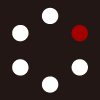
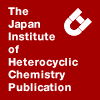
HETEROCYCLES
An International Journal for Reviews and Communications in Heterocyclic ChemistryWeb Edition ISSN: 1881-0942
Published online by The Japan Institute of Heterocyclic Chemistry
e-Journal
Full Text HTML
Received, 18th June, 2013, Accepted, 4th July, 2013, Published online, 16th July, 2013.
DOI: 10.3987/REV-13-776
■ Synthesis of Functionalized Cyclic Molecules by Palladium-Catalyzed Cyclization of Propargylic Esters with Bis-nucleophiles
Masahiro Yoshida*
Graduate School of Pharmaceutical Sciences, The University of Tokushima, Sho-machi, Tokushima 770-8505, Japan
Abstract
It is known that propargylic esters react with palladium complex leading to π-propargylpalladium complexes, which further cause various transformations in the presence of soft nucleophiles to produce the corresponding products. Among them, palladium-catalyzed cyclization of propargylic esters with bis-nucleophiles is one of the useful methodologies for the construction of functionalized cyclic molecules in one step. Since the pioneering work reported by Tsuji in 1985, a considerable number of reactions have been reported by the use of various bis-nucleophiles. In this review, a comprehensive overview of the studies on palladium-catalyzed reactions of propargylic esters with bis-nucleophiles is described, in which various functionalized cyclic molecules can be synthesized in a regio- and stereoselective manner.CONTENTS
1. Introduction
2. Use of β-Dicarbonyl Compounds as a Nucleophile
2-1. 2-Substituted 1,3-Diketones
2-2. Cyclic β-Keto Esters
2-3. β-Hydroxy-α-pyrones
2-4. 2-Oxocyclohex-3-enecarboxylates
2-5. β-Keto Esters with Aryl Halides
2-6. Propargyl β-Keto Esters
3. Use of β-Enamino Esters as a Nucleophile
4. Use of Catechol as a Nucleophile
5. Use of 2-Hydroxyphenylacetic Acetic Acid Esters as a Nucleophile
6. Conclusion
1. INTRODUCTION
Propargylic compounds containing an ester or a halide at the propargylic positions are known as useful intermediates in organic synthesis, and a number of reactions utilizing the properties of propargylic compounds have been reported.1–3 For example, propargylic carbonates react with palladium complex leading to π-propargylpalladium complexes, which further cause various transformations in the presence of soft nucleophiles to produce the 1,2-disubstituted allylic compounds along with the regenerated palladium complexes (Scheme 1).2,3 In the reaction, a nucleophile initially attacks to the central carbon of π-propargylpalladium to transform the π-allylpalladium complex via the palladacyclobutene,4,5 which further reacts with another nucleophile to afford the disubstituted products. Various transformations including cyclization reactions have been developed by the design of propargylic substrates and nucleophiles.
As an example of the reaction, we have reported the synthesis of 2-substituted benzofurans by the reaction of 2-phenoxy-substituted propargylic carbonate 1 with 2-methyl-1,3-cyclohexanedione (2) (Scheme 2).6 In the reaction, the intramolecular nucleophilic attack of the phenolic oxygen to the π-propargylpalladium 3 gave the π-allylpalladium intermediate 4, which was subjected to the intermolecular nucleophilic attack by 2 to afford the product 5.
We also found the formation of substituted cyclic carbonates by the “CO2-recycling” reaction of propargylic carbonates 6 having a hydroxyl group with phenols (Scheme 3).7 In the reaction, decarboxylation of 6 triggered by palladium initially occurred to form the π-propargylpalladium 7, which caused the nucleophilic attack by phenoxide leading to the π-allylpalladium 8. The intermediate 8 then trapped the liberated CO2 in situ to afford the carbonate species 9, which was transformed to the phenoxy-substituted cyclic carbonate 10 by intramolecular cyclization. In these reactions, a variety of cyclic molecules can be synthesized by introducing the nucleophilic moiety within the propargylic esters.
As another type of the palladium-catalyzed cyclization of propargylic compounds with nucleophiles, it is known that the use of bis-nucleophiles, which contain two nucleophilic parts within the molecules. In this reaction, the initially formed π-propargylpalladium intermediate is subjected to consecutive nucleophilic attacks by the bis-nucleophilic part to give the cyclized product (Scheme 4).
As this pioneering work, Tsuji reported propargylic carbonates 11 reacted with 1,3-diketone 12 to produce substituted furans 13 (Scheme 5).8 In this reaction, the π-propargylpalladium complex, which derived from propargylic carbonates and a palladium catalyst, reacted with 1,3-diketone to give the π-allylpalladium complex. Then the complex caused the intramolecular nucleophilic attack of the enolate oxygen followed by the isomerization of the olefin to produce the cyclized product 13. This type of cyclization is useful for the synthesis of various heterocyclic compounds in one step, and extensive studies about this process have been examined in recent years. In this review, a comprehensive overview of the studies on palladium-catalyzed reactions of propargylic esters with bis-nucleophiles is described, in which various functionalized cyclic molecules can be synthesized in a regio- and stereoselective manner.
