3987/REV-13-785
■ Sixteen-Membered Macrolides: Chemical Modifications and Future Applications
Keiichi Ajito,* Tomoaki Miura, Takeshi Furuuchi, and Atsushi Tamura
Intellectual Property Department, Meiji Seika Pharma Co., Ltd., 2-4-16, Kyobashi, Chuo-ku, Tokyo 104-8002, Japan
Abstract
To produce a novel macrolide antibiotic, which is biologically stable and effective against erythromycin-resistant Streptococcus pneumoniae constitutively expressing the erm gene, we designed and synthesized a variety of novel macrolides starting from 16-membered macrolides. Initially, metabolically stable 16-membered macrolides were produced by constructing a 4-O-alkylated cladinose moiety as a stable neutral sugar. Then, an arylalkyl group was introduced to the lactone ring to improve the antibacterial activities against resistant S. pneumoniae. Although the novel analogues, which possess an arylalkyl group at the C-3 position or the western hemisphere improved antibacterial activities against inducible resistant and efflux type S. pneumoniae, they did not have sufficient activities against constitutive erm-resistant S. pneumoniae. Further, exploration of a novel macrolactone led to identification of a unique 11-azalide framework. We systematically synthesized 14- to 16-membered azalides and azalactams, and a 16-membered azalide (azalactone) was selected as a template for further medicinal chemistry. Finally, we optimized the connecting position of an arylalkyl group, structure of an arylalkyl moiety, and a neutral sugar moiety, and we synthesized a novel 15-β-substituted 16-membered 11-azalide, which was biologically stable and effective against constitutive erm-resistant S. pneumoniae.CONTENTS
Introduction
Results and Discussion
1. Studies on the Metabolism of 16-Membered Macrolides and Generation of Metabolically Stable 16-Membered Macrolides
1.1. Improvement in the Stability of EM under Acidic Conditions
1.2. Improvement of Metabolic Stability of 16-Membered Macrolides under Physiological Conditions
1.3. Design and Synthesis of Metabolically Programmed 16-Membered Macrolides
Part 1: Discovery of Cladinose Analogues in 16-Membered Macrolides
1.4. Part 2: Optimization of Cladinose Analogues in 16-Membered Macrolides
1.5. Design and Synthesis of 16-Membered Macrolides Possessing a Metabolically Stable Neutral Sugar
Part 1: Discovery of 16-Membered Macrolides Possessing a Di-O-Alkyl-Neutral Sugar
1.6. Part 2: Optimization of 16-Membered Macrolides Possessing a Di-O-Alkyl-Neutral Sugar
1.7. Chemical Transformation at the C-3 Position of 16-Membered Macrolides
2. Application of an Arylalkyl Group to 16-Membered Macrolides
2.1. Emergence of Resistant S. pneumoniae and Mechanisms of Resistance
2.2. Discovery of an Arylalkyl Group in 14-Membered Macrolides
2.3. New Aspect and Limitations of Chemical Modifications at the C-3 Position of 16-Membered Macrolides
2.4. Introduction of an Arylalkyl Moiety onto the Western Hemisphere in 16-Membered Macrolides
Part 1: Regio- and Stereoselective Epoxidation and Regioselective Ring Opening Using Sodium Azide
2.5. Part 2: Optimization of an Arylalkylamino Moiety at the C-12 Position
2.6. Part 3: An Alternative Approach, Synthesis of 13-Amino-12-Hydroxyl Analogues
3. From Natural Products to Innovative Transformation of Macrolide Framework: Synthesis of Novel Azalides
3.1. Reported Azalides and Selection of an Alternative Macrocyclization Reaction
3.2. Isolation of a Linear Dialdehyde and Its One-Step Macrocyclization
3.3. Optimization of a 15-Membered 11-Azalide and Expansion of the Scope of Azalide Research
3.4. Preparation of 14- to 16-Membered Novel Azalides and Azalactams and Template Selection
3.5. Discussion of the Position for Introduction of an Arylalkyl Moiety at the Western Hemisphere
3.6. Molecular Design of a Neutral Sugar Moiety, which is Stable under Physiological Conditions
3.7. Final Optimization of 15-β-Substituted 16-Membered 11-Azalides
Conclusions and Perspective
Acknowledgements
Reference and Notes
INTRODUCTION
In 1957, Woodward1 defined a series of glycosides with a large lactone ring as “macrolides”, and the term “macrolide” was used to denote a new class of natural products. In the field of medicinal chemistry, macrolides are typically classified as antibacterial macrolide antibiotics, antifungal polyene antibiotics, and other macrolides like avermectin, however, recently some large-ring lactones without a carbohydrate moiety are known as macrolides, for example FK-506. In this account, the term “macrolide” indicated an antibacterial macrolide antibiotic.2 Clinically important macrolides such as clarithromycin3 (CAM) and azithromycin4 (AZM) consist of derivatives possessing a 14- to 16-membered lactone (Figure 1). Starting materials of these derivatives are 14-membered erythromycin (EM) or 16-membered leucomycins (LMs) and midecamycin A1 (MDM). CAM and AZM are known as “blockbuster drugs” in the worldwide market.
Macrolides are effective against respiratory infections, and they are administered to pediatric patients because of their ease of oral administration and established safety. Macrolides exhibit strong antibacterial activities, especially against Streptococcus pneumoniae, Streptococcus pyogenes, Moraxella catarrhalis, Haemophilus influenzae, and Mycoplasma pneumoniae and thus they are clinically important therapeutic agents. The domestic sales of macrolides and quinolones in Japan, unlike those of cephalosporins, are relatively stable (Figure 25a). CAM and AZM are major macrolides, and their domestic sales are still quite high (Figure 35b), although their basic patents (original substrate patents) in Japan have expired in 2006 (CAM) and 2005 (AZM), respectively. Macrolide antibiotics inhibit protein synthesis6 by binding to closely related sites on the 50S subunit of the 70S ribosome of bacteria.
A comprehensive study of 14-membered and 16-membered macrolides by Kirst and Sides7 showed that the 14-membered macrolides have stronger antibacterial activities and higher plasma concentration than the 16-membered macrolides. On the other hand, the 16-membered macrolides are superior to 14-membered macrolides because of lower incidence of drug interactions, lesser gastrointestinal stimulation, and acceptable bitter taste. Moreover, natural 16-membered macrolides8 and chemically modified 16-membered macrolides such as rokitamycin9 (RKM) and miokamycin10 (MOM) cannot become a substrate of the efflux pump by an mef gene, and thus they show remarkable antibacterial activities against a large variety of resistant bacteria, which possess the mef gene. Because of the advantages mentioned above, we used the 16-membered macrolides as our research target. The naturally occurring 14- and 16-membered macrolides are not always stable. Chemical instability under acidic conditions and metabolic instability under physiological conditions are the issues that need to be addressed in the 14- and 16-membered macrolides, respectively (cf. sections 1.1. and 1.2).
Until the 1980s, improvement in the stability of macrolide antibiotics was an important issue, however, EM resistant S. pneumoniae (ERSP) with an erm gene that emerged in the 1990s caused clinical problems because this type of ERSP could not be inhibited by CAM, AZM, or 16-membered macrolides. We focused on improvement in the stability of 16-membered macrolides and found a practical solution at an early stage. Then, we tried to design and synthesize a novel 16-membered macrolide, which is effective against resistant S. pneumoniae with an erm gene, by using different chemical approaches. Finally, we synthesized a novel and metabolically stable 16-membered 11-azalide, which was effective against resistant S. pneumoniae and resistant S. pyogenes with an erm gene. In this account, we describe (i) the regulation of metabolism of the 16-membered macrolides, (ii) several approaches to synthesize 16-membered macrolide derivatives, which are active against resistant S. pneumoniae, (iii) discovery of 16-membered 11-azalide framework, and (iv) its final optimization by accurate medicinal chemistry.
A carbohydrate moiety cannot be easily introduced during the synthesis of antibacterial macrolides similar to that observed in the total synthesis of macrolides. For example, Tatsuta et al.11 completed and reported introduction of a carbohydrate moiety to a protected lactone ring before the construction of an aglycone framework in the total synthesis of carbomycin B or oleandomycin. These findings indicate that efficient introduction of a carbohydrate moiety to a macrolactone is extremely difficult. Although initially we used glycosylation reactions to determine concept molecules (sections 1.3. and 1.5.), subsequently, we synthesized 16-membered macrolides without glycosylation reaction and focused on future development of process chemistry.
RESULTS AND DISCUSSION
1. Studies on the Metabolism of 16-Membered Macrolides and Generation of Metabolically Stable
16-Membered Macrolides12
1.1. Improvement in the Stability of EM under Acidic Conditions
Although EM has strong antibacterial activities, the spiroketal (Figure 4) formed through formation of an enol ether13 between a hydroxyl group at the C-6 position and a carbonyl group at the C-9 position under acidic conditions and subsequent attack of a tertiary hydroxyl group at the C-12 position at the C-9 position does not have marked antibacterial activities. Morimoto et al.3 performed regioselective 6-O-methylation to avoid intramolecular ketal formation and generated CAM (Figure 1) with significantly improved pharmacokinetics. The clinical efficacy of CAM is enhanced by (i) strong activity of a major metabolite against H. influenzae and (ii) a variety of non-antibacterial activities,14 for example, immunomodulatory properties. D’Ambrieres et al.15 converted a carbonyl group at the C-9 position of EM to an alkyloxy-imino ether to synthesize roxithromycin (RXM) (Figure 1), which showed improved pharmacokinetics. Djokić et al.4 transformed a 14-membered lactone of EM to a 15-membered azalactone using Beckmann rearrangement and finally synthesized 15-membered azalide, AZM (Figure 1) with a nitrogen atom-containing lactone ring. AZM is more stable and shows stronger antibacterial activity than EM against Gram-negative bacteria, including H. influenzae. Although CAM required twice a day administration, a long plasma half-life enabled once a day administration of AZM.
1.2. Improvement of Metabolic Stability of 16-Membered Macrolides under Physiological Conditions
Typically, the C-3” position of the neutral sugar moiety in natural 16-membered macrolides is a free hydroxyl group and the C-4” position is an acyloxy group, except spiramycin (SPM) (Figure 1). The acyl groups at the C-4” position are mobile under physiological conditions, and natural 16-membered macrolides are metabolized to their 3”, 4”-diol analogues with markedly low antibacterial activities. Ōmura et al.9 introduced a propionyl group to the C-3” position of leucomycin A5 (LM-A5) to generate RKM (Figure 1), and Omoto et al.10 introduced two acetyl groups to the C-9 and C-3” positions of MDM to generate MOM (Figure 1). One of the major metabolic pathways of MOM in humans is hydrolysis of the propionyl group at the C-4” position16 and simultaneous intramolecular migration of the 3”-acetyl group to the C-4” position to afford a metabolite Mb-1 (Figure 4), which shows acceptable antibacterial activities. In addition, the major metabolic pathways of RKM17 include in vivo deacylation at the neutral sugar moiety. Compared to their parent natural products, RKM and MOM showed improved pharmacokinetics, and their antibacterial activities against inducible methylase-type resistant S. pneumoniae with an erm gene (inducible resistant S. pneumoniae) increased by introduction of an acyl group to the C-3” position. Moreover, these two derivatives showed stronger antibacterial activity than CAM or AZM against resistant S. pneumoniae with an mef gene (efflux pump type) (minimum inhibitory concentrations (MIC, µg/mL) of RKM, MOM, CAM, and AZM are 0.13. 0.25, 1, and 1, respectively). On the other hand, introduction of an acetyl group to the 9-hydroxyl group in MOM increased hydrophobicity of the entire molecule, and the bitter taste peculiar to macrolides could be partially masked. The modified MOM continued to show efficacy as an oral antibiotic agent, particularly in the case of pediatric patients. Under physiological conditions, the acetyl group at the C-4” position of Mb-1 is removed to form Mb-2 (Figure 4). Thus, even these molecules, which possess 2 acyl groups at the C-3” and C-4” positions, did not show significant metabolic stability18 in clinical conditions. Improvement in pharmacokinetics may partially contribute to the desired properties of macrolide antibiotics proposed by Kirst.19
1.3. Design and Synthesis of Metabolically Programmed 16-Membered Macrolides
Part 1: Discovery of Cladinose Analogues in 16-Membered Macrolides
Meiji Seika Kaisha, Ltd., (now, Meiji Seika Pharma Co., Ltd.) has discontinued the studies on macrolides since the development of MOM further, it was very difficult to catch up with current studies on macrolides performed by other major research groups such as the Kitasato Institute, Taisho Pharmaceutical, Abbott, and Roussel (Hoechst Marion Roussel). Our group consists of not only general chemists but a carbohydrate chemist and a fermentation professional. Thus, we focused on glycosylation and biotransformation (bioconversion) as novel approaches for developing 16-membered macrolides. To date, the major chemical modifications in 16-membered macrolides include simple acylations because the 16-membered macrolides have many chemically sensitive moieties (cf. Figure 34). The findings from studies on synthetic chemistry, biotransformation, and metabolism enable the development of metabolically stable 16-membered macrolides (section 1.6.).
In 1977, Tatsuta et al.20 reported synthesis of a cladinose analogue of carbomycin B, compound (1) (Scheme 1), as a pioneer work in the application of “glycal chemistry” and showed that 1 had stronger antibacterial activity than carbomycin B against Mycobacterium smegmatis, but its activity was comparable to that of carbomycin B against other bacteria. Although 1 was the first example of a cladinose analogue of a 16-membered macrolide, no information was available about its structure-activity relationship (SAR) or metabolism. Thus, we hypothesized that compound 1 with “an acyl group at the C-4” is more stable under physiological conditions than the natural 16-membered macrolides, including carbomycin B, because of steric hindrance of the 3”-methoxy group” and “if an acyl group at the C-4” position was removed, a cladinose-type analogue (compound 2 or 3 in Scheme 1) might be still more potent than the diol-type metabolite (metabolite Mb-2 or Mb-6 in Figure 4)” because the 14-membered macrolides, which possess a cladinose-type neutral sugar, showed strong antibacterial activities.
We studied cladinose analogues 2 and 3 as our target molecules. Because direct introduction of a methyl group into the 3”-hydroxyl group was not easy, we used a glycosylation method. We used 1-(2-pyridylthio) sugar for glycosylation21 similar to that used by Woodward et al.22 for successful asymmetric total synthesis of EM. A cladinose moiety was regioselectively introduced into the C-4’ position of 6 via glycosylation in the presence of anhydrous silver perchlorate and pulverized molecular sieves in dry MeCN to afford the desired α-glycoside (5) together with a β-anomer. High α-stereoselectivity could not be achieved because of the lack of neighboring group effects in this 2-deoxy donor (7). Few studies have reported high stereoselectivity in the preparation of 2-deoxyglycosides, except in the case of some strategies.23 According to the 3”-methoxy group supposedly, a propionyl group at the C-4” position of 4 was stable as we expected, and our first hypothesis was qualitatively confirmed as a biotransformation level. Common microorganisms such as Mucor spinescens IAM 6071 are unable to cleave the 4”-O-propionyl group of compound 4; this phenomenon is considered attributable to steric hindrance by the 3”-methoxy group. If steric hindrance is indeed the case of this phenomenon, then our first hypothesis would be valid. However, since this phenomenon was observed only during biotransformation, the validation would be conditional, not absolute. Therefore, we performed the desired deacylation using Paecilomyces sp. PF1108 as a special case to obtain a cladinose analogue (3). Finally, stereoselective reduction at the C-9 position of 324 smoothly proceeded by biotransformation using Streptomyces mycarofaciens SF277225 to afford compound 2. Our previous studies26 on macrolides showed that the 16-membered macrolide derivatives, which possess an sp3 carbon at the C-9 position, showed better pharmacokinetics than those possessing an sp2 carbon. Another study showed27 that chemical reduction of a carbonyl group at the C-9 position of antibiotics in the leucomycin family resulted in a mixture of diastereoisomers, α- and β-alcohol. Various other methods for reducing the C-9 carbonyl group of 16-membered macrolides to the corresponding alcohol using different synthetic28 and biochemical29 approaches have been reported.
