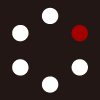
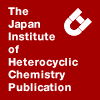
HETEROCYCLES
An International Journal for Reviews and Communications in Heterocyclic ChemistryWeb Edition ISSN: 1881-0942
Published online by The Japan Institute of Heterocyclic Chemistry
e-Journal
Full Text HTML
Received, 23rd October, 2014, Accepted, 19th January, 2015, Published online, 27th January, 2015.
DOI: 10.3987/REV-14-811
■ Synthesis of Triazole, Indole, and Five or Six-Membered Saturated Heterocyclic Compounds
Yasunari Monguchi,* Yoshinari Sawama, and Hironao Sajiki*
Laboratory of Organic Chemistry, Department of Pharmaceutical Sciences, Gifu Pharmaceutical University, 1-25-4 Daigakunishi, Gifu 501-1196, Japan
Abstract
Heterogeneously-catalyzed synthetic methods for the preparation of 1,2,3-triazoles, indolines, indoles, and saturated heterocyclic compounds, such as piperidine and pyrrolidine, are demonstrated. Triazoles are intermolecularly synthesized by the 1,3-dipolar cycloaddition of alkynes with azides using heterogeneous copper catalysts. Solvent-free triazole syntheses are also described. Inter- and intramolecular annulations as indole syntheses are summarized; e.g., a palladium on carbon (Pd/C)-catalyzed Yamanaka–Larock method affords 2- or 2,3-substituted indoles from 2-iodoaniline derivatives and mono- or di-substituted alkynes; an intramolecular Pd/C-catalyzed Buchwald–Hartwig reaction of 2-bromophenethylamine derivatives temperature-dependently affords indolines or indoles. Pd/C can also catalyze an intermolecular aromatic amination of bromoarenes with indoles to generate the corresponding N-arylindoles. The application of the hydrogenation technology using 10% Pd/C, 10% rhodium on carbon (Rh/C), or 10% ruthenium on carbon (Ru/C) as a catalyst is finally introduced for the synthesis of indole by the intramolecular hydrogenative N-alkylation of 2-cyanomethylaniline and piperidine, pyrrolidine, and tetrahydrofuran derivatives by the arene hydrogenation of pyrridine, pyrrole, and furan derivatives under relatively mild conditions, respectively.CONTENTS
1. Introduction
2. Copper-Catalyzed Heterogeneous Huisgen Cyclization for 1,2,3-Triazole Synthesis
2.1. Cu on Synthetic Adsorbent-Catalyzed Azide–Alkyne Cycloaddition
2.2. Cu on Chelate Resin-Catalyzed Azide–Alkyne Cycloaddition
3. Palladium on Carbon-Catalyzed Indole Synthesis
3.1. Yamanaka–Larock Indole Synthesis
3.2. N-Arylindole Synthesis by Intramolecular Aromatic Amination
4. Hydrogenation for the Synthesis of Heterocyclic Compounds
5. Conclusion
1. INTRODUCTION
Heterogeneous transition metal catalysts, such as palladium on carbon (Pd/C), have traditionally been used for the hydrogenation of a wide range of reducible functionalities including alkyne, alkene, and nitoro functionalities, and most of them are embedded on some insoluble supports, such as activated carbon, CaCO3, Al2O3, etc.1 Recently, the use of such heterogeneous transition metal catalysts for organic synthesis is demanded from the environmental and cost-efficient points of view based on their recoverability, reusability, and property of preventing contamination of the metal residue in the products.2 We have developed unique heterogeneous transition metal catalysts using industrially manufactured products3 as well as natural resources,4 such as a fibroin protein as a major component of raw silk, for the hydrogenation and cross-coupling reactions. Furthermore, novel synthetic methodologies for carbon–carbon,5 carbon–nitrogen,6 and carbon–oxygen7 bond forming reactions, hydrogen–deuterium exchange reactions,8 arene hydrogenation,9 hydrodehalogenation,10 and oxidation of hydrogen11 using conventional activated carbon-supported transition-metal catalysts, such as Pd/C,12 ruthenium on carbon (Ru/C), rhodium on carbon (Rh/C), and platinum on carbon (Pt/C), have been established. In this review, we focused on the heterogeneously catalyzed annulation as the 1,4-disubstituted 1,2,3-triazole13–15 and indole synthesis6a,6c,16,17 and an arene hydrogenation for the synthesis of saturated heterocyclic compounds.9b
2. COPPER-CATALYZED HETEROGENEOUS HUISGEN CYCLIZATION FOR 1,2,3-TRIAZOLE SYNTHESIS
The Huisgen cyclization of azides to alkynes is a powerful tool for the construction of the 1,2,3-triazole nucleus,18 which is recognized as one of the important structural units in bioactive compounds.19 In particular, the copper-catalyzed reaction using mono-substituted alkynes and azides for the regioselective synthesis of 1,4-di-substituted 1,2,3-triazoles independently developed by Sharpless20 and Meldal21 has been widely used for the preparation of a variety of functional materials because of its reliability, simplicity, and effectiveness.22 The current supports employed for the immobilized copper catalysts for the heterogeneous Huisgen cyclization are carbon materials,23 amine- or imino-bound silica,24 zeolite,25 superparamagnetic mesoporous silica,26 hydrotalcite,27 AlO(OH),28 metal oxides,29 polysaccharide,30 ligand-bound organic polymers,31 and ion exchange resins, such as Amberlyst A2132 and Dowex.33 The preparation of supported copper catalysts on these materials requires multiple steps in most cases and centrifugation or decantation for their separation from the reaction mixture in some cases.
2.1. Cu on Synthetic Adsorbent-Catalyzed Azide–Alkyne Cycloaddition13,14
Industrially produced materials should be good candidates as supports of immobilized metal catalysts due to their reliable qualities and stable supply. DIAION® HP20 (HP20, Mitsubishi Chemical Corporation), which is a commercially available polystyrene-polyvinylbenzene-based synthetic adsorbent for chromatographic separation, possesses a large surface area (ca. 590 m2/g) and fine pore structure (30 nm of radius) on the particle (440 µm of average diameter) and is stable in organic solvents and in acidic and basic media.34 These features encouraged us to use HP20 as a support for the heterogeneous transition-metal catalysts because of the possible efficient contact of organic substrates with active metal species on/in the organic adsorbent by a lipophilic enrichment effect. The palladium catalyst supported on HP20 was successfully prepared and used for the hydrogenation and cross-coupling reaction as expected.3a,3b
The copper species were simply embedded on HP20 according to the modified preparation procedure of Lipshutz's Cu/C.23a HP20 was immersed in an aqueous solution of Cu(NO3)2 and sonicated for 2 h. The resulting Cu/HP20 was filtered, washed with toluene, and dried. The immobilized copper spcies of Cu/HP20 was determined to be 1 weight % by measurement of the copper content in the filtrate and wash obtained during the catalyst preparation using an atomic absorption photometer (Scheme 1).13
1% Cu/HP20 (1 mol%) effectively catalyzed the cycloaddition of benzylazide (1a) with 3-methyl-1-butyn-3-ol (2a) in the presence of Et3N (1.1 equiv) to give the corresponding 1,4-disubstitued 1,2,3-triazole (3aa) in an excellent yield (Scheme 2).