The cladinose analogues 2 and 3, especially the 9-dehydro derivative (3), showed very strong antibacterial activities. The antibacterial activities of cladinose analogues 2 and 3 against clinically important pathogens in respiratory infections were around 8 times stronger (precisely 4 to 32 times stronger) than those of the corresponding diol analogues, Mb-630 (Figure 4) and DOP31 (Scheme 1, Table 132). This finding prompted us to exhaustively explore cladinose analogues of 16-membered macrolides. We hypothesized that “(i) an acyl group at the C-4” position is rather stable under physiological conditions and (ii) if the acyl group is removed by metabolism, the cladinose-type metabolite exhibits stronger antibacterial activities than the diol-type metabolite”.
Because we obtained attractive and novel cladinose analogues using glycosylation and biotransformation, we decided to explore an alternative synthetic route for extensive preparation of cladinose analogues, which could also be used to scale-up production. We had
some difficulties in direct methylation of a hydroxyl group at the C-3” position in the presence of a 4”-O-acyl group, an intact aldehyde, and an unmodified lactone ring; thus, we selected an indirect method to introduce a methyl group into a tertiary hydroxyl group at the C-3” position (Scheme 2). We used a methylthiomethyl (MTM) ether as a key intermediate to generate a 3”-OMe group. A 3”-MTM ether of MDM has already been reported33 as a useful semisynthetic analogue of MDM. A study performed in the late 1960s showed that an MTM ether converted into a methyl ether via heterogeneous hydrogenolysis.34 We selected an ethoxyethyl group as a protecting group at the C-9 position to isolate a 9-hydroxyl analogue (13), because the 9-O-acetyl group as a protecting group was not appropriately cleaved. A key compound 11, however, possessed chemically sensitive functional groups, a diene and an aldehyde, which were labile under hydrogenolysis conditions. As we expected, the standard protocol of hydrogenolysis of 11 easily afforded an undesired perhydrogenated compound with a 3”-OMe analogue. However, well-controlled deactivated Raney nickel under optimized conditions converted 11 to the desired 3”-methyl ether (12) in a moderate yield.35
Before detailed evaluation of the selected cladinose analogue(s), we performed SAR studies. We used MDM and josamycin (JM) (LM-A3) to obtain the SAR information at the C-3, C-9, and C-4” positions. The cladinose-type MDM derivatives (3-O-COEt and 4”-O-COEt) and the corresponding JM derivatives (3-O-Ac and 4”-O-COiBu) showed almost similar antibacterial activities. Antibacterial activities of 9-O-COEt derivatives were weaker than those of the corresponding 9-O-Ac derivatives (data not shown). The in vitro activity of 13 was slightly stronger than that of 14 (Table 2), but we selected a 9-O-Ac-analogue (14) for further studies because we expected it to have better pharmacokinetics and tolerable bitter taste (see the last part of section 1.2). Compound 14 was efficiently prepared from 15 in 3 steps (Scheme 2).
Serum concentrations of 14 and MOM after oral administration in mice are shown in Figure 5.35 The serum level of compound 14 was higher than that of MOM. Analysis of urinary excretion of the compounds by a bioassay method using Micrococcus luteus ATCC9341 showed that the urinary excretion of 14 was larger than that of MOM or MDM (Figure 6).35 These findings may be attributed to the strong antibacterial activities of cladinose-type metabolite(s). Then, we evaluated the in vivo potency of 14 using a mouse model of systemic infection. The median effective dose (ED50) values showed that the in vivo activity of 14 against Staphylococcus aureus Smith I was 4 to 5 times greater than that of MOM. In addition, compound 14 was about 4 times more potent than MOM against S. pneumoniae DP-I Type I.35 The MIC values of 14 were the same as those of MOM i.e. 0.20 μg/mL; therefore, the relatively strong in vivo efficacy of 14 deserved special mention, and we concluded that the antibacterial activities of some of major metabolites (for example, 2 vs. Mb-6) contributed to the strong in vivo efficacy. Strong in vivo efficacy at the clinical site is very important for suppressing the emergence of resistant bacteria. Then, we tried to develop novel cladinose analogues, which showed enhanced antibacterial activities.
1.4. Design and Synthesis of Metabolically Programmed 16-Membered Macrolides
Part 2: Optimization of Cladinose Analogues in 16-Membered Macrolides
The in vitro antibacterial activities of RKM are stronger than those of MOM. The C-3 position of RKM is a free hydroxyl group, and that of MOM is a propionyloxy group. The SAR studies of 16-membered macrolides showed that the in vitro potency of a molecule with a free hydroxyl group at the C-3 position is stronger than that with an acyloxy group at the C-3 position. Thus, we prepared 3-OH cladinose analogues of 16-membered macrolides to evaluate their biological activities. We planned to use the biotransformation method to prepare the 3-OH cladinose analogues because many kinds of efficient biotransformation methods36 have been used thus far to synthesize macrolide antibiotics.
In the leucomycin family of macrolides, an acyl group at the C-3 position could not be efficiently cleaved by chemical reactions under acidic or basic conditions (The acetyl group at the C-3 position in the tylosin family can be chemically cleaved under acidic conditions). Under acidic conditions, a neutral sugar moiety and an allylic alcohol at the C-9 position are very labile, and two ester bonds in the lactone ring and at the C-4” position are quite unstable under basic conditions. Okamoto et al.37 screened many types of microorganisms and found that Bacillus subtilis ATCC 14593 could convert JM to leucomycin A1 (LM-A1) (Figure 7). Although this practical 3-de-O-acylation in the leucomycin family of macrolides was reported for the first time, the reported conversion yield was 7.0%. Moreover, we had to remove a propionyl group (not an acetyl group); thus, we decided to screen novel microorganisms with powerful and regioselective 3-de-O-acylation potency.
We did not focus on bacteria or Actinomycetes species considering the antibacterial spectra of macrolide antibiotics. Shimizu et al.38 screened more than 250 strains of fungi and discovered two microorganisms, Phialophora sp. PF1083 and Preussia sp. PF1086, which could convert MDM to leucomycin A7 (LM-A7) (Figure 7). Under optimized fermentation and purification conditions, the PF1083 strain could selectively remove the 3-O-propionyl group of MDM to consistently afford LM-A7 in a yield of 30% or more. Finally, compound 13 was converted to compound 18 in a yield of 28% using the PF1083 strain. Substrate specificity studies39 showed that an enzymatic reaction using the PF1083 strain selectively recognized the substrate with a 4”-O-acylated neutral sugar moiety (cf. section 1.6).
Compared to compound 13, compound 18 showed markedly enhanced antibacterial activities (4 to 16 times stronger). Compound 18 was 4 times stronger than LM-A7 (Table 3) against S. pneumoniae, and it was confirmed that a 4”-O-acylated cladinose-type neutral sugar was important for increasing the activities of these compounds against target pathogens. Moreover, the antibacterial activities of a tentative major metabolite of 18, compound 19, were remarkably stronger (4 to 8 times stronger) against clinically important pathogens in respiratory infections than those of a diol-type metabolite, leucomycin V40 (LM-V) (Figure 7). Subsequently, we optimized 3-OH cladinose analogues in the 16-membered macrolides because 18 and its 4”-O-acyl analogues were supposed to exhibit stronger in vivo activities than the existing 16-membered macrolides, according to the above mentioned stronger antibacterial activities of both an intact molecule (18) and its tentative major metabolite.
To optimize an acyl group at the C-4” position, we had to prepare a key intermediate with a free hydroxyl group at the C-4” position. Moreover, two hydroxyl groups at the C-9 and the C-2’ positions and an aldehyde group had to be protected in the key intermediate. Although the hydroxyl groups at the C-3 and C-3” positions are less reactive, the C-3 hydroxyl group and the aldehyde group easily form a very reactive hemiacetal. Kurihara et al.41 addressed this issue and synthesized a key intermediate, a diol (22), by using a combination of previously reported methods (Scheme 3).
2’-O-Acetylleucomycin A7 (20) was converted to bis-TBS intermediate by using the Kitasato method reported by Sano et al.,42 which afforded the desired diol (22) by heterogeneous basic hydrolysis. Despite the highly basic conditions, the lactone ring was stabilized by a fused 7-membered silyl hemiacetal, and the 2’-O-acetyl group could be retained. The exceptional stability of the 2’-O-acetyl group under those phase transfer conditions43 is worth mentioning. Yields of regioselective acylation at the C-4” were more than 90%, because the tertiary alcohol at the C-3” position was originally less reactive. Conversions from compounds 23a-23d to 26a-26d were performed on the basis of our previous experience (section 1.3. Scheme 2). The hydroxyl group at the C-3” position, however, was less active than the general tertiary hydroxyl group because of steric hindrance44 by the TBS group at the C-18 position. Practical methylthiomethylation of 23 was finally accomplished by addition of benzoic anhydride45 to afford 24 in a moderate yield. The final deprotection of the TBS groups of 26a-26d by using tetrabutylammonium fluoride (TBAF) afforded desired 3-OH cladinose analogues (27a-27d).
Antibacterial activities of compounds 27a-27d against target pathogens are shown in Table 4. All five analogues showed very strong antibacterial activities, and the antibacterial activities of compounds 27b and 27c against S. pneumoniae were 2 to 4 times stronger than those of RKM. To our knowledge, these antibacterial activities are most potent among macrolides belonging to the leucomycin family. On the other hand, we could synthesize compound 27a in only 6 steps using LM-A5 (Figure 1) as the starting material, and we prepared the 9-O-acetyl derivative and 9-O-propionyl derivative of 27a. The in vivo activities of 27a and its 9-O-acyl derivatives against S. pneumoniae DP-I Type I,41 however, were only two times potent than that of RKM.
Thus, we concluded that optimized 3-O-COEt cladinose-type 16-membered macrolide (14) and 3-OH cladinose-type 16-membered macrolides (27) and its 9-O-acyl derivatives showed characteristic pharmacokinetics and stronger in vitro and in vivo antibacterial activities against target pathogens than the corresponding existing 16-membered macrolides, MOM or RKM, respectively. These novel analogues, however, were still relatively unstable under physiological conditions, and the in vivo efficacy of these analogues was not as high as that of CAM in mice model. Then, we decided to synthesize a 16-membered macrolide with high metabolic stability, that is, a 16-membered macrolide in which the neutral sugar moiety is not metabolized at all.
1.5. Design and Synthesis of 16-Membered Macrolides Possessing a Metabolically Stable Neutral Sugar
Part 1: Discovery of 16-Membered Macrolides Possessing a Di-O-Alkyl-Neutral Sugar
Since we could generate only one candidate (14) using the cladinose-type 16-membered macrolide, we used another strategy to develop a new chemical class of 16-membered macrolides, which possess a di-O-alky-neutral sugar. Previous studies have reported the synthesis of 4”-O-alkyl 16-membered macrolides (Figure 8). Sano et al.42 reported the synthesis and biological evaluation of 4”-O-alkylspiramycin I analogues (28). The original study described that “introduction of alkyl groups, which are poorly hydrolyzed to the corresponding 4”-hydroxyl group would be an interesting approach to further chemical modifications.” All the novel derivatives of 28, except 28a, showed in vitro antibacterial activities similar to or stronger than those of the parent spiramycin I (SPM I); however, the in vivo potency of 28b as a selected compound was not improved. The 3, 3”-di-O-acetyl derivatives of 28a and 28b were then synthesized, and the derivative of 28a showed enhanced antibacterial activity in vivo.46
Kiyoshima et al.47 reported synthesis of 4”-O-benzyltylosin analogues (29) using regioselective alkylation with the dibutyltin oxide method.48 Compared to the control (30), these compounds (29a-29d) showed improved in vitro stability, and their in vitro antibacterial activities were similar to those of 30. Synthesis of the above tylosin analogues did not seem to be quite difficult because of the (i) 9-dehydro structure (no allylic rearrangement) and (ii) reactive O-benzylation. On the other hand, synthesis of compounds 28b and 28c seemed to be rather difficult because of (i) sp3 carbon at the C-9 position (possibility of allylic rearrangement) and (ii) less reactive aliphatic alkylation, but a fused 7-membered silyl hemiacetal enable aliphatic alkylation at the C-4” position. Initially, we wanted to synthesize 3-O-COEt analogues (no fused 7-membered silyl hemiacetal was available); therefore, we used the previously established glycosylation approach.
Glycosylation of compound 6 with 1-(2-pyridylthio) sugars (33a-33i) proceeded in the presence of anhydrous silver perchlorate followed by reduction of a tertiary amine oxide to afford the desired 9-dehydro-di-O-alkyl analogues (31a-31i)49 (Scheme 4). Regioselectivity between the C-2’ and C-4’ positions was achieved (i.e. 2’-O-glycosylation did not occur), but stereochemistry at the C-1” position could not be controlled (the desired α-anomer, 38%; the undesired β-anomer, 38% based on consumption of compound 6, respectively). Although a variety of 4-O-alkyl groups of 33 were accepted under these glycosylation conditions, only 33e, which possesses a prenyl group resulted in a poor glycosylation yield (9.5%) because of its instability under acidic conditions.
All 9-dehydro-di-O-alkyl analogues (31a-31i) showed clear antibacterial activities, but the compounds 31a (ethyl), 31b (allyl), and 31h (methoxyethoxyethyl) showed rather weak potency (data not shown). Not only the length of the 4”-side chain but also its hydrophobicity affected the antibacterial activity. The antibacterial activities of other compounds were comparable to those of the parent compound midecamycin A3 (MDM-A3) (Scheme 4, Table 5). We selected compound 31d for further evaluation as a prototype molecule, because a carbon framework of the 4”-sidechain of 31d was the same as that of JM or carbomycin B.
Ishizuka49 soon proved sustainable antibacterial activity of 31d in rat plasma compared to the corresponding natural antibiotics, carbomycin B and MDM-A3 as shown in Figure 9. Further, compared to the corresponding natural antibiotics, compound 31d showed markedly improved pharmacokinetics, i.e., serum concentration and urinary recovery (Figure 10) in in vivo mouse models.49 The di-O-alkyl analogues, especially the 9-dehydro molecule (31d), showed sustainable antibacterial activities in the plasma, and their pharmacokinetics were superior to those of the existing macrolides; therefore, we decided to expand this research program and planned (i) stereoselective reduction at the C-9 ketone of 31d and its analogues for improvement of pharmacokinetics and (ii) regioselective hydrolysis of the propionyl group at the C-3 position for enhancement of antibacterial activities in application of biotransformation.
1.6. Design and Synthesis of 16-Membered Macrolides Possessing a Metabolically Stable Neutral Sugar
Part 2: Optimization of 16-Membered Macrolides Possessing a Di-O-Alkyl-Neutral Sugar
A carbonyl group at the C-9 position of compounds 31 was converted to the corresponding α-hydroxyl group in a moderate yield by biotransformation using SF277225 (Scheme 5). The antibacterial activities of compound 34d and some of its analogues were comparable and their stability in rat plasma was significantly improved compared to that of MDM50 (data not shown).
Serum concentration of 34d detected by microbial assay was extremely higher, and 34d remained in circulation for a longer time than MDM, and its AUC was also larger than that of MDM. In particular, the markedly high maximum plasma concentration (Cmax) level of 34d as the 16-membered macrolide antibiotic was comparable to that of CAM (Figure 11).50 To date, only few analogues belonging to the leucomycin family have been reported to have such high serum concentrations. Moreover, the urinary recovery of 34d within 24 hours in mice was 20% (Figure 12), while it was less than 1% for MDM, and 24% for CAM. Increased serum concentration and delayed excretion as well as high urinary recovery actually contributed to strong in vivo potency, especially against S. pneumoniae (cf. the last part of section 1.6).