The 1% Cu/HP20-catalyzed Huisgen cycloaddition exhibits a broad substrate applicability. 3-Methyl-1-butyn-3-ol (2a) smoothly reacted with various azides (1) at room temperature, although the cycloaddition of 3,5-dinitrophenylmethylazide (1b) to the triazole (3ba) required the application of heat for the complete consumption of 1b possibly because of its low solubility in toluene as an exception (Table 1). Such a problem could be solved by the solvent-free reaction as described below (Table 2). A wide range of alkynes (2) were also good substrates for the 1% Cu/HP20-catalyzed cycloaddition with 4-fluorobenzylazide (1c). Especially, the reaction of 2-ethynylpyridine (2e) was completed within 3 h probably because the nitrogen atom of the pyridine nucleus worked as a directing group of copper metal based on the coordination to facilitate its approach to the adjacent carbon-carbon triple bond.
The 1% Cu/HP20 could be reused until at least the 3rd run for the cycloaddition to 3ca without any significant loss of the catalyst activity, although a longer reaction time was required in the 4th and 5th runs to complete the reaction progress (from 5 h to 12 h and 24 h).
The elimination of solvents from organic reactions as well as the use of heterogeneous catalysts is recognized as one of the goals of green sustainable chemistry because it leads to the reduction of solvent wastes, size of the manufacturing plants, and cost.35,36 Only a couple of studies have reported the solvent-free heterogeneous Huisgen cycloaddition using copper oxide on acetylene carbon black23d and copper on Amberlyst A2132b as catalysts (Schemes 3 and 4). These reactions proceeded without any base. The former catalyst could be reused for at least five cycles, but microwave conditions and centrifuging were required. The reuse of the catalyst is not mentioned in the latter case.
The 1% Cu/HP20-catalyzed Huisgen cycloaddition could also be carried out in the presence of a catalytic amount of Et3N (0.22 equiv) under solvent-free conditions.14 A wide variety of azides (1) reacted with alkynes (2) at room temperature to give the corresponding triazoles (3) in good yields (Table 2). The huge advantage of the solvent-free conditions was to enhance the efficiency of the cyclization using the low soluble azide, 3,5-dinitrophenylmethylazide (1b), probably due to the substrates concentration effect on the lipophilic support, although the above-mentioned reaction in toluene in Table 1 never proceeded at room temperature and required heat at 60 °C for the completion. 2-Ethynylpyridine (2e) was also a good substrate for the preparation of 3ce under the solvent-free conditions.
The reuse test of 1% Cu/HP20 under the solvent-free conditions indicated that the reaction efficiency was significantly reduced after each run (1st run, 100%; 2nd run, 88%; 3rd run, 34%). Approximately 19% copper leaching from 1% Cu/HP20 during the reaction of 1c with 2a was observed by inductively coupled plasma atomic emission spectrometry (ICP-AES), thus the leaching of copper species would cause the problematic reuse of the 1% Cu/HP20 as a catalyst.
2.2. Cu on Chelate Resin-Catalyzed Azide–Alkyne Cycloaddition15
Chelate resins, DIAION CR11 (CR11) and DIAION CR20 (CR20), both produced by the Mitsubishi Chemical Corporation, polystyrene-divinylbenzene-based polymers possessing iminodiacetic acid and polyamine moieties as ligands, respectively, have been used for water purification by their capturing ability of heavy metal ions based on chelation.34a,37 These resins should also be guaranteed as industrial products in quality and properties under strictly controlled regulations.
Copper ions were immobilized on the CR11 and CR20 in an improved manner for the preparation for 1% Cu/HP20, by simply immersing CR11 and CR20 in an aqueous solution of Cu(NO3)2 but without sonication, and highly loaded copper catalysts, 12% Cu/CR11 and 7% Cu/CR20, were obtained by the effective complexation of copper ions with ligands on the polymers (Scheme 5).15
The 1,3-dipolar cycloaddition of benzylazide (1a) with ethynylbenzene (2d) was found to effectively proceed at 70 °C under solvent-free conditions in the presence of catalytic amounts of 12% Cu/CR11 (1–5 mol%) and Et3N (0.22 equiv), and the corresponding 1,2,3-triazole (3ad) was quantitatively obtained (Table 3). A wide range of azides (1) and alkynes (2) were good substrates for the solvent-free cycloaddition. Therefore, the 12% Cu/CR11-catalyzed Huisgen cycloaddition under the solvent-free conditions is of general use for the preparation of the 1,4-disubstituted 1,2,3-triazole (3).
The polyamino moieties on the support of 7% Cu/CR20 would also function as the base for the Huisgen cycloaddition; thus, benzylazide (1a) and ethynylbenzene (2d) successfully reacted at 70 °C in a solvent-free manner even in the absence of Et3N (Table 4). A variety of 1,4-disubstituted 1,2,3-triazoles (3) was synthesized under neat conditions at 70 °C using 7% Cu/CR20. Although linear alkyl substituted azides (1j) and alkynes (2h) were slightly less reactive as substrates, the reaction efficiencies were improved by increasing the catalyst amount and prolonged reaction time.
12% Cu/CR11 could be recovered and reused until the 4th run without any significant catalyst activity loss, but it decreased in the 5th run. The decreased catalyst activity would be caused by the leaching or split-off of copper species from the catalyst, since the disassociation of approximately 3.0% of the copper species from the 12% Cu/CR11 was detected by ICP-AES. On the other hand, the catalytic activity never decreased until at least the 5th run in the case of 7% Cu/CR20, and the desired triazole (3ad) was quantitatively obtained from each run.
In this section, the Huisgen cyclizations of azides with alkynes using heterogeneous copper catalysts are described. Especially, the solvent-free protocols would encourage the practical application for the synthesis of 1,4-disubstituted 1,2,3-triazoles in the process chemistry field. The next section will introduce the heterogeneous palladium-catalyzed synthesis of indoles and indoline, which are an important structural unit for biologically active compounds, in the inter- or intramolecular cyclization manner.
3. PALLADIUM ON CARBON-CATALYZED INDOLE SYNTHESIS
The indole framework is one of the most ubiquitous heterocyclic nuclei found in the chemical structure of biologically active compounds, such as serotonine, indometacin, sertindole, etc.38 Various methods for the construction of the indole nucleus have been developed.39
3.1. Yamanaka–Larock Indole Synthesis16
The one-pot direct synthesis of 2-substituted indoles from 2-haloanilines and mono-substituted alkynes by the Sonogashira reaction and the following cyclization in the presence of Pd(Ph3P)2Cl2, CuI, and Et3N was first reported by Yamanaka et al.,40 while Larock employed disubstituted alkynes with Pd(OAc)2, LiCl, and K2CO3 to obtain 2,3-disubstituted indoles in a highly regioselective manner (Scheme 6).41 There are a few reports of the Yamanaka-type 2-substututed indole syntheses from mono-substituted alkynes using not commercially available heterogeneous palladium catalysts42,43 or palladium-copper combined catalysts.44 The Pd/C-catalyzed Yamanaka-type annulation was also developed, but the combined use with triphenylphosphine as a ligand, CuI, and 2-aminoethanol was necessary (Scheme 7).45 Djakovitch et al. reported the LiCl-free Larock-type annulation for the 2,3-disubstituted indole synthesis using heterogeneous palladium species including Pd/C (Scheme 8)46 during the preparation of our paper for the Pd/C-catalyzed Yamanaka–Larock indole synthesis.16
Our initial optimal conditions are shown in Scheme 9. The 10% Pd/C-catalyzed annulation between N-tosyl-2-iodoaniline (4a) and 6-dodecyne (5a) led to the quantitative formation of N-tosyl-2,3-dipentylindole (6aa) using an equimolar amount of LiCl and 5 equiv of NaOAc in heated N-methylpyrrolidone (NMP) (120 °C).
A wide range of alkynes (5: 3 equiv) could be used for the preparation of the indole derivatives from N-tosyl-2-phenylindole (4a) under the above-mentioned optimal conditions (Table 5). The reactions of symmetrical dialkylacetylenes gave the corresponding 2,3-disubstituted indoles [6aa (Scheme 9), 6ab, 6ac, and 6ad (Table 5)] in good to quantitative yields, regardless of the alkyl chain length of 5. Unsymmetrical disubstituted acetylenes, such as 1-phenyl-1-hexyne (5e) and 1-phenyl-1-propyne (5f), were also smoothly cyclized, but complete regioselectivities could not be achieved. The cyclization of ethynylbenzene (5g), a mono-substituted acetylene, proceeded in a complete regioselective manner to give N-tosyl-2-phenylindole (6ag) as the sole product, while the use of 1-hexyne (5h) as a substrate gave a mixture of regioisomers, i.e., the 2- and 3-butyl-1-tosylindoles (6ah and 7ah) in the ratio of 89 : 11, respectively (Scheme 10). These results indicated that the pathway for the indole formation from mono-substituted alkynes would be the same as the proposed mechanism for the synthesis of 2,3-disubstituted indoles from disubstituted alkynes reported by Larock, which involves the insertion of an alkyne into the arene–palladium bond to form the vinylic palladium intermediate,41 although the two-step process including the Sonogashira-type coupling could not be thoroughly excluded (Scheme 11).40 The Yamanaka–Larock indole synthesis requires no use of LiCl as does the Djakovitch's protocol (see the synthesis of 6ac, 6ad, 6ae, 6af, and 6ag in Table 5).46
The optimal conditions for the LiCl-free 10% Pd/C-catalyzed Yamanaka–Larock indole synthesis using N-tosyl-2-iodoaniline (4) and alkyne (5) derivatives as substrates are summarized in the equation of Table 6. Various 2,3-di- and 2-monosubstituted indoles were successfully synthesized from alkynes (1.1 equiv) (6) and N-tosyl-2-iodoaniline derivatives (4) in the presence of 10% Pd/C (3 mol%) and NaOAc (1.1 equiv) in heated NMP (120 °C). Symmetrical alkynes (5a, 5i, 5c, and 5d) were effectively reacted with 4a to give the corresponding 2,3-disubstituted indoles (6aa, 6ai, 6ac, and 6ad) in excellent yields. Phenylacetylenes bearing a methoxy (5j) or methyl (5k and 5l) functionality on the benzene ring cyclized to the corresponding 2-arylindoles (6aj, 6ak, and 6al) in a completely regioselective manner. The reaction of the 1-naphthyl- (5m), 2-naphthyl- (5n) and 1-cyclohexenyl (5o) acetylene derivatives also gave the desired 2-naphth-1-yl- (6am), 2-naphth-2-yl- (6an), and 2-cyclohexenylindole (6ao) derivatives
in practically applicable yields. Furthermore, N-tosyl-2-iodo-4-methoxycarbonylaniline (4b) was also a good substrate. The distinctive features of the protocol are typically for both the Yamanaka- and Larock-type reactions and a high efficiency with lower amounts of alkyne and base.16
A practical, efficient, and general protocol for the 10% Pd/C-catalyzed Yamanaka–Larock indole synthesis in the absence of LiCl, was described. Symmetrical disubstituted alkynes reacted with N-tosyl-2-iodoaniline to give the corresponding 2,3-disubstituted indoles in excellent yields, and a variety of 2-mono-arylsubstituted indole derivatives were synthesized from the mono-arylalkynes in a completely regioselective manner. Easy deprotection of the N-tosyl group on the indole nucleus47 is also an advantage of this protocol. The next section will introduce the heterogeneously catalyzed intramolecular cyclization for the syntheses of indole and dihydroindole (indoline) derivatives.
3.2. N-Arylindole Synthesis by Intramolecular Aromatic Amination17
Scheme 12. Pd-catalyzed intramolecular aromatic amination of 2-bromophenthylamine derivative (8)Scheme 13. One-pot synthesis of phenylindoline (13) via consecutive intra- and intermolecular aromaic aminations2-Halophenethylamines have been used as the starting materials for construction of the indoline skeleton, which is readily found as a core structural unit of biologically active compounds, by the cyclization based on the copper-,48 copper-iron-,49 nickel-,50 or palladium-catalyzed51,52 intramolecular carbon–nitrogen bond forming reaction. Tocher et al. reported that the intramolecular cyclization of a 2-bromophenethylamine derivative (8) using Pd(PPh3)4 as a catalyst afforded the desired 2-substituted indoline derivative (9) together with decomposed product, indole (10a) (Scheme 12).51a 2-Chlorophenethylamine (11) was also applied by Schneider and Fort et al. for the one-pot synthesis of
N-arylindoline (13) by the intramolecular annulation using Pd(OAc)2 and active N-heterocyclic carbene and subsequent N-arylation of the resulting indoline (12a) with chloroarene (Scheme 13).51b
The aromatization of indoline to the indole was achieved by a variety of oxidation methods.53 Dehydrogenation reactions using heterogeneous transition metal catalysts, such as polymer-incarcerated gold,54 gold on graphite,55 ruthenium on alumina,56 palladium on hydroxyapatite (PdHAP),57 and palladium on carbon (Pd/C) (Scheme 14)58 were also reported in the literature, although the direct or one-pot synthesis of the indole from 2-halophenethylamine has scarcely been reported.59
We have recently developed a Pd/C-catalyzed Buchwald–Hartwig cross-coupling reaction between amines and bromoarenes forming a carbon–nitrogen bond with a very low level of palladium leaching.6b 2-Bromophenethylamine (14a) was used for the intramolecular Buchwald–Hartwig aromatic amination in the presence of 10% Pd/C (2 mol%), 1,1'-bis(diphenylphosphino)ferrocene (DPPF, 3 mol%), and NaOt-Bu (2–2.5 equiv) in mesitylene. The cyclization temperature-dependently gave different products; i.e., indoline (12a) at 140 °C and indole (10a) at 200 °C, both in 95% yields (Scheme 15). The amount of NaOt-Bu is also an important factor for this selectivity, and a 64 : 36 mixture of 12a and 10a was
obtained by using 2 equiv of NaOt-Bu even at 140 °C. These results indicated that the strongly basic conditions should be unfavorable for the aromatization of the indoline (12a) to indole (10a). Crabtree et al. achieved the Pd/C-catalyzed aromatization of 12a in refluxing toluene under neutral conditions (Scheme 14).58
Methyl-substituted indolines (12b and 12c) were synthesized under the same conditions indicated for the synthesis of 12a (Scheme 15 and Table 7, Entry 1) using 10% Pd/C (2 mol%), DPPF (3 mol%), and NaOt-Bu (2.5 equiv) in mesitylene at 140 °C (Table 7, Entries 3 and 5). Since the aromatization was not completed at 200 °C in the case of the methylated 2-bromophenethylamine derivatives (14b and 14c) as substrates, 1.5 equiv of acetic acid was added to neutralize the reaction media after completion of the indoline formation (12b and 12c). Methylated indoles (10b and 10c) as well as non-substituted indole (10a) were selectively obtained in good yields by the addition of acetic acid after further stirring at 140 °C without raising the temperature for the aromatization (Entries 2, 4, and 6). Furthermore, 5-methoxyindole (10d) was also obtained from 2-bromo-5-methoxyphenethylamine (14d) by the minor tuning of the reaction conditions (Entry 7).