Although we tried biotransformation of compound 34d using Phialophora sp. PF1083 for the purpose of regioselective 3-de-O-propionylation, the conversion yield was less than 10% (Scheme 5). The yield of 35i was also less than 10%, and we supposed that a low yield of this biotransformation is substrate specific, more precisely, 4”-O-substituent specific. Compounds 35d and 35i exhibited enhanced antibacterial activities against clinically important Gram-positive pathogens compared to those of LM-A7,51 and the half-life of 35d in rat plasma was 3 to 4 times longer than that of LM-A7 or RKM. Consideration 28% yield of compound 18 plus 16% recovery of starting material (13) using PF1083 (Figure 7), we were interested in the substrate specificity of PF1083. The studies performed by Shimizu39 indicated that an efficient 3-de-O-propionylation of 16-membered macrolides using Phialophora sp. PF1083 required a 4”-acylated neutral sugar moiety. Even preliminarily optimized overall yield of compound 35d from an intermediate (6) was almost 1%, and we had to develop a novel synthetic route of 35d and its analogues in order to (i) prepare medium size of compound 35d for in vivo studies using not only small rodent animals but large rodent animals also and (ii) optimize the 4”-O-alkyl group.
Kurihara et al.52 used LM-A7 as a starting material and utilized Kitasato protection (silyl hemiacetal). Although the 2’-O-acetyl group was acceptable for 4”-O-acylation (Scheme 3), 4”-O-alkylation and direct 3”-O-methylation required a very stable 2’-O-protecting group, i.e. 2’-O-TBS group. In addition, direct 3”-O-methylation required protection of a dimethylamino group at the C-3’ position. We synthesized completely protected desired 3”,4”-di-O-alkyl analogues (40) (Scheme 6). According to the original information,53 it was reported that a 2’-O-TMS protecting group could be hardly removed in 14-membered macrolide intermediates. Thus, a 2’-O-TBS group (a free dimethylamino group at the C-3’ position) could not be removed even in different conditions, but the 2’-O-TBS group of compounds 40 was simultaneously removed when we reduced the dimethylamino N-oxide of 40s to the free dimethylamino group. Although this mechanism could not be clarified yet, Professor Shuto54 provided us with a tentative mechanism. Optimized overall yield of 35d from LM-A7 was around 20%, and we successfully completed scale up synthesis of 35d and optimization of the 4”-O-alkyl group of 35d.
Antibacterial activities of 16-membered macrolides, which possess a 3”,4”-di-O-alkyl neutral sugar are shown in Table 6. Regarding to optimization of the 4”-O-alkyl group, an n-butyl group (35c), a 3-methylbutyl group (35d), and a benzyl group (35i) were selected according to the activities against clinically important Gram-positive pathogens, and compound 35d was finally selected by the activities against clinically important Gram-negative pathogens. Antibacterial activities of 35d against Gram-positive strains are comparable to those of RKM. Antibacterial activities of 3”-O-ethyl analogue and 3”-O-n-propyl analogue of 35d were decreased compared to those of 35d, and acidic stability of 3”-OH analogue of 35d was extremely poor because of lack of 1,3-diaxial steric hindrance by the 3”-methoxy group. Then, we examined characteristic pharmacokinetics and in vivo efficacy of 35d.
We performed preliminary pharmacokinetic analysis of 35d. We orally administered 200 mg/kg of 35d, RKM, and CAM to mice. Time course of serum concentrations of these 3 molecules is shown in Figure 13. Absolute serum concentrations of 35d detected by microbial assay were dramatically higher than those of RKM.52 The serum level of LM-A7 was lesser than that of RKM (data not shown). In particular, the markedly high Cmax level of 35d as the 3-OH-type 16-membered macrolide antibiotic was comparable to that of CAM. These results led us to perform further pharmacokinetic study of 35d using hamsters as larger test animals. In the field of 16-membered macrolides, pharmacokinetics are often strongly affected by animal species.9 We orally administered 500 mg/kg of these three molecules to hamsters. Compound 35d exhibited a dramatically improved serum level detected by microbial assay than RKM (Figure 14). Moreover, the Cmax levels of 35d at 30 minutes, 1 hour, and 2 hours after administration were quite higher than those of CAM. In conclusion, we discovered the novel 16-membered macrolide antibiotic (35d) with dramatically improved pharmacokinetics and strong in vitro potency.
Finally, we evaluated in vivo potency of 2 selected macrolides (34d and 35d) by protective effect against systemic infections in mice, compared them with a 3-O-propionyl analogue, MDM for 34d, and a 3-OH-type analogue, RKM for 35d. The ED50 values indicated that the in vivo efficacy of 34d and 35d against both S. pneumoniae DP-I type I (n = 8) and S. aureus Smith I (n = 5) was superior to that of MDM and RKM, respectively. Further, the in vivo potency of 34d against S. pneumoniae DP-I type I was 16 times stronger than that of MDM,55,56 and 35d was 4 to 8 times (6.5 times according to calculations) more active in vivo than RKM against the same pathogen. In conclusion, these novel 16-membered macrolide antibiotics (34d and 35d) with a di-O-alkyl neutral sugar moiety exhibited enhanced in vivo efficacy and dramatically improved pharmacokinetics in mice and hamsters.
1.7. Chemical Transformation at the C-3 Position of 16-Membered Macrolides
Compared to 14-membered macrolides, 16-membered macrolides have a relatively unstable lactone linkage.57 Steric hindrance around an ester bond in a 16-membered macrolactone (Figure 1, two broken circle lines) is relatively smaller than that in 14-membered macrolactone. Although a lactone linkage in 3-O-acyl-type 16-membered macrolides is relatively stable, a lactone linkage in the 3-OH-type 16-membered macrolides is relatively unstable. On the other hand, the in vitro potency of 3-OH-type 16-membered macrolides is stronger than that of 3-O-acyl-type analogues as described in the first part of section 1.4. We determined one approach for resolving this paradox at the C-3 position, where the in vitro potency and stabilization of the lactone linkage are inversely related.
We focused on the fact that in a three-dimensional structure, the hydroxyl group at the C-3 position was close to the aldehyde at the C-18 position and planned to synthesize 3-epi derivatives of 16-membered macrolides. In addition, the 3-epi-derivatives did not form a 3,18-hemiacetal because of the difference in the distance between the 3-epi-OH and the 18-aldehyde group in these derivatives and that between the 3-OH and 18-aldehyde group in the natural compounds. An important intermediate (44) was reacted with dimethyl sulfoxide, trifluoroacetic anhydride, and triethylamine to afford a ketone (45) in 30% yield (Scheme 7);58 however, oxidation of 44 using alternative oxidation agents such as pyridinium dichromate (PDC) or other mild oxidants (i.e., Dess-Martin periodinane or tetra-n-propylammonium perruthenate-N-methylmorpholine N-oxide [TPAP-NMO]) was not successful. Treatment of 45 with sodium borohydride followed by deprotections yielded the desired β-alcohol, 3-epi-LM-A7 (48). Remarkable β-selectivity can be explained by the following reasons: (i) the C-3 carbonyl group located at the β-site of the lactone and (ii) reagents generally attack a macrolactone from the outside of the lactone ring in macrolide chemistry. We prepared a 3-O-COEt congener, 3-epi-MDM (51), using 46.
The antibacterial activities of 3-epi-analogues (48 and 51) against clinically important pathogens in the respiratory infections were similar to or lesser than those of the corresponding natural products, LM-A7 and MDM (Table 7). The biological stability of 48 in rat plasma was significantly lower than that of LM-A7 even in the preliminary study. Half-life of 48 was approximately 8 times shorter than that of LM-A7. Thus, we developed an alternative approach for improvement of the biological stability of a lactone.
We simultaneously focused on the two aspects for a side chain at the C-3 position as follows: (i) a small volume for in vitro potency and (ii) an electron donating character for increasing the basic stability of the lactone linkage. We used compound 44 as a key intermediate, and the corresponding RKM intermediate (52b) was prepared from RKM in 3 steps using procedures similar to those used for preparing compound 44. An alternative important intermediate (52c) was synthesized from 41d (Scheme 6) in 3 steps, including (i) regioselective deprotection of the TBS group at the C-18 position, (ii) 18-dimethylacetal formation, and (iii) regioselective 2’-O-acetylation. Regioselective 3-O-methylation of these intermediates (44, 52b, 52c) using methyl iodide with a strong base in dimethyl sulfoxide followed by deprotections afforded the desired 3-O-methyl analogues (55a, 55b, 55c) (Scheme 8).
The antibacterial activities of these 3-O-methyl analogues (55a, 55b, 55c)58 against S. pneumoniae were similar to or slightly stronger than those of the corresponding 3-OH analogues, LM-A7, RKM, and the promising derivative (35d), respectively (Table 8). Moreover, compounds 55b and 55c showed improved biological stability in rat plasma. After incubation for 2 hours at 37 °C, 55b and 55c were 2 to 3 times more stable than the corresponding 3-OH analogues, RKM, and 35d. In conclusion, compound 55c was proposed to be one of the most biologically stable 16-membered macrolide (6 to 8 times more stable than RKM) with antibacterial activities as strong as RKM. On the basis of these results, we concluded that methylation of the 3-OH group and 3”, 4”-di-O-alkylation are one of the most promising modifications for improvement of the biological stability of the 16-membered macrolides.
Among these characteristic molecules, compound 14 was assigned as a candidate for in-house development, and compound 35d was discussed as a candidate in collaboration with a well-known pharmaceutical company in Japan.
2. Application of an Arylalkyl Group to 16-Membered Macrolides
2.1. Emergence of Resistant S. pneumoniae and Mechanisms of Resistance
Severe problems due to dangerous pathogens59 at clinical site are important topics of consideration even today. In the 1990s, emergence of a resistant strain of clinically important pathogens in respiratory infections was an important cause of concern, especially in the pediatric field. Among them, a predominant type (with an erm gene) of ERSP could not be inhibited using CAM, AZM, or 16-membered macrolides. On the other hand, emergence of EM resistant S. pyogenes60 was reported in 1991, and resistance to EM in S. pyogenes has become widespread.61 Isolation frequency of ERSP in Japan has gradually increased62 and reached 80% (Figure 15). High frequency of resistant S. pneumoniae is common in Japan, and this frequency is not so high in European countries or in the United States. The global clinical trial, Prospective Resistant Organism Tracking and Epidemiology for Ketolide Telithromycin (PROTEKT),63 indicated that the frequency of ERSP was 77.9% in Japan, 30.9% in the US, and 24.4% in European countries. Another trial, Community-Acquired Pneumonia (CAP),64 indicated that the frequency of ERSP was 81.4% in Japan.
Macrolide resistance in S. pneumoniae occurs mainly by two mechanisms, target-site modification (an erm gene) or efflux of the drug out of the cell (an mef gene). The most common form of target-site modification65 includes dimethylation of a specific adenine residue on the 23S rRNA (A2058 in Escherichia coli numbering) by an rRNA methylase. Because macrolide antibiotics exert antibacterial activities by interaction of a ribosomal subunit,66 dimethylation by an erm gene of the adenine residue on the rRNA is recognized as a mechanism of resistance. Kadota67 reported that the frequency of both types of resistant S. pneumoniae (with an erm gene or an mef gene) was increasing in Japan (Figure 16), and Farrell et al.68 concluded that resistant S. pneumoniae possessing both genes (an erm gene and an mef gene) markedly increased in only three years in the US (Figure 17). The distribution of macrolide resistance of S. pneumoniae isolates (erm-type vs. mef-type) differed depending on the country (Figure 18).65 For example, development of resistance by target-site modification by methylase (an erm gene) was observed in France and Spain, and efflux of the drug (an mef gene) was the more common mechanism of development of resistance in the US. A worldwide review indicated that the frequency of target-site modification by methylase was more than 50%, thus, we focused on resistant S. pneumoniae possessing methylase (an erm gene) as a primary target. In addition, we decided to investigate the antibacterial activities of 16-membered macrolides against other clinically important Gram-positive pathogens in respiratory infections, such as S. pyogenes, which possesses an erm gene. Our study using 16-membered macrolides was advantageous because intact or chemically modified 16-membered macrolides8 were effective against resistant S. pneumoniae possessing an mef gene. Therefore, they could not become a substrate for an efflux pump.
2.2. Discovery of an Arylalkyl Group in 14-Membered Macrolides
In the early 1990s, major groups performing studies on macrolides began synthesis of novel macrolide derivatives with a focus on antibacterial activities against resistant bacteria. Kashimura et al.69 reported the synthesis and antibacterial activities of TE-802 at the 35th Interscience Conference on Antimicrobial Agents and Chemotherapy (ICAAC), which was held in San Francisco in 1995. The chemical structure of TE-802 included a fused tricyclic aglycone consisting of a diazaheptane ring and oxazolidinone accompanied with a 14-membered lactone (Figure 19). Agouridas et al.70 reported the synthesis and antibacterial activities of Ru-004 (later HMR3004), which possesses a quinoline ring, and it was a prototype of telithromycin71 (TEL). The C-3 position of these derivatives is a carbonyl group, and these analogues are known as “ketolides.” TE-80272 showed antibacterial activities against EM resistant Staphylococcus aureus (MIC, 0.39 µg/mL), resistant Klebsiella pneumoniae (MIC, 6.26 µg/mL), and highly resistant S. pneumoniae (MIC, 6.25 µg/mL), although CAM was not effective against these strains. Ru-00473 showed antibacterial activities not only against inducible resistant S. pneumoniae (MIC, 0.02 µg/mL) but also against resistant S. pneumoniae constitutively expressing an erm gene (constitutive resistant S. pneumoniae) (MIC, 0.15 µg/mL).
Because TE-802 has a characteristic structure, generation of structurally related and patent-free lead compound(s) was difficult. On the other hand, a synthetic strategy to comprehensively introduce an arylalkyl group to the lactone ring was suggested to be appropriate if the arylalkyl group of Ru-004 was deeply concerned with the activity against constitutive resistant S. pneumoniae. According to this hypothesis, major macrolide research groups all over the world focused on the design, synthesis, and biological evaluation of novel arylalkylated 14-membered macrolides. Clinical trials of TEL71 began in 1998 in European countries, and it was approved for the first time in France in 2001. The ketolides cethromycin74 and solithromycin75 (Figure 19), which possess an arylalkyl group are currently under a late stage of development. Cethromycin was granted the designation of an orphan drug for anthrax in the US in 2007. A current study has explained the scientific relationships76 between these arylalkyl moieties and their antibacterial activities against resistant bacteria. However, to date, no macrolide antibiotic without drawbacks, including serious side effects77 or extremely bitter taste,78 and which is effective against constitutive resistant S. pneumoniae has been launched. Thus, we decided to develop a 16-membered macrolide, which was safe, metabolically stable, and effective against resistant S. pneumoniae with an erm gene.
2.3. New Aspect and Limitations of Chemical Modifications at the C-3 Position of 16-Membered Macrolides.
We observed that replacement of a 3-O-propionyl group of MDM by a quinolin-2-yl-carbonyl group partially enhanced the antibacterial activities against both susceptible and resistant S. pneumoniae.58 In addition, Tanikawa et al.79 modified the 3-OH group using an acyl moiety and generated acylides (Figure 19). One of the acylides, TEA0777, showed increased antibacterial activities against resistant Gram-positive bacteria. A combination of NMR spectroscopy and molecular modeling of Ru-004 (Figure 19) indicated80 that, in solution, the quinoline ring was anchored to the two carbonyl groups, 1-CO and 3-CO, by electrostatic interactions. On the basis of these findings, we decided to synthesize a variety of 3-O-(3-aryl-2-propenyl) derivatives of LM-A7.