N-Arylindole derivatives are also expected to be potential bioactive compounds; e.g., sertindole has an antipsychotic activity.38b Although the introduction of the aryl function to the N-1 position of the indole nucleus has been achieved by the aromatic amination of aryl halides using palladium catalysts,43,60,61 only a few heterogeneously catalyzed N-arylation reactions for indoles have been reported in the literature. Djakovitch et al. achieved the heterogeneous palladium-catalyzed synthesis of N-arylated 2-phenylindole (15) by the cross-coupling between 2-phenylindole (10e) and 4-iodonitrobenzene (Scheme 16).43
Pd/C could catalyze the direct introduction of aryl groups into the N-1 position of the indole derivatives (10) under the conditions similar to those for the intramolecular aromatic amination of the 2-bromophenethylamines (14) (Table 8). N-Phenylindole and its derivatives (16aa, 16ab, 16af, 16ba, 16da, and 16ea) were obtained in excellent yields from the N-1 free indole derivatives (10a, 10b, 10d, and 10e) and aryl bromides. Although the alternative use of Cs2CO3 instead of NaOt-Bu was necessary
for the effective progress of the N-arylation of 10a with bromobenzene derivatives bearing an electron-withdrawing group,6b the corresponding N-arylindoles (16ac, 16ad, and 16ae) were generated in good yields. Bromopyridine derivatives as well as 2-bromoquinoline were also good substrates regardless of the substitution position of the bromine on the pyridine nucleus (16ag, 16ah, and 16ai).
A one-pot synthesis, which is a synthetic methodology for the multistep reaction conducted in one reaction vessel, is generally desired from the viewpoints of time-, effort-, cost-, and waste-reduction based on the avoidance of several purification and separation processes of the intermediates. The N-arylindoles (16) could be satisfactorily synthesized in a one-pot manner from 2-bromophenethylamine (14a) via the consecutive Pd/C-catalyzed intramolecular aromatic amination and aromatization at 200 °C, and subsequent N-arylation of the resulting indole with bromoarenes at 180 °C (Table 9).
Both the indoline and indole derivatives could be temperature-dependently and distinctively synthesized from 2-bromophenethylamine under the Pd/C-catalyzed aromatic amination conditions. The pH control is an important factor for the Pd/C-catalyzed aromatization of indolines to indoles; the neutralization of the reaction media by the addition of acetic acid after the formation of indoline derivatives was effective for their aromatization to the corresponding indoles. Pd/C was found to be an effective heterogeneous catalyst for the intermolecular cross-coupling between the indole derivatives and bromoarenes to synthesis the N-arylated indoles.
In this section, both indole syntheses via the annulation and arene introduction to the N-1 position of indoles catalyzed by heterogeneous palladium catalysts were demonstrated. In the next section, the application of hydrogenation conditions using carbon-supported transition-metal catalysts to the synthesis of heterocyclic compounds is described.
4. HYDROGENATION FOR THE SYNTHESIS OF HETEROCYCLIC COMPOUNDS
We recently reported that 10% Pd/C catalyzed either the dialkylation of primary alkylamines to the corresponding tertiary amines or monoalkylation to the corresponding secondary amines when using nitriles as the alkylating reagents under hydrogenation conditions. Furthermore, the use of 5% Rh/C instead of 10% Pd/C for the alkylation of primary alkylamines with nitriles leads to the selective synthesis of dialkylamines (Scheme 17).6a,6c
The nitrile-mediated N-monoalkylation of arylamines could be used for the intramolecular alkylation of 2-cyanomethylaniline (17). The reaction of 17 smoothly proceeded under Pd/C-catalyzed hydrogenation conditions at room temperature to quantitatively give the corresponding indole (10a) (Scheme 18).6a,6c This method will be applied to the synthesis of various kinds of indole derivatives.
Arene hydrogenation is one of the most fascinating methods to access the saturated cyclic compounds bearing substituents because a variety of excellent methods for the introduction of substituents into aromatic rings rather than alicycles has been developed. However, arene hydrogenation typically requires harsh reaction conditions, such as a high hydrogen pressure and temperature, due to the resonance stabilization of arene nucleus.1 We recently developed three protocols for the arene hydrogenation under relatively mild conditions (< 10 atm) using 10% Rh/C, H2, in H2O at 80 °C (protocol A), 10% Rh/C, H2, in i-PrOH at 60 °C (protocol B), or 10% Ru/C, H2, in i-PrOH at 60–120 °C (protocol C) (Scheme 19).9b Protocol A is most environmentally benign due to the use of water as the solvent, while protocol B was found to be the most efficient. Protocol C is an economically friendly procedure due to the use of the low-cost Ru/C. Therefore, these protocols could be appropriately selected taking into account the environmental burden, reactivity of substrates, and cost.
The protocols could be used for the hydrogenation of heteroarenes. Pyridine, pyrrole, and furan derivatives could undergo the hydrogenation under the mild 10% Rh/C- or 10% Ru/C-catalyzed hydrogenation conditions (protocol A–C) to afford the corresponding piperidine (18 and 19), pyrrolidine (20), and tetrahydrofuran (21) derivatives, respectively, in excellent yields (Figure 1).
5. CONCLUSION
This review summarized the heterogeneously catalyzed syntheses of 1,4-disubstituted 1,2,3-triazoles by Huisgen cycloaddition and indoles by the Yamanaka–Larock annulation and intramolecular Buchwald–Hartwig aromatic amination. The copper catalysts embedded in an organic polymer could be used for the Huisgen cycloaddition due to the effective adsorption of organic substrates into the polymer support based on its lipophilic nature; the solvent-free Huisgen cycloaddition proceeds using chelate resin-supported copper catalysts. The intra- and intermolecular annulations for the construction of indole nuclei are efficiently catalyzed by Pd/C. In the last part, the application of the activated carbon-supported transition-metal catalyzed hydrogenation conditions was introduced; 1) indole is synthesized by the 10% Pd/C-catalyzed intramolecular reductive alkylation of 2-cyanomethylaniline, 2) saturated heteroaromatic compounds, piperidine, pyrrolidine, and tetrahydrofuran derivatives, are obtained by the 10% Rh/C- or 10% Ru/C-catalyzed arene hydrogenation. The heterogeneous transition-metal catalysts would be more widely used for the synthesis of heterocyclic compounds due to their advantages, such as their good recovery, reuse, and decreased metal-contamination risk.
ACKNOWLEDGEMENTS
We sincerely thank the N.E. Chemcat Corporation for the kind gifts of 10% Pd/C and Pd(OAc)2 and ICP-AES measurements and the Mitsubishi Chemical Corporation for the kind gifts of DIAION HP20, CR11, and CR20. We also thank all current and previous members in our laboratory for their hard work and thoughtful discussion.
References
1. .
2. (a) L. Yin and J. Liebscher, Chem. Rev., 2007, 107, 133; CrossRef (b) M. J. Climent, A. Corma, and S. Iborra, Chem. Rev., 2011, 111, 1072. CrossRef
3. (a) Y. Monguchi, Y. Fujita, K. Endo, S. Takao, M. Yoshimura, Y. Takagi, T. Maegawa, and H. Sajiki, Chem. Eur. J., 2009, 15, 834; CrossRef (b) Y. Monguchi, K. Sakai, K. Endo, Y. Fujita, M. Niimura, M. Yoshimura, T. Mizusaki, Y. Sawama, and H. Sajiki, ChemCatChem., 2012, 4, 546; CrossRef (c) T. Maegawa, T. Takahashi, M. Yoshimura, H. Suzuka, Y. Monguchi, and H. Sajiki, Adv. Synth. Catal., 2009, 351, 2091; CrossRef (d) T. Takahashi, M. Yoshimura, H. Suzuka, T. Maegawa, Y. Sawama, Y. Monguchi, and H. Sajiki, Tetrahedron, 2012, 68, 8293; CrossRef (e) H. Sajiki, S. Mori, T. Ohkubo, T. Ikawa, A. Kume, T. Maegawa, and Y. Monguchi, Chem. Eur. J., 2008, 14, 5109; CrossRef (f) S. Mori, T. Ohkubo, T. Ikawa, A. Kume, T. Maegawa, Y. Monguchi, and H. Sajiki J. Mol. Catal. A, 2009, 307, 77. CrossRef
4. (a) H. Sajiki, T. Ikawa, H. Yamada, K. Tsubouchi, and K. Hirota, Tetrahedron Lett., 2003, 44, 171; CrossRef (b) H. Sajiki, T. Ikawa, and K. Hirota, Tetrahedron Lett., 2003, 44, 8437; CrossRef (c) T. Ikawa, H. Sajiki, and K. Hirota, Tetrahedron, 2005, 61, 2217; CrossRef (d) Y. Kitamura, A. Tanaka, M. Sato, K. Oono, T. Ikawa, T. Maegawa, Y. Monguchi, and H. Sajiki, Synth. Commun., 2007, 37, 4381. CrossRef
5. (a) H. Sajiki, T. Kurita, A. Kozaki, G. Zhang, Y. Kitamura, T. Maegawa, and K. Hirota, J. Chem. Res., 2004, 593 [Erraturn: J. Chem. Res., 2005, 344]; (b) H. Sajiki, T. Kurita, A. Kozaki, G. Zhang, Y. Kitamura, T. Maegawa, and K. Hirota, Synthesis, 2005, 537 [Erraturn: Synthesis, 2005, 852]; (c) H. Sajiki, G. Zhang, Y. Kitamura, T. Maegawa, and K. Hirota, Synlett, 2005, 619 [Erraturn: Synlett, 2005, 1046]; (d) T. Maegawa, Y. Kitamura, S. Sako, T. Udzu, A. Sakurai, A. Tanaka, Y. Kobayashi, K. Endo, U. Bora, T. Kurita, A. Kozaki, Y. Monguchi, and H. Sajiki, Chem. Eur. J., 2007, 13, 5937; CrossRef (e) Y. Kitamura, A. Sakurai, T. Udzu, T. Maegawa, Y. Monguchi, and H. Sajiki, Tetrahedron, 2007, 63, 10596; CrossRef (f) Y. Kitamura, S. Sako, T. Udzu, A. Tsutui, T. Maegawa, Y. Monguchi, and H. Sajiki, Chem. Commun., 2007, 5069; CrossRef (g) S. Mori, T. Yanase, S. Aoyagi, Y. Monguchi, T. Maegawa, and H. Sajiki, Chem. Eur. J., 2008, 14, 6994; CrossRef (h) Y. Kitamura, S. Sako, A. Tsutui, Y. Monguchi, T. Maegawa, Y. Kitade, and H. Sajiki, Adv. Synth. Catal., 2010, 352, 718; CrossRef (i) Y. Yabe, T. Maegawa, Y. Monguchi, and H. Sajiki, Tetrahedron, 2010, 66, 8654; CrossRef (j) T. Yanase, S. Mori, Y. Monguchi, and H. Sajiki, Chem. Lett., 2011, 40, 910; CrossRef (k) T. Yanase, Y. Monguchi, and H. Sajiki, RSC Advances, 2012, 2, 590; (l) Y. Monguchi, T. Hattori, Y. Miyamoto, T. Yanase, Y. Sawama, and H. Sajiki, Adv. Synth. Catal., 2012, 354, 2561; CrossRef (m) Y. Monguchi, T. Yanase, S. Mori, and H. Sajiki, Synthesis, 2013, 45, 40. CrossRef
6. (a) H. Sajiki, T. Ikawa, and K. Hirota, Org. Lett., 2004, 6, 4977; CrossRef (b) Y. Monguchi, K. Kitamoto, T. Ikawa, T. Maegawa, and H. Sajiki, Adv. Synth. Catal., 2008, 350, 2767; CrossRef (c) T. Ikawa, Y. Fujita, T. Mizusaki, S. Betsuin, H. Takamatsu, T. Maegawa, Y. Monguchi, and H. Sajiki, Org. Biomol. Chem., 2012, 10, 293.