Initially, we introduced an allyl group to the 3-OH group of the appropriately protected intermediate (44) (Scheme 9). Compared to a conventional method, palladium-catalyzed allylation81 provided 44 in an improved yield. Heck reactions of compound 56 with a variety of arylhalides afforded desired coupling products (57a-57m) accompanied with minor products with isomerized double bonds. Isomerization of the double bond in the allyl moiety at the C-3 position could be effectively prevented using palladacycle82 or palladium dichloride. A couple of deprotection processes yielded structurally novel analogues, 59a-59m in moderate yields.83
Majority of the derivatives among 59a-59m showed stronger antibacterial activities than LM-A7, except 59k (4-(trifluoromethyl)phenyl) and 59l (biphenyl) (data not shown). Among compounds 59a-59j and 59m, 59a (quinolin-3-yl) and 59m (4-(imidazol-1-yl)phenyl) showed the strongest antibacterial activities against S. pneunomiae and S. pyogenes. Although these compounds had weak antibacterial activities against constitutive resistant S. pneumoniae (MIC, 16 to 64 µg/mL) (Table 9), they were the first examples in our research group, which could respond to constitutive resistant S. pneumoniae or S. pyogenes. Because compound 59a showed a well-balanced antibacterial spectrum, we subsequently synthesized a 3-O-(3-quinolin-3-yl-2-propenyl) analogue of RKM, 60 (Figure 20) using a procedure similar to that used to synthesize 59.
Contrary to our hypothesis, the antibacterial activities of 60 were lower than those of RKM. The antibacterial activities of 60 were about 2 to 4 times weaker against S. pneumoniae and 16 to 32 times weaker against clinically important Gram-negative pathogens in respiratory infections (data not shown). Thus, introduction of a 3-quinolin-3-yl-2-propenyl group to the 3-hydroxyl group of LM-A7 increased the antibacterial activities, but introduction of the same group to the corresponding position of RKM showed a marked decrease in its antibacterial activities. These differences could be explained on the basis of findings reported Takenuki84 in that the propionyl group at the C-3” position of RKM 18-methylhemiacetal was located in the space close to direction of the C-3 position (Figure 20). Conformational analysis of 60 showed that the 3-quinolin-3-yl-2-propenyl group of 60 overlapped with the original position of the propionyl group at the C-3” position, and thus, a carbohydrate moiety changed its three-dimensional position to avoid the quinoline ring. Changing of the three-dimensional structure of 60, especially the relative positioning between the lactone ring and the disaccharide moiety, was supposed to reduce its biological activities. Because it was judged that chemical modification at the C-3 position would be difficult to discover a novel interaction with ribosomal RNA for enhancement of antibacterial activities against resistant S. pneumoniae, we had to reconsider and precisely analyze the important role of an arylalkyl moiety of Ru-004.
2.4. Introduction of an Arylalkyl Moiety onto the Western Hemisphere85 in 16-Membered Macrolactones
Part 1: Regio- and Stereoselective Epoxidation and Regioselective Ring Opening Using Sodium Azide86
TEL and Ru-004 possess an arylalkyl moiety attached to 11, 12-cyclic carbamate in the western hemisphere of 14-membered macrolactone (Figure 19). The arylalkyl group protected87 the A752 residue in domain II of ribosomal RNA against chemical modification. These observations suggested that the aryl side chain might interact with the second binding site in rRNA. Furthermore, X-ray crystallographic analysis of the ketolide-ribosome complex indicated76 that the aryl group of TEL or ABT-773/cethromycin bound to domain II through stacking or hydrogen bonding. Thus, the aryl moiety is significantly important for antibacterial activities against EM-resistant pathogens. Subsequently, we designed novel 16-membered macrolides, which possess an arylalkyl moiety attached to a cyclic carbamate in the western hemisphere.
Muroi et al.88 reported stereoselective and non-regioselective epoxidation at the C-10 to C-13 positions on JM/LM-A3. To improve regioselectivity of epoxidation, we explored several substituents at the C-9 position. Our results showed that regioselectivity of epoxidation at the C-12 and C-13 positions was greatly improved when we used the 9-O-acetyl-type intermediate (62) with mCPBA. This oxidation step also converted the 3’-dimethylamino group to its amine oxide and thus it was consequently reduced back to the original dimethyl amino group by treatment with sodium hydrosulfite. A structure of the epoxide (63) was confirmed by X-ray crystallographic analysis.89
Ring-opening reaction of the epoxide with sodium azide proceeded at the allylic position to afford an azide (64), which was converted to an amine (65) by triphenylphosphine. 2’-Acetate of 65 (66) was reacted with benzaldehyde in the presence of sodium triacetoxyborohydride followed by treatment of 1,1’-carbonyldiimidazole to afford N-benzyl-12,13-N,O-cyclic carbamate (68) (Scheme 10). On the other hand, alternative arylalkyl derivatives, 72b-72d, were prepared from compound 65 via 12-N-arylalkyl-13-hydroxyl intermediates (71b-71d). The yields of 71 were not satisfactory because of formation of undesired 12-N,N-dialkyl derivatives. Deprotections of these intermediates gave the original molecules, 70a-70d,90 accompanied with a reference analogue (69). Absolute configurations at the C-12 and C-13 positions were determined by NOE experiments.90
Although the antibacterial activities of 70a-70d against S. pneumoniae (susceptible and inducible resistant) were better than those of MDM (data not shown), their activities were generally comparable to those of MDM. We supposed that the construction of a cyclic carbamate in the western hemisphere in 16-membered macrolide was not always an appropriate approach for increasing the antibacterial activities against EM-resistant pathogens. Therefore, we decided to investigate the fundamental SAR of derivatives without a cyclic carbamate.
2.5. Introduction of an Arylalkyl Moiety onto the Western Hemisphere85 in 16-Membered Macrolactones
Part 2: Optimization of an Arylalkylamino Moiety at the C-12 Position86
On the basis of the synthetic route described above (Scheme 10), we synthesized several analogues possessing an arylalkyl group at the western hemisphere without a cyclic carbamate. We synthesized a dimethylamino analogue (73) and seven phenyl alkylene analogues (74a-74g)90 (Figure 21). Compound 74g showed weaker antibacterial activities than 74a-74f against S. pneumoniae (susceptible and inducible resistant). In vitro antibacterial activities against S. pneumoniae (Table 10) indicated that the length of an alkyl spacer was optimized as n = 3, i.e., propylene. Because introduction of a substituent to the benzene ring of compound 74c did not enhance their antibacterial activities, we therefore optimized an aryl moiety by synthesis and evaluation of aromatic analogues (75 to 79) (Figure 21). Chemical structures of aromatic rings in 77, 78, and 79 were referred to those of ABT-773/cethromycin, Ru-004, and TEL, respectively.
Transformation of a benzene ring to a pyridine ring (74c to 75) did not improve antibacterial activities, but introduction of a nitrogen atom to a naphthalene ring (76 to 78) enhanced antibacterial activities against important Gram-positive pathogens in respiratory infections (Table 11). In conclusion, a novel 16-membered macrolide possessing a [3-(quinolin-4-yl)propyl]amino moiety at the C-12 and a hydroxyl group at the C-13 position showed moderate antibacterial activities against inducible resistant S. pneumoniae and responded to constitutive resistant S. pneumoniae. To consider whether we should continue to investigate the western hemisphere of 16-membered lactone, we discussed about possibilities that an arylalkyl moiety of the western hemisphere interacted with the domain II in the rRNA.
Then, we synthesized two tailor-made analogues of 74c, compounds 8091 and 81 (Figure 22). Compound 80 had a phenylpropenyl group at the C-3 position and compound 81 had an acetyl group at the C-3” position. Compounds 80 and 81 showed 4 to 8 times stronger antibacterial activities than 74c against inducible resistant S. pneumoniae. Moreover, while compound 80 showed weak activities against constitutive resistant S. pneumoniae and constitutive resistant S. pyogenes, RKM, CAM, or compound 81 had no effect on these pathogens.
Our results indicated that the antibacterial activities of our novel 16-membered macrolides modified at the western hemisphere against resistant Gram-positive bacteria could be enhanced with further modifications at the C-3 or at the C-3” positions. Therefore, we performed novel modifications at the western hemisphere of 16-membered macrolides.
2.6. Introduction of an Arylalkyl Moiety onto the Western Hemisphere in 16-Membered Macrolactones
Part 3: An Alternative Approach, Synthesis of 13-Amino-12-Hydroxyl Analogues86
The results of our SAR studies indicated that (i) the structure of an aryl moiety and (ii) the length of an alkyl spacer were very important for strong antibacterial activities. Promising derivatives synthesized to date (compounds 59a, 59m, 74f, 76, 78, and 80) could only respond to constitutive resistant S. pneumoniae, and their antibacterial activities were weaker than those of ketolides. Therefore, we decided to increase the antibacterial activities against constitutive resistant S. pneumoniae using 16-membered macrolides. We introduced an arylalkyl group only to the hydroxyl group at the C-3 position and/or the nitrogen atom at the C-12 positions in our derivatives. On the other hand, (i) introduction of an arylalkyl group to the 9-hydroxyl group and (ii) application of a 10,11-epoxide were not found to be useful as per the results of our in-house SAR studies. Therefore, we explored the synthesis and biological evaluation of 13-arylalkylamino-12-hydroxyl analogues92 as a new class of macrolides.
12-Amino-13-hydroxyl intermediate (66) was consequently treated with 2-nitrobenzenesulfonyl chloride and then methanesufonyl chloride to afford a 12-nosylamino-13-mesylate (82), which was converted to an N-nosylaziridine (83) under basic conditions (Scheme 11). A trifluoroacetate anion regioselectively attacked at the C-12 position from the β-face and work up treatment gave a 12-hydroxyl-
13-nosylamino analogue (84). Methylation of the 13-amino group and deprotection of the nosyl group followed by reductive alkylation in application of 3-(quinolin-4-yl)propanal and sodium cyanoborohydride constructed a total framework of a desired 13-arylalkylamino-12-hydroxyl intermediate (87). After isolation of 87, the 12-hydroxyl group was oxidized by Dess-Martin periodinane to a ketone, and then was reduced to a hydroxyl group by sodium borohydride. In this 2-step process (reaction condition [g] in Scheme 11), there were some possibilities that the C-13 position was isomerized. The yield of oxidation was very low, and the stereochemistry at the C-12 and C-13 positions could not be determined even after deprotections. The final product (88), however, could be isolated with high purity. On the other hand, 3”-O-acetyl analogue of 88, compound 89, was independently synthesized from MOM using a procedure similar to that used for 88.
Compounds 88 and 89 showed strong antibacterial activities against S. pneumoniae and S. pyogenes and their activities against constitutive resistant S. pneumoniae were markedly stronger than those of relatively potent 16-membered macrolides synthesized thus far (compounds 59a, 59m, 74f, 76, 78, and 80). Although we analyzed the possibility of performing further studies on 13-arylalkylamino analogues in the future, we did not decide to focus on further optimization of these novel analogues because of the following reasons: (i) many synthetic steps, (ii) unclear stereochemistry at the C-12 and C-13 positions, and (iii) very low yield of the oxidation step (reaction condition (g) (1) in Scheme 11). Therefore, we had to explore a novel research strategy.
3. From Natural Products to Innovative Transformation of Macrolide Framework: Synthesis of Novel Azalides86
3.1. Reported Azalides93 and Selection of an Alternative Macrocyclization Reaction
In the previous chapter (chapter 2), we confirmed that introduction of an arylalkyl group to the western hemisphere of the 16-membered lactone was very important for its antibacterial activities against EM-resistant Streptococcus species. Because we could establish a synthetic method to introduce a substituent to an α-amino group at the C-12 position, we subsequently planned to synthesize 15-membered 11-azalides, which possess an arylalkyl group at the N-11 position (Figure 23, right bottom). Since the method of synthesis of AZM reported by Pliva in 1981 (foreign application priority data of the original substrate patent of AZM is March 6, 1981 in Yugoslavia), many novel azalides have been synthesized94-99 (Figure 23). These examples included 13-membered to 17-membered azalides. Because the position of the nitrogen atom in all derivatives was “9” (next to the C-8 position) or “10” (next to the C-9 position), our target molecules, 11-azalide, were not known with a lactone ring of any size. On the other hand, several examples of synthesis including chemical reconstruction of a lactone ring, were reported in compounds 90, 91, 93, and 96, but the introduction of an arylalkyl
group to the lactone ring has not been reported thus far. Moreover, any azalides, which were effective against constitutive resistant S. pneumoniae were previously unknown.
After we started research on azalides, Taisho Pharmaceutical Co., Ltd. reported synthesis of 15-membered 12-azalides.100 Sugimoto et al.101 introduced a variety of substituents into an azalactone (C-9, C-13, or C-14 positions) and a neutral sugar moiety, and generated compounds 98-100 (Figure 24), which were effective against EM-resistant S. pneumoniae. The derivatives are also known as 11a-azalides. Although novel azalides have been recently reported,102 the novel structure of an azalide, except that of the above 15-membered 12-azalide, has not been reported thus far.
To synthesize our target molecules, 15-membered 11-azalides (Figure 23), macrolactonization was supposed to be a general approach as the key cyclization reaction. However, in 1990s, total synthesis103 of important natural products, including rapamycin (double Stille coupling) and epothilone A (ring closing metathesis) was achieved without macrolactonization, because this process did not always provide a satisfactory yield. Moreover, macrolactonization was believed to be too complicated or result in insufficient yield of antiinfective agents in pharmaceutical industries. Therefore, we tried to apply reductive alkylation as a macrocyclization reaction. This means one-step macrocyclization using “a linear dialdehyde and an amine.” Synthesis of at least 4 compounds104 with a 7-membered ring was performed using the one-step cyclization using a linear dialdehyde and an amine in 2009 (Scheme 12); however, to our knowledge, no compounds with an 8-membered ring or more were synthesized using this method. In 1982, Ōmura et al.105 reported that the basic framework of the 16-membered macrolide, JM/LM-A3, except an aldehyde group at the C-18 position, was quite stable under hydride reduction conditions for reductive alkylation/reductive amination.
Typically, 8- and 9-membered lactones106 are very difficult to be synthesized using lactonization (Figure 25). In the case of cyclic ether formation, a specific ring size is not observed (Figure 26). On the other hand, 8- to 11-membered cyclanes107 are highly strained (Figure 27). However, no information is available about relationships between the ring size and the difficulties associated with one-step macrocyclization using a linear dialdehyde and an amine. In our collaborative conformational analysis108 about one-step macrocyclization, 14- and 16-membered azacyclanes would be easily formed compared to 8-, 10-, and 12-membered azacyclanes in a model study. The results of these analyses are still preliminary, and more results are required to publish these data elsewhere.
3.2 Isolation of a Linear Dialdehyde and its One-Step Macrocyclization109
We selected a 15-membered 11-azalide (107) and a 16-membered diazalide (110) as prototype compounds and investigated their synthetic route (Scheme 13). Because we planned to use oxidative ring opening reaction using lead tetraacetate for formation of the dialdehyde (104), the 2’-hydroxyl group or 3’-dimethylamino group in addition to the aldehyde group at the C-18 position required to be protected. Although we have already established an N-oxide protection of the 3’-dimethylamino group, poor reproducibility was often observed in the deprotection process with triphenylphosphine, particularly on a large scale. Thus, we decided to protect the 2’-position and prepared a completely protected intermediate (102). Oxidation of 102 by using osmium tetroxide with N-methylmorpholine-N-oxide as a cooxidant yielded a tetraol (103), which was converted to the desired key intermediate (104) by ring cleavage using lead tetraacetate. This dialdehyde was very reactive and unstable, but it could be purified by silica gel column chromatography to afford
pure 104, which showed one doublet (δ 9.59, H-10) and one triplet (δ 9.75, H-13 as the numbering before ring opening) in 1H NMR spectroscopy (CDCl3). Owing to its instability, we used 104 without purification after determination of its structure. One-step macrocyclization of 104 with 1.1 eq. of benzylamine and 3.9 eq. of sodium cyanoborohydride in ethanol in the presence of excess acetic acid afforded an 11-azalactone (105) possessing a novel framework in a 10% yield. Sequential deprotections yielded the desired 15-membered 11-azalide (107) as the first proto-type molecule. In NMR analysis of compound 107, NOEs were observed between methylene protons in the benzyl group and the protons at the C-10 and C-12 positions, respectively, and interactions between the methylene protons in the benzyl group and the carbons (C-10 and C-12 positions) were observed in the HMBC experiment. The structure of compound 107 was confirmed by these magnetic resonance data. Similarly, one-step cyclization of 104 with 1.1 eq. of 1,2-dimethylhydrazine hydrochloride afforded a 16-membered diazalactone (108) and following deprotections yielded the 16-membered diazalide (110).