7. (a) Y. Monguchi, T. Takahashi, Y. Iida, Y. Fujiwara, Y. Inagaki, T. Maegawa, and H. Sajiki, Synlett, 2008, 2291; (b) S. Mori, M. Takubo, T. Yanase, T. Maegawa, Y. Monguchi, and H. Sajiki, Adv. Synth. Catal., 2010, 352, 1630; CrossRef (c) Y. Sawama, M. Takubo, S. Mori, Y. Monguchi, and H. Sajiki, Eur. J. Org. Chem., 2011, 3361. CrossRef
8. For review: (a) H. Esaki, T. Kurita, Y. Fujiwara, T. Maegawa, Y. Monguchi, and H. Sajiki, J. Syn. Org. Chem. Jpn., 2007, 65, 1179; CrossRef (b) Y. Sawama, Y. Monguchi, and H. Sajiki, Synlett, 2012, 23, 959. CrossRef
9. (a) T. Maegawa, A. Akashi, H. Esaki, F. Aoki, H. Sajiki, and K. Hirota, Synlett., 2005, 5, 845; (b) T. Maegawa, A. Akashi, K. Yaguchi, Y. Iwasaki, M. Shigetsura, Y. Monguchi, and H. Sajiki, Chem. Eur. J., 2009, 15, 6953. CrossRef
10. (a) H. Sajiki, A. Kume, K. Hattori, and K. Hirota, Tetrahedron Lett., 2002, 43, 7247; CrossRef (b) Y. Monguchi, A. Kume, K. Hattori, T. Maegawa, and H. Sajiki, Tetrahedron, 2006, 62, 7926; CrossRef (c) H. Sajiki, A. Kume, K. Hattori, H. Nagase, and K. Hirota, Tetrahedron Lett., 2002, 43, 7251; CrossRef (d) A. Kume, Y. Monguchi, K. Hattori, H. Nagase, and H. Sajiki, Appl. Catal. B, 2008, 81, 274; CrossRef (e) Y. Monguchi, A. Kume, and H. Sajiki, Tetrahedron, 2006, 62, 8384; CrossRef (f) Y. Monguchi, S. Ishihara, A. Ido, M. Niikawa, K. Kamiya, Y. Sawama, H. Nagase, and H. Sajiki, Org. Process Res. Dev., 2010, 14, 1140; CrossRef (g) A. Ido, S. Ishihara, A. Kume, T. Nakanishi, Y. Monguchi, H. Sajiki, and H. Nagase, Chemosphere, 2013, 90, 57; CrossRef (h) S. Ishihara, A. Ido, Y. Monguchi, H. Nagase, and H. Sajiki, J. Hazard. Mater., 2012, 229–230, 15; CrossRef (i) Y. Monguchi, A. Ido, M. Niikawa, N. Nagatsu, R. Mizukoshi, H. Nagase, and H. Sajiki, Heterocycles, 2015, 90, 186; CrossRef (j) T. Imanishi, Y. Fujiwara, Y. Sawama, Y. Monguchi, and H. Sajiki, Adv. Synth. Catal., 2012, 354, 771; CrossRef (k) Y. Sawama, Y. Yabe, M. Shigetsura, T. Yamada, S. Nagata, Y. Fujiwara, T. Maegawa, Y. Monguchi, and H. Sajiki, Adv. Synth. Catal., 2012, 354, 777; CrossRef (l) Y. Sawama, T. Imanishi, R. Nakatani, Y. Fujiwara Y. Monguchi, and H. Sajiki, Tetrahedron, 2014, 70, 4540. CrossRef
11. Y. Monguchi, T. Ida, T. Maejima, T. Yanase, Y. Sawama, Y. Sasai, S.-i. Kondo, and H. Sajiki, Adv., Synth., Catal., 2014, 356, 313. CrossRef
12. (a) M. Seki, J. Syn. Org. Chem. Jpn., 2006, 64, 853; CrossRef (b) M. Seki, Synthesis, 2006, 2975; CrossRef (c) F.-X. Felpin, T. Ayad, and S. Mitra, Eur. J. Org. Chem., 2006, 2679; CrossRef (d) G. Marck, A. Villiger, and R. Buchecker, Tetrahedron Lett., 1994, 35, 3277; CrossRef (e) D. Gala, A. Stamford, and M. Kugelman, Org. Process Res. Dev., 1997, 1, 163; CrossRef (f) D. S. Ennis, J. McManus, W. Wood-Kaczmar, J. Richardson, G. E. Smith, and A. Carstairs, Org. Process Res. Dev., 1999, 3, 248; CrossRef (g) C. R. LeBlond, F. Zhao, B. M. Bhanage, M. Shirai, and M. Arai, Chem. Eur. J., 2000, 6, 843; CrossRef (h) K. Köhler, R. G. Heidenreich, J. G. E. Krauter, and J. Pietsch, Chem. Eur. J., 2002, 8, 622; CrossRef (i) R. G. Heidenreich, K. Köhler, J. G. E. Krauter, and J. Pietsch, Synlett, 2002, 1118; CrossRef (j) A. T. Andrews, Y. Sun, and J. R. Sowa, Jr., Org. Lett., 2001, 3, 1555; CrossRef (k) Z. Novák, A. Szabó, J. Répási, and A. Kotschy, J. Org. Chem., 2003, 68, 3827; (l) T. Tagata and M. Nishida, J. Org. Chem., 2003, 68, 9412; CrossRef (m) A. Arcadi, G. Cerichelli, M. Chiarini, M. Correa, and D. Zorzan, Eur. J. Org. Chem., 2003, 4080; CrossRef (n) M. Lysén and K. Köhler, Synlett, 2005, 1671. CrossRef
13. Y. Kitamura, K. Taniguchi, T. Maegawa, Y. Monguchi, Y. Kitade, and H. Sajiki, Heterocycles, 2009, 77, 521. CrossRef
14. Y. Kitamura, K. Taniguchi, T. Maegawa, Y. Monguchi, Y. Kitade, and H. Sajiki, Heterocycles, 2014, 88, 233. CrossRef
15. Y. Monguchi, K. Nozaki, T. Maejima, Y. Shimoda, Y. Sawama, Y. Kitamura, Y. Kitade, and H. Sajiki, Green Chem., 2013, 15, 490. CrossRef
16. Y. Monguchi, S. Mori, S. Aoyagi, A. Tsutsui, T. Maegawa, and H. Sajiki, Org. Biomol. Chem., 2010, 8, 3338. CrossRef
17. Y. Monguchi, T. Marumoto, H. Takamatsu, Y. Sawama, and H. Sajiki, Adv. Synth. Catal., 2014, 356, 1866. CrossRef
18. (a) R. Huisgen, G. Szeimies, and L. Moebius, Chem. Ber., 1967, 100, 2494; CrossRef (b) R. Huisgen, in '1,3-Dipolar Cycloaddition Chemistry,' ed. by A. Padowa, Wiley, New York, 1984, pp. 1–176.