Compared to MOM, compounds 107109 and 110 showed slightly reduced antibacterial activities (data not shown), and further modifications were suggested to use these molecules as lead compounds. On the other hand, antibacterial activity of a fused cyclic carbamate analogue (111) against inducible resistant S. pneumoniae was 16 to 32 times weaker than that of MOM. Thus, we determined whether 107 or 110 should be selected on a priority basis as a lead compound. When we used “unsymmetrical” dialkylhydrazine instead of (symmetrical) dimethylhydrazine, we could not isolate each regioisomer even before or after deprotection(s). Therefore, we decided to select compound 107 as the lead molecule.
The first synthetic route of 15-membered azalides had two major problems as follows: (i) very low yields of key oxidation steps and (ii) negative impact on human health (osmium tetroxide) and the environment (lead tetraacetate) because of the oxidative reagents (Scheme 13). Sasai et al.110 reported an alternative synthetic route of 15-membered azalide by using ozone oxidation (Scheme 14). This route of synthesis did not require protection of the 2’-hydroxyl group and markedly improved the total yield. Although ozone oxidation was difficult to control in a large-scale reaction, LONZA111 has already industrialized the ozone oxidation process to a ton-scale. On the other hand, we prepared the 11-NH derivative (114) as the common intermediate in the downstream for efficient medicinal chemistry, via hydrogenolysis of an 11-N-p-methoxybenzyl analogue of 105.
3.3. Optimization of a 15-Membered 11-Azalide and Expansion of the Scope of Azalide Research
Initially, we optimized the length of an alkyl spacer between a benzene ring and a 15-membered azalactone. When the substituent at the N-11 position was a benzyl group, deacetylation of the 2’-hydroxyl group proceeded in a regioselective manner (Scheme 13). In the case of substituents longer than the benzyl group (a phenethyl group or longer), deacetylation of the 9-hydroxyl group was partially observed along with complete deacetylation at the 2’-position. Therefore, we prepared compounds 115a-115c and 116a-116d (Figure 28).
Almost all derivatives (115 and 116) except 115c showed strong antibacterial activities against susceptible and resistant Streptococcus species with an mef gene (Table 13). Because compounds 116b and 116c (a C3 or C4 spacer) showed optimum antibacterial activities, including those against inducible resistant S. pneumoniae, we optimized an aromatic part with a spacer of a C3 length (Figure 28). Additionally, we focused on (i) the nature of the bond between a substituent and an azalactone and (ii) the degree of unsaturation of a spacer moiety. Among compounds 117 to 124, compounds 119 (a hydrazine type) and 121 (a triple bond type) did not possess antibacterial activities against inducible resistant S. pneumoniae, and the antibacterial activities against resistant S. pneumoniae were greatly affected not only by a structure of an aromatic ring but also by that of a spacer. All 15-membered 11-azalides similar to 16-membered macrolides showed strong activities against resistant S. pneumoniae with an mef gene. Compounds 117 (pyridin-4-yl), 122 (6-methoxyquinolin-4-yl), and 123 (quinolin-4-yl) showed relatively stronger antibacterial activities against inducible resistant S. pneumoniae (Table 14). Our working hypothesis that introduction of an arylalkyl group to the western hemisphere of azalide can generate a novel macrolide effective against resistant S. pneumoniae was supposed to be scientifically valid. Therefore, we continued to perform chemical modifications at the western hemisphere, but even the strongest analogue (122) did not respond to constitutive resistant S. pneumoniae and S. pyogenes.
The comprehensive SAR data about 16-membered macrolide derivatives obtained in our laboratory showed that 3-O-modified 59a and 59m responded to constitutive resistant S. pneumoniae (MIC, 16 to 64 μg/mL), and 13-N-modified 88 and 89 showed moderate antibacterial activities against constitutive resistant S. pneumoniae (MIC, 8 to 16 μg/mL). Because the optimized 15-membered 11-azalide did not respond to constitutive resistant S. pneumoniae, we had to develop an advanced or alternative chemical modification at the western hemisphere of the 16-membered azalactone.
Thus, our results indicate that three-dimensional design of an aryl moiety is supposed to be very important to generate a novel macrolide, which exhibits strong antibacterial activities against resistant Streptococcus species. Our first approach (sections 3.2. and 3.3.) using a dialdehyde (104) (Figure 29), however, enabled us to structurally diversify only a small area in an original lactone moiety. Then, we used 9-formylcarboxylic acid (125) as a key intermediate for sequential macrocyclization to provide a variety of novel templates.
3.4. Preparation of 14- to 16-Membered Novel Azalides and Azalactams112 and Template Selection
A reactive linear dialdehyde (104) was reacted with 1.5 eq. of 1,8-diazabicyclo[5,4,0]undec-7-ene (DBU) at room temperature and yielded a key intermediate formylcarboxylic acid (125) (Scheme 15). Although the yield of this β-elimination was 35%, the overall two-step yield starting from the tetraol (103) without isolation of 104 was 52%. Compound 125 could be prepared from 104 by only standing at room temperature as a chloroform solution in the presence of anhydrous sodium sulfate. An aldehyde of 125 was reacted with an amino alcohol possessing an arylalkyl moiety under reductive amination conditions to afford a seco acid (126) in 65% yield. We used the Yamaguchi protocol to convert the seco acid to a desired 15-membered azalactone (127) in 43% yield followed by deprotections to yield novel 15-membered azalides (130 and 131). The yield was not optimized in the macrolactonization reaction because a 2,3-double bond-type analogue was formed as a byproduct. Slow addition of a DMAP solution could suppress the formation of a dimer during macrolactonization (Scheme 15). Addition of the solution at a normal speed resulted in 29% of 127 and 22% of the dimer.112 The acetyl group at the C-9 position was partially removed according to a neighboring effect by a tertiary amine at the N-11 position. Meanwhile, a deprotection step of the acetyl group at the C-2’ position gave two series of analogues, a 9-OAc type and a 9-OH type, and these phenomena were ideal for our comprehensive SAR studies. Longer reaction time for deacetylation using methanol resulted in methanolysis of the ester bond in the lactone. Synthesis of a 15-membered azalide by using this method was superior to the one-step macrocyclization using the linear dialdehyde (104) because of (i) the possibility of preparation of azalides with different structures and (ii) an improved total yield.
The 14-membered azalactam (135) could be prepared using a procedure similar to that used to prepare 130 and 131 starting from 125 (Scheme 16). When we introduced a diamine moiety, an aminoazide (Ar(CH2)4NH(CH2)2N3) provided a better yield under reductive amination conditions than a mono-N-protected diamine (Ar(CH2)4NH(CH2)2NHR). In addition, we prepared a common 11-NH-type intermediate (136) for efficient medicinal chemistry. Because compounds 130 and 131 showed moderate antibacterial activities against inducible resistant S. pneumoniae (Table 15), we planned to perform comprehensive medicinal chemistry in application of the macrolactonization methodology (Scheme 15). We disclosed our plan for the first time at an in-house research conference, and some time was required before implementation of our research plan. As mentioned in section 3.1., macrolactonization did not always provide a satisfactory yield, and it was believed to be too complicated or result in insufficient yield for medicinal chemistry of antiinfective agents in pharmaceutical industries at that time.
A member of our research group has joined a research project called “total synthesis of swinholide A” in the K. C. Nicolaou113 group at The Scripps Research Institute. To optimize macrolactonization of a seco acid (137) for preparation of a dimer (139), they evaluated different approaches. One of these approaches was an “original” Yamaguchi protocol, which could consistently afford a 22-membered lactone (138) in 69% yield (Scheme 17). These results provided us with a good opportunity to continue our research using a formylcarboxylic acid (125), because (i) 14- to 16-membered lactones were supposed to be as easily cyclized as a 22-membered lactone (Figure 25) and (ii) many improved Yamaguchi protocols had already been reported.
On the basis of the synthetic routes shown in Schemes 15 and 16, we synthesized a variety of 14- to 16-membered azalides and azalactams (Figure 30). The 14-membered azalactone analogue (140) did not show strong antibacterial activities. A prototype azalactam (135) and an N-methylamide analogue (148) did not respond to inducible resistant S. pneumoniae.
Antibacterial activities of the selected azalides and azalactams are showed in Tables 15 and 16. Generally, the azalides had stronger antibacterial activities against Streptococcus species than the azalactams. On the other hand, azalactams showed relatively stronger activities against clinically important Gram-negative pathogens in respiratory infections112 (data not shown). Among azalides, the 16-membered analogues (143 to 146) showed remarkable antibacterial activities against inducible resistant S. pneumoniae. Therefore, we decided to select the 16-membered azalide (azalactone derivative) as a template for further medicinal chemistry.
3.5 Discussion about the Position for Introduction of an Arylalkyl Moiety at the Western Hemisphere112
We were able to select a 16-membered 11-azalide as a template for further medicinal chemistry; thus, we subsequently determined the position at the western hemisphere at which an arylalkyl moiety could be introduced. The SAR studies performed in-house indicated that the biological activities of the derivatives could be increased only to a limited extent by the introduction of a substituent at the 9-hydroxyl group or the 11-nitrogen atom. Therefore, we planned to introduce the arylalkyl moiety at the C-13 position and the C-15 position by carbon-carbon bonding.
Initially, we synthesized 13-substituted analogues (Scheme 18). There are various strategies to construct a meaningful and powerful “focused library by small molecules” derived from structurally complicated natural products such as anticancer agents or antibiotics. As characteristic examples, one-by-one synthesis and its exact structure determination by conventional approach or split-and-pool methodology for peptide synthesis are well known. On the other hand, attractive strategies such as “chemical biology studies”114 and “click chemistry”115 were subsequently introduced as novel approaches to construct a high-quality library. Then, we used (+)-3-methylaminomethyl-5-hexen-1-ol116 for reductive amination of 155 and isolated the diastereoisomers 156 and 157; compound 156 was a less polar isomer with an Rf value 0.34, and compound 157 was a polar isomer with an Rf value 0.14 determined using chloroform:methanol (5:1). Finally, each diastereoisomer was separately converted to the desired 13-substituted 16-membered azalide, but their absolute configurations at the C-13 were not determined. Macrolactonization was successfully performed using the Shiina protocol117 using 2-methyl-6-nitrobenzoic anhydride (MNBA) instead of the acid chloride. When we used a novel approach118 by using tri-tert-butylphosphine in the Heck reaction, we mainly detected an isomer generated by rearrangement of the double bond. Moreover, in some cases, small amounts of cis isomers were detected along with major trans isomers. In this series (Scheme 18), Heck reactions with other bromoquinolines, for example, 6-bromoquinoline, 8-bromoquinoline, or 3-bromoisoquinoline, resulted in a very low yield (15% to 25%).
We simultaneously performed synthetic studies of 15-substituted 16-membered azalides (Scheme 19). Heck reaction of 167 or 168 with several kinds of bromoheterocyclic compounds afforded the desired coupling products with higher yields than those obtained after the reactions with 158 or 159; further, we did not detect product generated through double bond rearrangement. We prepared both diastereoisomers at the C-15 position and did not determine their stereochemistry. However, these compounds had sufficient antibacterial activities (vide post, Table 18) for further modifications, and thus we determined their stereochemistry at the C-15 and established the optimal method for synthesis of the single diastereoisomer of 15-substituted 16-membered azalides. To synthesize the β-isomer only at the C-15, we prepared compound 168 in a stereoselective manner. The coupling reaction of 155 with (R)-7-methylamino-1-hepten-4-ol119 yielded (15R)-166 (a seco acid of 168) as a single diastereoisomer, which was converted into the key intermediate (168). Kanemoto119 prepared (R)-7-methylamino-1-hepten-4-ol from D-ornithine hydrochloride in 8 steps.
We comprehensively synthesized a variety of 13-substituted and 15-substituted 16-membered azalides (Figure 31) according to the synthetic routes indicated in Scheme 18 and Scheme 19. 13-Substituted analogues and 15-substituted analogues typically showed comparable antibacterial activities. Among the 13-substituted analogues, compounds 165, 177, and 179 did not show antibacterial activities against inducible resistant S. pneumoniae. Among the 15-substituted analogues, compounds 173, 185 (both α-configuration at the C-15), and 188 (pyridinyl) did not show antibacterial activities against inducible resistant S. pneumoniae. Compound 174 (15-β-configuration) was 2 to 4 times more potent against a variety of pathogens than compound 173 (15-α-configuration).
Although the antibacterial activities of the 13-substituted analogues were comparable to the 15-substituted analogues (Tables 17 and 18), the 15-substituted analogues were slightly more potent. Compound 190 was the first example among 16-membered azalides, which showed weak antibacterial activity against constitutive resistant S. pneumoniae. Moreover, the problems associated with the 13-substituted analogues were very low yields and partial rearrangements of the double bond in Heck reactions. We performed conformational analyses of the β-isomer of 180 or 181, 186 (β-isomer), and ABT-773/cethromycin.89 The three-dimensional position of a quinoline ring of the minimized structure of 186 (a 15-substituted analogue) significantly overlapped with that of ABT-773/cethromycin. Therefore, we finally selected the 15-β-substituted 16-membered 11-azalide as a preferable template for the final optimization. Generally, the absolute configuration of the ω-position (the position next to an oxygen atom of the lactone linkage) in natural macromolecules is very important for their biological activities. In our 15-substituted 16-membered azalides, “unnatural” β-configuration showed stronger antibacterial activity than the natural α-configuration. These phenomena seem to be somehow rare in the chemistry of natural products. Kosan120 reported the synthesis and strong antibacterial activity of a 13-substituted 14-membered ketolide against resistant S. pneumoniae.
3.6 Molecular Design of a Neutral Sugar Moiety, which is Stable under Physiological Conditions121
Although a carbon framework of prototype compounds (174 and 186 to 193) of 15-β-substituted 16-membered 11-azalides (Figure 31) had been optimized, their C-3 position and a neutral sugar moiety in addition to their aryl moiety remained to be optimized. As described in sections 1.4. and 1.6., the in vitro potency of a 16-membered macrolide with a free hydroxyl group at the C-3 position was stronger than that with an acyloxy group at the C-3 position. Thus, we had to synthesize 3-OH-type analogues of the 15-substituted 16-membered azalides. The antibacterial activities of these azalides against inducible resistant S. pneumoniae were moderate or slightly weak (MIC, 2 to 8 µg/mL), and their neutral sugar moieties were unstable under physiological conditions, because the structure of a neutral sugar moiety of the above-mentioned prototype compounds was 3”-OH and 4”-O-COEt type. We therefore optimized the neutral sugar moiety before synthesizing 3-OH analogues or optimizing an aryl moiety in a substituent at the C-15 position. To build up a metabolically stable neutral sugar moiety, we designed and synthesized a macrolide belonging to a new chemical class, for example, compound 35d (Scheme 6), which possessed 4”-O-alkyl cladinose. Synthesis of a 4”-O-alkyl cladinose-type analogue of the 16-membered macrolide, however, was relatively difficult, and its in vitro antibacterial activities were not superior to those of the corresponding 4”-O-acyl cladinose-type molecule. Therefore, we explored a novel neutral sugar moiety that (i) could be easily synthesized, (ii) had enhanced activities, and (iii) was relatively stable against metabolism.