19. M. J. Giffin, H. Heaslet, A. Brik, Y.-C. Lin, G. Cauvi, C.-H. Wong, D. E. McRee, J. H. Elder, C. D. Stout, and B. E. Torbett, J. Med. Chem., 2008, 51, 6263. CrossRef
20. V. V. Rostovtsev, L. G. Green, V. V. Fokin, and K. B. Sharpless, Angew. Chem., 2002, 114, 2708; CrossRef Angew. Chem. Int. Ed., 2002, 41, 2596. CrossRef
21. C. W. Tornøe, C. Christensen, and M. Meldal, J. Org. Chem., 2002, 67, 3057. CrossRef
22. (a) V. D. Bock, H. Hiemstra, and J. H. van Maarseveen, Eur. J. Org. Chem., 2006, 51; CrossRef (b) M. Meldal and C. W. Tornøe, Chem. Rev., 2008, 108, 2952; CrossRef (c) H. C. Kolb, M. G. Finn, and K. B. Sharpless, Angew. Chem., 2001, 113, 2056; CrossRef Angew. Chem. Int. Ed., 2001, 40, 2004; CrossRef (d) H. C. Kolb and K. B. Sharpless, Drug Discovery Today, 2003, 8, 1128. CrossRef
23. (a) B. H. Lipshuz and B. R. Taft, Angew. Chem., 2006, 118, 8415; CrossRef Angew. Chem. Int. Ed., 2006, 45, 8235; CrossRef (b) P. Cintas, K. Martina, B. Robaldo, D. Garella, L. Boffa, and G. Cravotto, Collect. Czech. Chem. Commun., 2007, 72, 1014; CrossRef (c) H. Sharghi, R. Khalifeh, and M. M. Doroodmand, Adv. Synth. Catal., 2009, 351, 207; CrossRef (d) H. Kang, H. J. Lee, J. C. Park, H. Song, and K. H. Park, Top. Catal., 2010, 53, 523; CrossRef (e) F. Alonso, Y. Noglie, G. Radivoy, and M. Yus, Org. Biomol. Chem., 2011, 9, 6385. CrossRef
24. (a) T. Miao and L. Wang, Synthesis, 2008, 363; (b) T. Shamin and S. Paul, Catal. Lett., 2010, 136, 260; CrossRef (c) P. Veerakumar, M. Velayudham, K-L. Lu, and S. Rajagopal, Catal. Sci. Technol., 2011, 1, 1512. CrossRef
25. (a) S. Chassaing, M. Kumarraja, A. S. S. Sido, P. Pale, and J. Sommer, Org. Lett., 2007, 9, 883; CrossRef (b) A. Alix, S. Chassaing, P. Pale, and J. Sommer, Tetrahedron, 2008, 64, 8922; CrossRef (c) S. Chassaing, A. S. S. Sido, A. Alix, M. Kumarraja, P. Pale, and J. Sommer, Chem. Eur. J., 2008, 14, 6713; CrossRef (d) S. Chassaing, A. Alix, T. Boningari, K. S. S. Sido, M. Keller, P. Kuhn, B. Louis, J. Sommer, and P. Pale, Synthesis, 2010, 1557; (e) V. Bénéteau, A. Olmos, T. Boningari, J. Sommer, and P. Pale, Tetrahedron Lett., 2010, 51, 3673; CrossRef (f) R. Hosseinzadeh, H. Sepehrian, and F. Shahrokhi, Heteroat. Chem., 2012, 23, 415; CrossRef (g) R. Xiao, R. Yao, and M. Cai, Eur. J. Org. Chem., 2012, 4178. CrossRef
26. B. S. Lee, M. Yi, S. Y. Chu, J. Y. Lee, R. Kwon, K. R. Lee, D. Kang, W. S. Kim, H. B. Lim, J. Lee, H.-J. Youn, D. Y. Chi, and N. H. Hur, Chem. Commun., 2010, 46, 3935. CrossRef
27. K. Namitharan, M. Kumarraja, and K. Pitchumani, Chem. Eur. J., 2009, 15, 2755. CrossRef
28. I. S. Park, M. S. Kwon, Y. Kim, J. S. Lee, and J. Park, Org. Lett., 2008, 10, 497. CrossRef
29. (a) T. Katayama, K. Kamata, K. Yamaguchi, and N. Mizuno, ChemSusChem, 2009, 2, 59; CrossRef (b) K. Yamaguchi, T. Oishi, T. Katayama, and N. Mizuno, Chem. Eur. J., 2009, 15, 10464. CrossRef
30. A. Kumar, S. Aerry, A. Saxena, A. De, and S. Mozumdar, Green Chem., 2012, 14, 1298. CrossRef
31. (a) M. Lammens, J. Skey, S. Wallyn, R. O'Reilly, and F. D. Prez, Chem. Commun., 2010, 46, 8719; CrossRef (b) Z. Zhang, C. Dong, C. Yang, D. Hu, J. Long, L. Wang, H. Li, Y. Chen, and D. Kong, Adv. Synth. Catal., 2010, 352, 1600; CrossRef (c) Y. M. A. Yamada, S. M. Sarkar, and Y. Uozumi, J. Am. Chem. Soc., 2012, 134, 9285; CrossRef (d) T. Suzuka, Y. Kawahara, K. Ooshiro, T. Nagamine, K. Ogihara, and M. Higa, Heterocycles, 2012, 85, 615. CrossRef
32. (a) C. Girard, E. Önen, M. Aufort, S. Beauvière, E. Samson, and J. Herscovici, Org. Lett., 2006, 8, 1689; CrossRef (b) I. Jlalia, F. Meganem, J. Herscovici, and C. Girard, Molecules, 2009, 14, 528. CrossRef
33. U. Sirion, Y. J. Bae, B. S. Lee, and D. Y. Chi, Synlett, 2008, 2326.
34. (a) Home page for DIAION of Mitsubishi Chemical Co., see http://www.diaion.com/Index_E.htm; (b) T. Adachi, S. Ando, and J. Watanabe, J. Chromatogr. A, 2002, 944, 41. CrossRef
35. (a) 'Solvent-free Organic Synthesis, 2nd edition,' ed. by K. Tanaka, WILEY-VCH, Weinheim, 2008; CrossRef (b) 'Topics Current Chemistry 254, Organic Solid State Reactions, ' ed. by F. Toda, Springer, Berlin, 2005; (c) D. C. Dittmer, Chem. Ind., 1997, 19, 779; (d) R. S. Varma, Green Chem., 1999, 1, 43; CrossRef (e) K. Tanaka and F. Toda, Chem. Rev., 2000, 100, 1025; CrossRef (f) R. S. Varma, Pure Appl. Chem., 2001, 73, 193; (g) P. J. Walsh, H. Li, and C. A. de Parrodi, Chem. Rev., 2007, 107, 2503; CrossRef (h) M. A. P. Martins, C. P. Frizzo, D. N. Moreira, L. Buriol, and P. Machado, Chem. Rev., 2009, 109, 4140. CrossRef
36. Y. Monguchi, Y. Fujita, S. Hashimoto, M. Ina, T. Takahashi, R. Ito, K. Nozaki, T. Maegawa, and H. Sajiki, Tetrahedron, 2011, 67, 8628. CrossRef
37. (a) R. Koivula, J. Lehto, L. Pajo, T. Gale, and H. Leinonen, Hydrometallurgy, 2000, 56, 93; CrossRef (b) A. Cavaco, S. Fernandes, M. M. Quina, and L. M. Ferreira, J. Hazard. Mater., 2007, 144, 634; CrossRef (c) S. A. Cavaco, S. Fernandes, C. M. Augusto, M. J. Quina, and L. M. Gando-Ferreira, J. Hazard. Mater., 2009, 169, 516; CrossRef (d) L. M. Gando-Ferreira, I. S. Romao, and M. J. Quina, Chem. Eng. J., 2011, 172, 277. CrossRef
38. (a) A. J. Kochanowska-Karamyan and M. T. Harmann, Chem. Rev., 2010, 110, 4489; CrossRef (b) T. Balle, J. Perregaard, M. T. Ramirez, A. K. Larsen, K. K. Søby, T. Liljefors, and K. Andersen, J. Med. Chem., 2003, 46, 265. CrossRef
39. For review: (a) M. Inman and C. J. Moody, Chem. Sci., 2013, 4, 29; CrossRef (b) M. Platon, R. Amardeil, L. Djakovitch, and J. C. Hierso, Chem. Soc. Rev., 2012, 41, 3929; CrossRef (c) S. Cacchi and G. Fabrizi, Chem. Rev., 2011, 111, PR215; CrossRef (d) D. F. Taber and P. K. Tirunahari, Tetrahedron, 2011, 67, 7195; CrossRef (e) S. Cacchi and G. Fabrizi, Chem. Rev., 2005, 105, 2873; CrossRef (f) G. Battistuzzi, S. Cacchi, and G. Fabrizi, Eur. J. Org. Chem., 2002, 2671; CrossRef (g) G. W. Gribble, J. Chem. Soc., Perkin Trans. 1, 2000, 1045. CrossRef
40. T. Sakamoto, Y. Kondo, S. Iwashita, T. Nagano, and H. Yamanaka, Chem. Pharm. Bull., 1988, 36, 1305. CrossRef
41. (a) R. C. Larock and E. K. Yum, J. Am. Chem. Soc., 1991, 113, 6689; CrossRef (b) R. C. Larock, E. K. Yum, and M. D. Refvik, J. Org. Chem., 1998, 63, 7652. CrossRef
42. K. B. Hong, C. W. Chul, and E. K. Yun, Tetrahedron Lett., 2004, 45, 693. CrossRef
43. L. Djakovitch, V. Dufaud, and R. Zaidi, Adv. Synth. Catal., 2006, 348, 715. CrossRef
44. S. Chouzier, M. Gruber, and L. Djakovitch, J. Mol. Catal. A.: Chem., 2004, 212, 43. CrossRef
45. M. Pal, V. Subramanian, V. R. Batchu, and I. Dager, Synlett, 2004, 1965.
46. N. Batail, A. Bendjeriou, T. Lomberget, R. Barret, V. Dufaud, and L. Djakovitch, Adv. Synth. Catal., 2009, 351, 2055. CrossRef
47. J. S. Bajwa, G.-P. Chen, K. Prasad, O. Repič, and T. J. Blacklock, Terahedron Lett., 2006, 47, 6425. CrossRef
48. (a) Y.-B. Huang, C.-T. Yang, J. Yi, X.-J. Deng, Y. Fu, and L. Liu, J. Org. Chem., 2011, 76, 800; CrossRef (b) B. de Lange, D. J. Hyett, P. J. D. Mass, D. Mink, F. B. J. van Assema, N. Sereinig, A. H. M. de Vries, and J. G. de Vries, ChemCatChem, 2011, 3, 289; CrossRef (c) T. Kubo, C. Katoh, K. Yamada, K. Okano, H. Tokuyama, and T. Fukuyama, Tetrahedron, 2008, 64, 11230; CrossRef (d) A. Shafir and S. L. Buchwald, J. Am. Chem. Soc., 2006, 128, 8742; CrossRef (e) H. Zhang, Q. Cai, and D. Ma, J. Org. Chem., 2005, 70, 5164; CrossRef (f) A. S. Gajare, K. Toyota, M. Yoshifuji, and F. Ozawa, Chem. Commun., 2004, 1994; (g) F. Y. Kwong and S. L. Buchwald, Org. Lett., 2003, 5, 793; CrossRef (h) K. Yamada, T. Kubo, H. Tokuyama, and T. Fukuyama, Synlett, 2002, 231. CrossRef
49. D. Guo, H. Huang, Y. Zhou, J. Xu, H. Jiang, K. Chen, and H. Liu, Green Chem., 2010, 12, 276. CrossRef
50. R. Omar-Amrani, A. Thomas, E. Brenner, R. Schneider, and Y. Fort, Org. Lett., 2003, 5, 2311. CrossRef
51. (a) J. C. Anderson, A. Noble, and D. A. Tocher, J. Org. Chem., 2012, 77, 6703; CrossRef (b) R. Omar-Amrani, R. Schneider, and Y. Fort, Synthesis, 2004, 2527.
52. For the palladium-catalyzed annulation of nitrogen-protected 2-halophenethylamine derivatives: (a) H. J. C. Deboves, C. Hunter, and R. F. W. Jackson, J. Chem. Soc., Perkin Trans. 1, 2002, 733; CrossRef (b) J. A. Brown, Tetrahedron Lett., 2000, 41, 1623; CrossRef (c) A. S. Guram, R. A. Rennels, and S. L. Buchwald, Angew. Chem. Int. Ed. Engl., 1995, 34, 1348; CrossRef Angew. Chem., 1995, 107, 1456. CrossRef
53. For example: (a) F. Su, S. C. Mathew, A. M. Möhlmann, X. Wang, and S. Blechert, Angew. Chem. Int. Ed., 2011, 50, 657; Angew. Chem., 2011, 123, 683; CrossRef (b) Y. Nomura, Y. Kawashita, and M. Hayashi, Heterocycles, 2007, 74, 629; CrossRef (c) H. Chi and M. P. Doule, Chem. Commun., 2007, 745; CrossRef (d) T. Mukaiyama, A. Kawana, Y. Fukuda, and J. Matsuo, Chem. Lett., 2001, 390; CrossRef (e) M. Ochiai, D. Kajishima, and T. Sueda, Heterocycles, 1997, 46, 71; CrossRef (f) A. Inada, Y. Nakamura, and Y. Morita, Chem. Lett., 1980, 1287. CrossRef
54. H. Miyamura, M. Morita, T. Inasaki, and S. Kobayashi, Bull. Chem. Soc. Jpn., 2011, 84, 588. CrossRef
55. M.-H. So, Y. Liu, C.-M. Ho, and C.-M. Che, Chem. Asian J., 2009, 4, 1551. CrossRef
56. K. Yamaguchi and N. Mizuno, Angew. Chem. Int. Ed., 2003, 42, 1480; CrossRef Angew. Chem., 2003, 115, 1518. CrossRef
57. T. Hara, K. Mori, T. Mizugaki, K. Ebitani, and K. Kaneda, Tetrahedron Lett., 2003, 44, 6207. CrossRef
58. A. Moores, M. Poyatos, Y. Luo, and R. H. Crabtree, New J. Chem., 2006, 30, 1675. CrossRef
59. (a) Orito et al. reported that the N-unprotected phenethylamine derivatives, 2-bromo-4,5-dimethoxyphenethylamine and 2-bromo-4,5-methylenedioxyphenethylamine, underwent cyclization and aromatization to directly generate indole derivatives, but in approximately 30% yields, by the treatment with K2CO3 or NaOt-Bu in the presence of a catalytic amount of Pd(OAc)2 and Cu(OAc)2 in refluxed toluene. R. Harada, N. Nishida, S. Uchiito, Y. Onozaki, N. Kurono, H. Senboku, T. Masao, T. Ohkuma, and K. Orito, Eur. J. Org. Chem., 2012, 366; CrossRef (b) Tokuyama et al. described in their total synthesis of PDE-II that the N-Ns-protected 2-bromoaniline derivative could be directly converted to the corresponding N-unprotected indole derivative by the CuI-catalyzed intramolecular amination followed by the in-situ removal of the Ns group and aromatization. K. Okano, N. Mitsuhashi, and H. Tokuyama, Chem. Commun., 2010, 46, 2641. CrossRef
60. (a) G. A. Grasa, M. S. Viciu, J. Huang, and S. P. Nolan, J. Org. Chem., 2001, 66, 7729; CrossRef (b) D. W. Old, M. C. Harris, and S. L. Buchwald, Org. Lett., 2000, 2, 1403; CrossRef (c) G. Mann, J. F. Hartwig, M. S. Driver, and C. Fernández-Rivas, J. Am. Chem. Soc., 1998, 120, 827. CrossRef
61. For general review for N-1, C-2 or C-3 site-selective arylation of indole: L. Joucla and L. Djakovitch, Adv. Synth. Catal., 2009, 351, 673. CrossRef