Omoto et al.122 reported that acetylation of a 4”-O-COEt neutral sugar (i) by using acetic anhydride and pyridine at a high temperature afforded a 4”-O-Ac-3”-O-COEt neutral sugar (ii) accompanied by intramolecular migration of a propionyl group (Scheme 20, left top). Considering this migration of the propionyl group, compound 168 was reacted with ethyl isocyanate in pyridine at 100 °C in a sealed tube to obtain a mixture of 3”-O-CONHEt derivative of 168 and a desired compound 195. After optimization of this reaction condition, we finally treated 168 with ethyl isocyanate in the presence of 1,4-diazabicyclo[2.2.2]octane (DABCO) in pyridine at 60 °C in a sealed tube to obtain a 93% yield of compound 195. In addition, compound 195 was prepared using an imidazolide (194). Heck reaction at the allyl group of compound 195, which possesses an unnatural neutral sugar, smoothly proceeded to afford a 68% yield of a quinolin-3-yl derivative (196a). Metabolic stability of the 15-substituted 16-membered azalide, which possesses a 3”-O-COEt-4”-O-CONHEt-type neutral sugar moiety, was significantly higher than that of an azalide, which possesses a 3”-OH-4”-O-COEt-type neutral sugar moiety. The 3”-OH-4”-O-COEt-type analogue was decomposed more than 90% in only 5 minutes in mouse liver S9, but about 50% of the 3”-O-COEt-4”-O-CONHEt-type analogue remained intact even after 1 hour in the same condition.123 Then, we optimized a carbamoyl group at the C-4” position.
A neutral sugar moiety of a key intermediate (197) was converted to 3”-O-COEt-4”-O-CONHR (or 3”-O-COEt-4”-O-CONMe2) type derivatives, and after deprotections, afforded compounds 198a to 198f (Scheme 20). All these analogues showed strong antibacterial activities against inducible resistant S. pneumoniae (MIC, 0.13 to 0.5 µg/mL) and resistant S. pneumoniae with an mef gene (MIC, 0.03 to 0.06 µg/mL).121 Further, almost all these analogues responded to constitutive resistant S. pneumoniae. Among them, compound 198a showed the strongest activities against the target pathogens, and the structure of the neutral sugar moiety was decided as 3”-O-COEt-4”-O-CONHEt. This indicated that the size of the substituents in the neutral sugar moiety of 198a was quite similar to that of RKM. Moreover, the metabolic stability of 198a in mouse liver S9 was markedly greater than that of MOM (Figure 32) and its stability in human liver S9 was completely comparable to that of TEL (Figure 33).89
3.7 Final Optimization of 15-β-Substituted 16-Membered 11-Azalides121
We used a key intermediate (195) with a metabolically stable neutral sugar moiety and screened an aryl moiety at the C-15 substituent (Scheme 21). Heck reaction of 195 and deprotections afforded five analogues. For the preparation of 196i only, Heck reaction required high temperature and activation by microwave.
Compared to 191 (3”-OH-4”-O-COEt), 198a (3”-O-COEt-4”-O-CONHEt) showed markedly increased antibacterial activities against resistant Streptococcus species after chemical transformation of the neutral sugar moiety (Table 19). Among these analogues, compounds 198i and 198k showed the strongest activities against constitutive resistant S. pneumoniae, and we continued the final optimization focusing on an isoquinoline ring (198i) and a 6-aminopyridine group (198k).
To achieve improved antibacterial activities, we synthesized 3-OH-type of 15-β-substituted 16-membered 11-azalides, which possess a metabolically stable neutral sugar moiety, focusing on an isoquinoline ring and a 6-aminopyridine group. This method of synthesis involved several approaches discussed thus far (Scheme 22). Starting from LM-A7 prepared by biotransformation38 of MDM with PF1083, a tetraol (201) was synthesized in application of Kitasato protection method42 (3,18-silyl hemiacetal). Subsequently, we converted the tetraol (201) to a key intermediate, 15-β-allyllactone (203) using our original method. The efficiency of macrolactonization of a seco acid was reported124 to be highly affected by the three-dimensional conformation and protecting groups of the seco acid. In our case, fortunately, macrolactonization proceeded to afford a 68% yield of a lactone (203) despite the presence of a fused 7-membered silyl hemiacetal.
There were two plausible routes for synthesis of 206, which were (i) condition (f) then (g) (Heck reaction, and then, neutral sugar transformation), and (ii) condition (g) then (f) (neutral sugar transformation, and then, Heck reaction).
Neutral sugar transformation, including a reaction with ethyl isocyanate, and intramolecular migration of a propionyl group in a sealed tube proceeded in 78-93% yield, and this reaction was not affected by a 7-membered fused silyl hemiacetal. Deprotections of compounds 206a-206c afforded the desired products 207a-207c. A quinolin-3-yl analogue (207a), an isoquinolin-4-yl analogue (207b), and a 6-aminopyridin-3-yl analogue (207c) showed strong antibacterial activities against target pathogens (Table 20), and their SARs observed for these compounds were similar to those observed during the screening of 3-O-COEt-type derivatives. We focused on the strong antibacterial activities of not only compounds 207a and 207b, which possess a fused aryl moiety, but also compound 207c with an amino group introduced even at the single aromatic ring such as pyridine. Therefore, we finally designed a hybrid-type molecule of compounds 207b and 207c and synthesized a 1-aminoisoquinolin-4-yl analogue (207e) via an important intermediate 206d prepared from the allyl intermediate 205 with 4-bromo-1-[2-(trimethylsilyl)ethoxycarbonylamino]isoquinoline. When we used this arylbromide protected by the Teoc group in Heck reaction with 205, partial rearrangement of the double bond at the 15-substituent was observed as the first example in 15-substituted derivatives. After 2-step deprotections of the acetyl groups and the silyl hemiacetal, a single double bond isomer could be isolated.
1-Aminoisoquinolin-4-yl analogue (207e) showed strong antibacterial activities against constitutive resistant S. pneumoniae (Table 20). Antibacterial activities of 207e against resistant S. pneumoniae with an erm gene were similar to those of TEL, and its activities against resistant S. pneumoniae with an mef gene and resistant S. pyogenes were clearly stronger than those of TEL. On the other hand, the antibacterial activity of 207e against H. influenzae was slightly weaker than that of TEL, but it was stronger than that of CAM or RKM.121 To our knowledge, 207e is the first leucomycin analogue derived from 16-membered macrolides that has optimal and strong activities against clinically important pathogens in respiratory infections.
Metabolic stability of 207e in mouse liver S9 was qualitatively confirmed to be comparable to that of 198a. We described that 3-OH-type 16-membered macrolides were relatively unstable than the 3-O-acyl-type macrolides because of less hindered lactone linkage in section 1.7. In the case of 15-β-substituted 16-membered 11-azalides, however, chemical or biological instability was not observed at all, probably because a large substituent at the C-15 position would stabilized the lactone linkage. Further studies on 207e, including evaluation of in vivo efficacy, pharmacokinetic studies, and toxicity studies, are currently underway.
CONCLUSIONS AND PERSPECTIVE
16-Membered macrolides have many sensitive functional groups, including an aldehyde, double bonds, an allylic alcohol, 2-deoxy sugar with less 1,3-diaxial interaction, and less hindered lactone linkage (Figure 34). Moreover, synthesis and analysis of macrolides have some limitations, such as, complicated NMR analyses, a time consuming purification process, and a lack of opportunities for crystallization. Therefore, for a long time, the major chemical modifications of 16-membered macrolides included simple introductions of acyl groups to hydroxyl groups.
We referred to the pioneer work about the cladinose analogue in a 16-membered macrolide reported by Tatsuta et al.20 and the alternative original research about 16-membered macrolides, which possess a 4”-O-alkyl neutral sugar moiety, reported by Sano et al.42 and Kiyoshima et al.47 We integrated their research concepts and designed and synthesized novel 16-membered macrolides (34d and 35d), which possess a 4-O-alkyl cladinose moiety, and proved that these novel macrolides had better pharmacokinetics and in vivo potency than the existing 16-membered macrolides did. We performed several novel chemical modifications to address the issue of the emergence of resistant Gram-positive pathogens, including S. pneumoniae and S. pyogenes. Our major strategies were (i) introduction of an arylalkyl group to the hydroxyl group at the C-3 position or the western hemisphere and (ii) construction of a variety of 11-azalides. Although we introduced an arylalkyl group to the hydroxyl group at the C-3 position or the western hemisphere, we could not generate promising derivatives that showed strong antibacterial activities against constitutive resistant S. pneumoniae. However, the introduction of an arylalkyl group to the western hemisphere of a 16-membered macrolide enhanced its antibacterial activities against inducible resistant S. pneumoniae. Thus, a more precise three-dimensional molecular design focusing on an arylalkyl group was required to improve antibacterial activities against constitutive resistant Streptococcus species. Therefore, we prepared 14-, 15-, and 16-membered azalides (azalactone derivatives) and azalactams, and selected a 16-membered 11-azalide as a novel template for further medicinal chemistry on the basis of its antibacterial activities against target pathogens. A position for the introduction of an arylalkyl group in the western hemisphere was decided as the C-15 position, according to synthetic efficiency (higher yield and no rearrangement of double bond) and in-house fundamental conformational analysis. The final optimization of the 15-β-substituted 16-membered 11-azalide at the C-3 position, an arylalkyl moiety, and a neutral sugar moiety generated compound 207e.
The antibacterial activity of compound 207e against constitutive resistant S. pneumoniae was similar to that of TEL, but it showed stronger antibacterial activity against resistant S. pneumoniae with an mef gene (efflux type) than TEL. Moreover, 207e showed 4 to 8 times stronger antibacterial activities than TEL did against constitutive and efflux-type resistant S. pyogenes. In conclusion, we synthesized a novel azalide with the desired profiles in efficiency and metabolic stability, by using a novel transformation of the carbon framework.
The development of a novel oral antibiotic is not very easy. Although ABT-773/cethromycin74 is in the final stage, its development required a relatively long time. Further, development of EDP-420/modithromycin125 was reported to be terminated. On the other hand, macrolide drug discovery programs115c, 126 are ongoing, and they focus on alternative target pathogens such as methicillin-resistant Staphylococcus aureus (MRSA) and vancomycin-resistant Enterococci (VRE). Although azalide research has been performed for a long time, macrolide chemists have to explore novel approaches to generate a novel macrolide or a novel azalide with improved clinical efficacy, safety, and taste. We still have many possibilities toward generation of novel macrolides and azalides with or without an arylalkyl group. Recent studies127 suggest that a novel azalide, which is clinically safe and effective against resistant Streptococcus species will be hopefully developed in Japan in the near future.
ACKNOWLEDGEMENTS
The authors wish to thank Dr. E. Kaji (Vice President, Kitasato Univ.), Dr. O. Hoshino (Prof. Emeritus, Tokyo Univ. of Science), and Dr. S. Kobayashi (Prof. Emeritus, Tokyo Univ. of Science) for supervision to our macrolide research. We also thank Dr. K. Tadano (Prof. Emeritus, Keio Univ.), Dr. H. Sasai (Prof., Osaka Univ.), Dr. T. Sugai (Prof., Keio Univ.), and Dr. M. Ikunaka (Prof., Yasuda Women’s Univ.) for collaboration. We are grateful to Dr. M. Ihara (Prof. Emeritus, Tohoku Univ.), Dr. K. Tomioka (Prof., Doshisha Women’s College), Dr. D. Uemura (Prof., Kanagawa Univ.), Dr. T. Honda (Prof. Emeritus, Hoshi Univ.), Dr. Y. Torisawa (Prof., Takasaki Univ. of Health and Welfare), Dr. H. Takayama (Prof., Chiba Univ.), Dr. X. Liu (Prof., Heibei Univ.), Dr. T. Sunazuka (Prof., Kitasato Univ.), Dr. K. Toshima (Prof., Keio Univ.), Dr. Y. Hayashi (Prof., Tohoku Univ.), Dr. S. Aoki (Prof., Tokyo Univ. of Science), Dr. K. Nagasawa (Prof., Tokyo Univ. of Agri. & Tech.), Dr. T. Hirose (Assoc. Prof., Kitasato Univ.), and Dr. H. Ishikawa (Assoc. Prof., Kumamoto Univ.) for valuable discussion for macrolide research. We are also grateful to Dr. T. Nakata (Riken (ret.)), Dr. T. Kitahara (Prof. Emeritus, The Univ. of Tokyo), Dr. N. Miyata (Prof. Emeritus, Nagoya City Univ.), Dr. H. A. Kirst (Eli Lilly and Co. (ret.)), Dr. L. Katz (Society for Industrial Microb. & Biotech.), Dr. G. W. Ashley (Kosan Biosciences), Dr. S. Morimoto, Dr. S. Omura, Dr. T. Asaka, and Dr. M. Kashimura (Taisho Pharmaceutical) for encouragement.
References
1. R. B. Woodward, Angew. Chem., 1957, 69, 50. CrossRef
2. a) T. Sunazuka, S. Omura, S. Iwasaki, and S. Ōmura, “Chemical Modification of Macrolides”, S. Ōmura (Ed), “Macrolide Antibiotics. Chemistry, Biology, and Practice” Second Edition, Academic Press Inc., 2002, pp. 99-180; b) T. Asaka, A. Manaka, and H. Sugiyama, Current Topics in Medicinal Chemistry, 2003, 3, 961; CrossRef c) H. A. Kirst, Expert Opin. Ther. Patents, 2010, 20, 1343. CrossRef
3. S. Morimoto, Y. Takahashi, Y. Watanabe, and S. Omura, J. Antibiot., 1984, 37, 187. CrossRef
4. a) G. Kobrehel and S. Djokić, JP 57158798 (1982) (BE 892357); b) G. M. Bright, A. A. Nagel, J. Bordner, K. A. Desai, J. N. Dibrino, J. Nowakowska, L. Vincent, R. M. Watrous, F. C. Sciavolino, A. R. English, J. A. Retsema, M. R. Anderson, L. A. Brennan, R. J. Borovoy, C. R. Cimochowski, J. A. Faiella, A. E. Girard, D. Girard, C. Herbert, M. Manousos, and R. Mason, J. Antibiot., 1988, 41, 1029; CrossRef c) S. Djokić, G. Kobrehel, N. Lopotar, B. Kamenar, A. Nagl, and D. Mrvos, J. Chem. Res., Synop., 1988, 152.
5. a) Cited from IMS data with their written permission; b) Cited from financial statements of each company and etc. Sales of clarithromycin: from Taisho Pharmaceutical and Abbott Japan only; Sales of azithromycin: from Pfizer only.
6. a) S. B. Taubman, N. R. Jones, F. E. Young, and J. W. Corcoran, Biochem. Biophys. Acta, 1966, 123, 438; CrossRef b) Y. Nakajima, “Mode of Action and Resistance Mechanisms of Antimicrobial Macrolides”, S. Ōmura (Ed), “Macrolide Antibiotics. Chemistry, Biology, and Practice” Second Edition, Academic Press Inc., 2002, pp. 453-499; c) C. Walsh, Nature Reviews Microbiology, 2003, 1, 65. CrossRef
7. a) H. A. Kirst and G. D. Sides, Antimicrob. Agents Chemother., 1989, 33, 1413; CrossRef b) H. A. Kirst and G. D. Sides, Antimicrob. Agents Chemother., 1989, 33, 1419. CrossRef
8. A. T-Kamradt, J. Clancy, M. Cronan, F. D-Hajj, L. Wondrack, W. Yuan, and J. Sutcliffe, Antimicrob. Agents Chemother., 1997, 41, 2251.
9. H. Sakakibara, O. Okekawa, T. Fujiwara, M. Otani, and S. Ōmura, J. Antibiot., 1981, 34, 1001. CrossRef
10. S. Omoto, K. Iwamatsu, S. Inouye, and T. Niida, J. Antibiot., 1976, 29, 536. CrossRef
11. a) K. Tatsuta, A. Tanaka, K. Fujimoto, M. Kinoshita, and S. Umezawa, J. Am. Chem. Soc., 1977, 99, 5826; CrossRef b) K. Tatsuta, Y. Amemiya, S. Maniwa, and M. Kinoshita, Tetrahedron Lett., 1980, 21, 2837; CrossRef c) K. Tatsuta, T. Kobayashi, H. Gunji, and H. Masuda, Tetrahedron Lett., 1988, 29, 3975; CrossRef d) K. Tatsuta, T. Ishiyama, S. Tajima, Y. Koguchi, and H. Gunji, Tetrahedron Lett., 1990, 31, 709. CrossRef
12. This research program (section 1.3 to 1.6) was predominantly performed by Mr. A. Shimizu, Mr. K. Kurihara, Miss N. Kikuchi (Mrs. Shiokawa), Dr. T. Ishizuka, Miss E. Tanaka (Mrs. Tokumaru), Miss M. Iida, Mrs. A. Miyata, and Miss K. Kakinuma (Mrs. Tohyama) under the direction of Drs. S. Shibahara, S. Omoto, O. Hara, M. Araake, S. Gomi, H. Suzuki, T. Yaguchi, and S. Miyadoh. Mr. Kurihara received his Ph.D. title with these works supervised by Professor Osamu Hoshino from Tokyo University of Science in 1997.
13. T. Lazarevski, G. Radobolja, and S. Djokić, J. Pharm. Sci., 1978, 67, 1031. CrossRef
14. S. Kudoh, J. Tamaoki, K. Nakata, H. Takizawa, and H. Goto, “Novel Activity of Erythromycin and Its Derivatives”, S. Ōmura (Ed), “Macrolide Antibiotics. Chemistry, Biology, and Practice” Second Edition, Academic Press Inc., 2002, pp. 533-569.
15. a) S. G. D’Ambrieres, A. Lutz, J. C. Gasc, FR 2473525 (1981); b) J. C. Gasc, S. G. D’Ambrieres, A. Lutz, and J. F. Chantot, J. Antibiot., 1991, 44, 313. CrossRef
16. T. Shomura, S. Someya, K. Umemura, M. Nishio, and S. Murata, Yakugaku Zasshi, 1982, 102, 781.
17. Toyo Jozo Research Center, Abstract of 31st Annual Meeting of Japan Chemotherapeutics Association, TMS-19-Q, p. 109, Jun. 3, 1983, Osaka, Japan.
18. M. Morikawa, M. Inoue, M. Tsuboi, T. Shomura, S. Someya, S. Murata, K. Umemura, and M. Sugiura, J. Pharm. Dyn., 1982, 5, 314. CrossRef
19. H. A. Kirst, J. Antimicrob. Chemother., 1991, 28, 787. CrossRef
20. a) K. Tatsuta, A. Tanaka, M. Kinoshita, and S. Umezawa, Chem. Lett., 1977, 769; CrossRef b) K. Tatsuta, K. Fujimoto, M. Kinoshita, and S. Umezawa, Carbohydr. Res., 1977, 54, 85. CrossRef
21. a) E. J. Corey, K. C. Nicolaou, and L. S. Melvin, Jr., J. Am. Chem. Soc., 1975, 97, 653; CrossRef b) A. K. Mallams and R. R. Rossman, J. Chem. Soc.,Perkin Trans. I, 1989, 799. CrossRef
22. R. B. Woodward, E. Logusch, K. P. Nambiar, K. Sakan, D. E. Ward, B.-W. Au-Yeung, P. Balaram, L. J. Browne, P. J. Card, C. H. Chen, R. B. Chenevert, A. Fliri, K. Frobel, H.-J. Gais, D. G. Garratt, K. Hayakawa, W. Heggie, D. P. Hesson, D. Hoppe, I. Hoppe, J. A. Hyatt, D. Ikeda, P. A. Jacobi, K. S. Kim, Y. Kobuke, K. Kojima, K. Krowicki, V. J. Lee, T. Leutert, S. Malchenko, J. Martens, R. S. Matthews, B. S. Ong, J. B. Press, T. V. R. Babu, G. Rousseau, H. M. Sauter, M. Suzuki, K. Tatsuta, L. M. Tolbert, E. A. Truesdale, I. Uchida, Y. Ueda, T. Uyehara, A. T. Vasella, W. C. Vladuchick, P. A. Wade, R. M. Williams, and H. N.-C. Wong, J. Am. Chem. Soc., 1981, 103, 3215. CrossRef
23. a) K. Suzuki and T. Mukaiyama, Chem. Lett., 1982, 683; CrossRef b) K. Toshima, S. Mukaiyama, T. Yoshida, T. Tamai, and K. Tatsuta, Tetrahedron Lett., 1991, 32, 6155. CrossRef
24. K. Ajito, K. Kurihara, A. Shimizu, M. Araake, O. Hara, and S. Shibahara (Meiji Seika Kaisha, Ltd.), Japan Kokai 211888 (1994), Aug. 2, 1994.
25. K. Ajito, A. Shimizu, K. Kurihara, T. Ishizuka, N. Kikuchi, A. Miyata, M. Araake, O. Hara, and S. Shibahara (Meiji Seika Kaisha, Ltd.), Japan Kokai 206897 (1994), Jul. 26, 1994.
26. K. Ajito, K. Kurihara, A. Shimizu, S. Gomi, N. Kikuchi, M. Araake, T. Ishizuka, A. Miyata, O. Hara, and S. Shibahara (Meiji Seika Kaisha, Ltd.), US Patent 5,407,918, Apr. 18, 1995.
27. L. A. Freiberg, R. S. Egan, and W. H. Washburn, J. Org. Chem., 1974, 39, 2474. CrossRef
28. a) H. Sakakibara, T. Fujiwara, M. Aizawa, and S. Ōmura, J. Antibiot., 1981, 34, 1577; CrossRef b) K. Tsuzuki, H. Matsubara, A. Nakagawa, and S. Omura, J. Antibiot., 1986, 39, 1784. CrossRef
29. a) M. Suzuki, N. Nagahama, Y. Seki, and T. Yamaguchi (Tanabe Seiyaku Co., Ltd.), Japan Kokai 126880 (1975), Oct. 6, 1975; b) K. Nagaoka, H. Ogawa, Y. Matsuhashi, K. Watanabe, T. Matsunobu, T. Shomura, and H. Goi (Meiji Seika Kaisha, Ltd.). Japan Kokai 8793 (1979), Jan. 23, 1979; c) Y. Matsuhashi, H. Ogawa, and K. Nagaoka, J. Antibiot., 1979, 32, 777; CrossRef d) S. Ōmura, C. Kitao, and N. Sadakane, J. Antibiot., 1980, 33, 911. CrossRef
30. T. Tsuruoka, S. Inouye, T. Shomura, N. Ezaki, and T. Niida, J. Antibiot., 1971, 24, 526. CrossRef
31. M. Suzuki, T. Furumai, K. Takeda, and T. Setoguchi (Tanabe Seiyaku Co., Ltd.), Japan Kokai 10288 (1973), Feb. 8, 1973.
32. K. Ajito, A. Shimizu, S. Shibahara. O. Hara. K. Kurihara. M. Araake, K. Tohyama, S. Miyadoh, S. Omoto, and S. Inouye, J. Antibiot., 1997, 50, 366. CrossRef
33. S. Inouye, S. Omoto, K. Iwamatsu, and T. Niida, J. Antibiot., 1980, 33, 61. CrossRef
34. a) N. A. Hughes, Carbohyd. Res., 1968, 7, 474; CrossRef b) J. S. Jewell and W. A. Szarek, Tetrahedron Lett., 1969, 43; CrossRef c) G. J. F. Chittenden, Carbohyd. Res., 1970, 15, 101. CrossRef
35. K. Ajito, K. Kurihara, S. Shibahara, O. Hara, T. Okonogi, N. Kikuchi, M. Araake, H. Suzuki, S. Omoto, and S. Inouye, J. Antibiot., 1997, 50, 150. CrossRef
36. S. Ōmura and C. Kitao, Hakko to Kogyo, 1979, 37, 749.
37. R. Okamoto, T. Fukumoto, K. Imafuku, T. Okubo, K. Kiyoshima, A. Takamatsu, and T. Takeuchi, J. Ferment. Technol., 1979, 57, 519.
38. A. Shimizu, S. Gomi, K. Ajito, T. Yaguchi, E. Tanaka, O. Hara, and S. Miyadoh (Meiji Seika Kaisha, Ltd.), US Patent 5,219,736, Jun. 15, 1993.
39. A. Shimizu, S. Gomi, T. Yaguchi, K. Kurihara, and K. Ajito, The 9th Symposium by Group Biocatalyst Chemistry Japan, Jan. 24, 1997, University of Shizuoka.
40. S. Ōmura and A. Nakagawa, J. Antibiot., 1975, 28, 401. CrossRef
41. K. Kurihara, K. Ajito, S. Shibahara, O. Hara, M. Araake, S. Omoto, and S. Inouye, J. Antibiot., 1998, 51, 771. CrossRef
42. H. Sano, T. Sunazuka, H. Tanaka, K. Yamashita, R. Okachi, and S. Ōmura, J. Antibiot., 1984, 37, 750. CrossRef
43. D. Ikeda, K. Ajito, S. Kondo, and T. Takeuchi, Chem. Lett., 1990, 1431. CrossRef
44. H. Sano, T. Sunazuka, H. Tanaka, K. Yamashita, R. Okachi, and S. Ōmura, J. Antibiot., 1984, 37, 760. CrossRef
45. J. D. Albright and L. Goldman, J. Am. Chem. Soc., 1967, 89, 2416. CrossRef
46. H. Sano, T. Sunazuka, H. Tanaka, K. Yamashita, R. Okachi, and S. Ōmura, J. Antibiot., 1985, 38, 1350. CrossRef
47. K. Kiyoshima, M. Sakamoto, T. Ishikura, Y. Fukagawa, T. Yoshioka, H. Naganawa, T. Sawa, and T. Takeuchi, Chem. Pharm. Bull., 1989, 37, 861. CrossRef
48. S. David and S. Hanessian, Tetrahedron, 1985, 41, 643. CrossRef
49. K. Kurihara, K. Ajito, S. Shibahara, T. Ishizuka, O. Hara, M. Araake, and S. Omoto, J. Antibiot., 1996, 49, 582. CrossRef
50. K. Ajito, K. Kurihara, S. Shibahara, O. Hara, A. Shimizu, M. Araake, and S. Omoto, J. Antibiot., 1997, 50, 92.
51. K. Ajito, A. Shimizu, N. Kikuchi, S. Gomi, O. Hara, and S. Shibahara (Meiji Seika Kaisha, Ltd.), Japan Kokai 33794 (1995), Feb. 3, 1995.
52. K. Kurihara, N. Kikuchi, and K. Ajito, J. Antibiot., 1997, 50, 32. CrossRef
53. S. Omura, S. Morimoto, T. Nagate, T. Adachi, and Y. Kohno, Yakugaku Zasshi, 1992, 112, 593.
54. Professor Dr. Satoshi Shuto, Laboratory of Organic Chemistry for Drug Development, Faculty of Pharmaceutical Sciences, Hokkaido University, Personal Communication, Jun. 19, 2001.
55. K. Ajito, K. Kurihara, S. Shibahara, O. Hara, S. Gomi, A. Shimizu, M. Araake, and S. Omoto, Abstract of 38th Symposium on the Chemistry of Natural Products, pp. 739-744, Oct. 14-16, 1996, Sendai, Japan.
56. K. Kurihara and K. Ajito, “Dramatic Improvement in Pharmacokinetics of Sixteen-Membered Macrolides”, 37/661 (2), “Recent Research Development of Antibiotics” First Edition, Transworld Research Network, 2002, pp. 141-169.
57. A. Inoue, T. Deguchi, M. Yoshida, and K. Shirahara, J. Antibiot., 1983, 36, 442. CrossRef
58. T. Furuuchi, K. Kurihara, T. Yoshida, and K. Ajito, J. Antibiot., 2003, 56, 399. CrossRef
59. H. W. Boucher, G. H. Talbot, J. S. Bradley, J. E. Edwards, Jr., D. Gilbert, B. Rice, M. Scheld, B. Spellberg, and J. Bartlett, Clin. Infect. Dis., 2009, 48, 1. CrossRef
60. A. L. Bisno, N. Engl. J. Med., 1991, 325, 783. CrossRef
61. a) G. C. Schito, A. Pesce, and A. Marchese, J. Antimicrob. Chemother., 1997, 39, 562; CrossRef b) M Bassetti, G. Manno, A. Collida, A. Ferrando, G. Gatti, E. Ugolotti, M. Cruciani, and D. Bassetti, Emerging Infectious Diseases, 2000, 6, 180. CrossRef
62. H. Goto, H. Takeda, S. Kawai, T. Watanabe, M. Okazaki, K. Shimada, K. Nakano, H. Yokouchi, H. Ikemoto, T. Mori, J. Igari, T. Oguri, M. Yamamoto, Y. Karasawa, K. Kudo, N. Kobayashi, T. Tanaka, M. Sumitomo, T. Matsushima, M. Oka, Y. Niki, M. Suga, H. Inoue, T. Nakadate, A. Suwabe, Y. Ashino, F. Gejyo, M. Okada, N. Aoki, N. Kitamura, Y. Suzuki, M. Tosaka, S. Kohno, Y. Hirakata, A. Kondou, J. Matsuda, M. Nakano, M. Nasu, K. Hiramatsu, and S. Oikawa, Jap. J. Antibiot., 2006, 59, 323.
63. a) D. Felmingham, R. R. Reinert, Y. Hirakata, and A. Rodloff, J. Antimicrob. Chemother., 2002, 50, Suppl. S1, 25; CrossRef b) D. J. Farrell and D. Felmingham, Antimicrob. Agents Chemother., 2004, 48, 1882. CrossRef
64. R. Isozumi, Y. Ito, T. Ishida, M. Osawa, T. Hirai, I. Ito, K. Maniwa, M. Hayashi, H. Kagioka, M. Hirabayashi, K. Onari, H. Tomioka, K. Tomii, I. Gohma, S. Imai, S. Takakura, Y. Iinuma, S. Ichiyama, and M. Mishima, J. Clin. Microbiol., 2007, 45, 1440. CrossRef
65. D. J. Farrell, I. Morrissey, S. Bakker, and D. Felmingham, J. Antimicrob. Chemother., 2002, 50, Suppl. S1, 39. CrossRef
66. a) F. Schlunzen, R. Zarivach, J. Harms, A. Bashan, A. Tocilj, R. Albrecht, A. Yonath, and F. Franceschi, Nature, 2001, 413, 814; CrossRef b) J. L. Hansen, J. A. Ippolito, N. Ban, P. Nissen, P. B. Moore, and T. A. Steitz, Molecular Cell, 2002, 10, 117. CrossRef
67. J. Kadota, The 52nd Annual Meeting of Japanese Society of Chemotherapy, 2004. 6. 3-4, Okinawa.
68. D. J. Farrell, S. G. Jenkins, S. D. Brown, M. Patel, B. S. Lavin, and K. P. Klugman, Emerging Infectious Diseases, 2005, 11, 851. CrossRef
69. T. Asaka, M. Kashimura, Y. Misawa, T. Ono, K. Suzuki, H. Yoshida, T. Yoshida, T. Akashi, C. Yokoo, T. Nagate, and S. Morimoto, The 35th Intersci. Conf. Antimicrob. Agents Chemother. (ICAAC), (Sep. 17-20, 1995, San Francisco), Abstract No. F-176.
70. C. Agouridas, Y. Benedetti, A. Denis, O. L. Martret, and J. F. Chantot, The 35th ICAAC, Abstract No. F-157.
71. A. Denis, C. Agouridas, J. M. Auger, Y. Benedetti, A. Bonnefoy, F. Bretin, J. F. Chantot, A. Dussarat, C. Fromentin, S. G. D’Ambrieres, S. Lachaud, P. Laurin, O. L. Martret, V. Loyau, N. Tessot, J. M. Pejac, and S. Perron, Bioorg. Med. Chem. Lett., 1999, 9, 3075. CrossRef
72. M. Kashimura, T. Asaka, Y. Misawa, K. Matsumoto, and S. Morimoto, J. Antibiot., 2001, 54, 664. CrossRef
73. C. Agouridas, A. Denis, J.-M. Auger, Y. Benedetti, A. Bonnefoy, F. Bretin, J.-F. Chantot, A. Dussarat, C. Fromentin, S. G. D’Ambrieres, S. Lachaud, P. Laurin, O. L. Martret, V. Loyau, and N. Tessot, J. Med. Chem., 1998, 41, 4080. CrossRef
74. Y. S. Or, R. F. Clark, S. Wang, D. T. W. Chu, A. M. Nilius, R. K. Flamm, M. Mitten, P. Ewing, J. Alder, and Z. Ma, J. Med. Chem., 2000, 43, 1045. CrossRef
75. a) C.-H. Liang, J. Duffield, A. Romero, Y.-H. Chiu, D. Rabuka, S. Yao, S. Sucheck, K. Marby, Y.-K. Shue, Yoshi Ichikawa, and C.-K. Hwang (Optimer Pharmaceuticals, Inc.), WO 2004/080391, Sep. 23, 2004; b) P. Fernandes, D. Pereira, B. Jamieson, and K. Keedy, Drugs of the Future, 2011, 36, 751.
76. a) R. Berisio, J. Harms, F. Schluenzen, R. Zarivach, H. A. S. Hansen, P. Fucini, and A. Yonath, J. Bacteriol., 2003, 185, 4276; CrossRef b) F. Schluenzen, J. M. Harms, F. Franceschi, H. A. S. Hansen, H. Bartels, R. Zarivach, and A. Yonath, Structure, 2003, 11, 329; CrossRef c) D. Tu, G. Blaha, P. B. Moore, and T. A. Steitz, Cell, 2005, 121, 257. CrossRef
77. a) B. M. Psaty, Clinical Infectious Diseases, 2008, 47, S176; CrossRef b) Y. Hatanaka, Y. Zamami, T. Koyama, N. Hobara, X. Jin, Y. Kitamura, and H. Kawasaki, Br. J. Pharmacol., 2008, 155, 826; CrossRef c) D. Bertrand, S. Bertrand, E. Neveu, and P. Fernandes, Antimicrob. Agents Chemother., 2010, 54, 5399; CrossRef d) C.-N. Liu and C. J. Somps, Toxicol. Lett., 2010, 194, 66. CrossRef
78. F. Traunmuller, M. Zeitlinger, P. Zeleny, M. Muller, and C. Joukhadar, Antimicrob. Agents Chemother., 2007, 51, 3185. CrossRef
79. T. Tanikawa, T. Asaka, M. Kashimura, Y. Misawa, K. Suzuki, M. Sato, K. Kameo, S. Morimoto, and A. Nishida, J. Med. Chem., 2001, 44, 4027. CrossRef
80. G. Bertho, J. G. Benarous, M. Delaforge, C. Lang, A. Parent, and J. P. Girault, J. Med. Chem., 1998, 41, 3373. CrossRef
81. R. Lakhmiri, P. Lhoste, and D. Sinou, Tetrahedron Lett., 1989, 30, 466. CrossRef
82. W. A. Herrmann, C. Brossmer, C.-P. Reisinger, T. H. Riermeier, K. Ofele, and M. Beller, Chem. Eur. J., 1997, 3, 1357. CrossRef
83. T. Furuuchi, T. Miura, K. Kurihara, T. Yoshida, T. Watanabe, and K. Ajito, Bioorg. Med. Chem., 2008, 16, 4401. CrossRef
84. Unpublished communication from Dr. Takenuki.
85. The term “western hemisphere” expresses left hand side of a macrolactone. It roughly indicates the C-10 to C-13 positions in 14-membered macrolides and the C-10 to C-15 positions in 16-membered macrolides. This expression (not only western hemisphere but northern or southern hemisphere) can be used for other chemical classes. See, a) A. B. Smith, III, H. Smits, and D.-S. Kim, Tetrahedron, 2010, 66, 6597; CrossRef b) X. Wang, T. J. Paxton, N. Li, and A. B. Smith, III, Org. Lett., 2012, 14, 3998. CrossRef
86. Research programs introduced in sections 2.4 to 2.6 and 3.1 to 3.7 were performed by the following members. Project leader: Mr. T. Miura. Synthetic chemistry leader: Dr. K. Kurihara then Mr. T. Miura. Biology leader: Dr. T. Yoshida then Dr. H. Fushimi. Synthetic chemistry: Dr. T. Furuuchi, Mr. K. Kanemoto, Miss S. Natsume (Mrs. Masaki), Mr. N. Ohkura, Miss Y. Yanagisawa (Mrs. Fujihira). Computational chemistry: Mr. T. Watanabe. Biology: Mr. Y. Takayama, Mr. K. Yamamoto, Mr. T. Matsuhira, Miss M. Watanabe (Mrs. Kumura), Miss N. Oonara. Pharmacokinetics: Mrs. H. Takata, Mr. K. Kijima. Analytical chemistry: Mrs. T. Miyara, Miss S. Miki, Mrs. K. Suzuki. Strategy & Planning: Dr. A. Tamura. Intellectual Property: Miss K. Yasufuku, Mrs. K. Kobayashi, and Mrs. E. Okazaki. Manuscript: Mrs. Y. Saito. English: Mrs. M. Takagi. Mr. Miura received his Ph.D. title with these works supervised by Professor Susumu Kobayashi from Tokyo University of Science in 2008.
87. L. H. Hansen, P. Mauvais, and S. Douthwaite, Mol. Microbiol., 1999, 31, 623. CrossRef
88. M. Muroi and T. Kishi, Chem. Pharm. Bull., 1978, 26, 2718. CrossRef
89. Tomoaki Miura, Dissertation, Tokyo University of Science (2008), see: pp. 79-80.
90. T. Miura, K. Kurihara, T. Furuuchi, T. Yoshida, and K. Ajito, Bioorg. Med. Chem., 2008, 16, 3985. CrossRef
91. K. Kurihara, T. Miura, N. Ohkura, T. Yoshida, T. Furuuchi, and K. Ajito (Meiji Seika Kaisha, Ltd.), WO 2002/064607, Aug. 22, 2002.
92. K. Kurihara, T. Miura, N. Ohkura, T. Furuuchi, Y. Fujihira, T. Yoshida, H. Fushimi, and K. Ajito (Meiji Seika Kaisha, Ltd.), WO 2006/073172 A1, Jul. 13, 2006.
93. S. Mutak, J. Antibiot., 2007, 60, 85 and cited there in. CrossRef
94. S. T. Waddell and T. A. Blizzard (Merck), WO 94/15617, Jul. 21, 1994.
95. A. B. Jones, J. Org. Chem., 1992, 57, 4361. CrossRef
96. K. Shankaran and R. R. Wilkening (Merck), EP 0549040 A1 (1993), Jun. 30, 1993.
97. G. Lazarevski, G. Kobrehel, and Z. Kelneric (Pliva), WO 99/51616, Oct. 14, 1999.
98. S. T. Waddell and T. A. Blizzard, Tetrahedron Lett., 1993, 34, 5385. CrossRef
99. N. Lopotar and S. Djokić (Pliva), EP 0410433 B1 (1996), Feb. 28, 1996.
100. T. Asaka, A. Manaka, T. Tanikawa, T. Sugimoto, Y. Shimazaki, and M. Sato (Taisho Pharmaceutical), WO 2003/014136 A1, Feb. 20, 2003.
101. T. Sugimoto, T. Tanikawa, K. Suzuki, and Y. Yamasaki, Bioorg. Med. Chem., 2012, 20, 5787. CrossRef
102. Z. M. Istuk, A. Cikos, D. Gembarovski, G. Lazarevski, I. Dilovic, D. M-Calogovic, and G. Kragol, Bioorg. Med. Chem., 2011, 19, 556. CrossRef
103. a) K. C. Nicolaou, T. K. Chakraborty, A. D. Piscopio, N. Minowa, and P. Bartinato, J. Am. Chem. Soc., 1993, 115, 4419; CrossRef b) Z. Yang, Y. He, D. Vourloumis, H. Vallberg, and K. C. Nicolaou, Angew. Chem. Int. Ed. Engl., 1997, 36, 166. CrossRef
104. a) G. Butora, S. D. Goble, A. Pasternak, L. Yang, C. Zhou, and C. R. Moyes (Merck Sharp & Dohme), WO 2004/094371 A2 (2004), Nov. 4, 2004; b) X. Xu, J. Lu, R. Li, Z. Ge, Y. Dong, and Y. Hu, Synlett, 2004, 122; CrossRef c) G. F. Painter, P. J. Eldridge, and A. Falshaw, Bioorg. Med. Chem., 2004, 12, 225; CrossRef d) V. Bonnet, R. Duval, V. Tran, and C. Rabiller, Eur. J. Org. Chem., 2003, 24, 4810. CrossRef
105. S. Ōmura, K. Miyano, H. Matsubara, and A. Nakagawa, J. Med. Chem., 1982, 25, 271. CrossRef
106. G. Illuminati and L. Mandolini, Acc. Chem. Res., 1981, 14, 95. CrossRef
107. E. L. Eliel, S. H. Wilen, and L. N. Mander, P. 677, Chapter 11, Configuration and Conformation of Cyclic Molecules, Stereochemistry of Organic Compounds, 1994 by John Wiley & Sons, Inc.
108. Unpublished data calculated by Dr. Naofumi Nakayama at Conflex Corporation under supervision by Associate Professor Dr. Yasuharu Sakamoto at Riken. A part of these analyses was reported by K. Ajito in the 23rd French-Japanese Symposium of Medicinal and Fine Chemistry at Nagasaki on May 13, 2013.
109. T. Miura, S. Natsume, K. Kanemoto, K. Atsumi, H. Fushimi, H. Sasai, T. Arai, T. Yoshida, and K. Ajito, J. Antibiot., 2007, 60, 407. CrossRef
110. H. Sasai, T. Arai, T. Miura, K. Atsumi, and K. Ajito (Meiji Seika Kaisha, Ltd.), WO 2005/007666 A1, Jan. 27, 2005.
111. D. Kuo, J. E. Leresche, R. Proplesch, J. P. Roduit, Y. Bessard, and E. Armbruster (Lonza), US 2005/0159458 A1, Jul. 21, 2005.
112. T. Miura, K. Kanemoto, S. Natsume, K. Atsumi, H. Fushimi, T. Yoshida, and K. Ajito, Bioorg. Med. Chem., 2008, 16, 10129. CrossRef
113. a) K. C. Nicolaou, K. Ajito, A. P. Patron, H. Khatuya, P. K. Richter, and P. Bertinato, J. Am. Chem. Soc., 1996, 118, 3059; CrossRef b) K. C. Nicolaou, A. P. Patron, K. Ajito, P. K. Richter, H. Khatuya, P. Bertinato, R. A. Miller, and M. J. Tomaszewski, Chem. Eur. J., 1996, 2, 847. CrossRef
114. K. C. Nicolaou, F. Roschangar, and D. Vourloumis, Angew. Chem. Int. Ed., 1998, 37, 2014. CrossRef
115. a) H. C. Kolb and K. B. Sharpless, Drug Discovery Today, 2003, 8, 1128; CrossRef b) T. Hirose, T. Sunazuka, Y. Noguchi, Y. Yamaguchi, H. Hanaki, K. B. Sharpless, and S. Ōmura, Heterocycles, 2006, 69, 55; CrossRef c) A. Sugawara, T. Sunazuka, T. Hirose, K. Nagai, Y. Yamaguchi, H. Hanaki, K. B. Sharpless, and S. Ōmura, Bioorg. Med. Chem. Lett., 2007, 17, 6340. CrossRef
116. FAB-MS m/z 144 (M+H)+ as C8H17NO; 1H NMR (300 MHz, CDCl3) δ 1.45 (m, 2-H), 1.57 (m, 3-H), 1.76 (m, 2-H), 2.02 (m, 4-H), 2.33 (dd, 3-CH2), 2.39 (s, NCH3), 2.65 (dd, 3-CH2), 3.46 (ddd, HOCH2), 3.65 (ddd, HOCH2), 5.00 (m, 1-H), 5.73 (m, 2-H).
117. I. Shiina, M. Kubota, and R. Ibuka, Tetrahedron Lett., 2002, 43, 7535. CrossRef
118. A. F. Littke and G. C. Fu, J. Am. Chem. Soc., 2001, 123, 6989. CrossRef
119. T. Miura, K. Kanemoto, S. Natsume, N. Ohkura, Y. Fujihira, T. Watanabe, H. Fushimi, K. Atsumi, and K. Ajito (Meiji Seika Kaisha, Ltd.), WO 2005/019238, Mar. 3, 2005.
120. Y. Li, S. J. Shaw, G. Ashley, and D. C. Myles, (Kosan Biosciences, Inc.), WO 2003/061671, Jul. 31, 2003.
121. T. Miura, S. Natsume, K. Kanemoto, E. Shitara, H. Fushimi, T. Yoshida, and K. Ajito, Bioorg. Med. Chem., 2010, 18, 2735. CrossRef
122. S. Omoto, K. Iwamatsu, S. Inouye, and T. Niida, J. Antibiot., 1976, 29, 536. CrossRef
123. T. Miura, K. Kanemoto, and K. Ajito, J. Synth. Org. Chem. Jpn., 2011, 69, 1339. CrossRef
124. a) M. Hikota, H. Tone, K. Horita, and O. Yonemitsu, J. Org. Chem., 1990, 55, 7; CrossRef b) M. Hikota, H. Tone, K. Horita, and O. Yonemitsu, Tetrahedron, 1990, 46, 4613; CrossRef c) T. Hamada, M. Hikota, O. Yonemitsu, E. Osawa, and H. Gotoh, Abstract, Symposium on the Chemistry of Natural Products, 1991, 33, pp. 313-320; d) N. Nakajima and O. Yonemitsu, Farumashia, 1991, 27, 25; e) (related reference) N. Nakajima, T. Matsushima, O. Yonemitsu, H. Gotô, and E. Osawa, Chem. Pharm. Bull., 1991, 39, 2819. CrossRef
125. Y. S. Or, G. Wang, L. T. Phan, D. Niu, N. H. Vo, Y. L. Qiu, Y. Wang, M. Busuyek, Y. Hou, Y. Peng, H. Kim, T. Liu, J. J. Farmer, and G. Xu, WO 03/097659 (2003).
126. T. Sunazuka, H. Shudo, K. Nagai, K. Yoshida, Y. Yamaguchi, H. Hanaki, and S. Ōmura, J. Antibiot., 2008, 61, 175. CrossRef
127. a) T. Sugimoto, K. Yamamoto, J. Kurosaka, N. Sasamoto, M. Kashimura, T. Miura, K. Kanemoto, T. Ozawa, K. Chikauchi, and E. Shitara (Taisho Pharmaceutical Co., Ltd., Meiji Seika Kaisha, Ltd.), WO 2009/019868 A1, Feb. 12, 2009; b) T. Sugimoto, K. Yamamoto, J. Kurosaka, N. Sasamoto, M. Kashimura, T. Miura, K. Kanemoto, S. Yoshida, K. Kumura, and K. Ajito (Taisho Pharmaceutical Co., Ltd., Meiji Seika Kaisha, Ltd.), WO 2009/129281 A1, Nov. 19, 2009.