2. USE OF β-DICARBONYL COMPOUNDS AS A NUCLEOPHILE
2-1. 2-Substituted 1,3-Diketones
Although the use of 1,3-diketones as a bis-nucleophile in the palladium-catalyzed cyclization of propargylic esters have been reported by Tsuji as shown in Scheme 5, the stereochemistry of the reaction has not been well examined. During the course of our early studies about palladium-catalyzed reactions of propargylic compounds,6,7,9 we decided to use 2-substituted 1,3-diketones with propargylic esters in the diastereoselective synthesis of cyclic molecules. When propargylic carbonate 14a and 2-methylcyclohexane-1,3-dione (15a) were treated with 5 mol % Pd2(dba)3·CHCl3 and 20 mol % DPPF in DMSO at 120 °C for 5 min, tetrahydrobenzofuranone 16aa having trans-stereochemistry was produced in 84% yield as a single diastereomer (Scheme 6).10
A proposed mechanism for the production of 16aa is shown in Scheme 7. The propargylic carbonate 14a reacts with palladium to form the π-propargylpalladium complex, which causes the nucleophilic attack of 1,3-diketone 15a to give the π-allylpalladium intermediate via the palladacyclobutene.4 The π-allylpalladium intermediate is further subjected to the intramolecular attack of the enolate oxygen to give the cyclized product 16aa. The observed trans-diastereoselectivity would be explained via the transition state 17, which has lower energy because of the absence of steric repulsion between the phenyl and methyl groups.
Table 1 shows the examinations using various substrates. When the reaction of 2-propylcyclohexane-1,3-dione (15b) with 14a was attempted, the propyl-substituted product 16ab was produced in 75% yield (entry 1). The benzyl- and 2-cyanoethyl-substituted substrates 15c and 15d uneventfully reacted with 14a to afford the corresponding products 16ac and 16ad in 76% and 82% yields, respectively (entries 2 and 3). The reactions of propargylic carbonates 14b and 14c containing a 1- and 2-naphthyl group at the propargylic position with 15a also proceeded to produce the corresponding products 16ba and 16ca, respectively (entries 4 and 5). Reactions using the 3-furanyl- and n-pentyl-substituted substrates 14d and 14e gave the corresponding products 16da and 16ea in moderate yields (entries 6 and 7).
2-2. Cyclic β-Keto Esters
We next examined the reaction of propargylic carbonates with the cyclic β-keto ester. When propargylic carbonate 14a and methyl 2-oxocyclohexanecarboxylate (18) were subjected to the reaction with 5 mol % Pd2(dba)3·CHCl3 and 20 mol % DPPF in DMSO at 120 °C for 5 min, trans-substituted hexahydrobenzofuran 19a was obtained in 92% yield as a single diastereomer (Scheme 8).10,11
As the proposed mechanism, initially formed π-propargylpalladium complex from the propargylic carbonate 14a reacts with the β-keto ester 18 to give to the π-allylpalladium intermediate. Then intramolecular nucleophilic attack via the favorable transition state 20 occurs to afford the cyclized product 19a (Scheme 9).
The reactions using various propargylic carbonates 14b–e with 18 are summarized in Table 2. The reactions of the naphthyl-substituted substrates 14b and 14c proceeded to produce the cyclized products 19b and 19c in 66% and 76% yields, respectively (entries 1 and 2). The substrates 14d and 14e having a 3-furanyl and a n-pentyl group also reacted to afford the corresponding products 19d and 19e in 51% and 56% yields, respectively (entries 3 and 4). It was clear that these reactions proceed in a highly diastereoselective manner since the resulting hexahydrobenzofurans 19a–e were produced as a single diastereomer.
The reaction of the 3,4-dimethoxyphenyl-substituted propargylic acetate 21 with the 2-oxocyclohexanecarboxylic ester 18 in the presence of K3PO4 afforded the corresponding hexahydrobenzofuran 19f in 81% yield (Scheme 10). The resulting product 19f contains a core structure of porosin, a neolignan from Ocotea porosa and Urbanodendron verrucosum,12 and this cyclization reaction could be synthetically useful for the synthesis of this kind of natural products.
2-3. β-Hydroxy-α-pyrones
Furo[3,2-c]pyran-4-ones are one of the important class of heteroaromatic molecules which are components in a variety of biologically active natural products.13 We focused on the nucleophilic activity of 4-hydroxy-2-pyrones as a bis-nucleophile, which is expected to construct the furo[3,2-c]pyran-4-ones by the palladium-catalyzed cyclization with propargylic esters. When propargylic carbonate 14a and 4-hydroxy-2-pyrone 22a were treated with 5 mol % of Pd2(dba)3·CHCl3, 20 mol % DPPF in NMP at 25 ºC, the reaction proceeded to afford the 2-phenyl-substituted furopyranone 23aa in 89% yield as a sole product (Scheme 11).14 Interestingly, the 3-benzylidene-subsituted furopyranone 24aa was also obtained as the reaction temperature was increased. Thus, the regioisomer 24aa was produced together with 23aa when the reaction was carried out at 100 ºC (23aa:24aa = 1:1.9, 86% yield), and 24aa was exclusively obtained in 79% yield in the reaction at 120 ºC. From these results, it has been found that the regioselectivity of the reaction can be controlled depending on the reaction temperature.
A proposed mechanism for the formation of the furopyranones 23aa and 24aa is shown in Scheme 12. The π-propargylpalladium complex, resulting from the propargylic carbonate 14a with palladium, reacts with the 4-hydroxy-2-pyrone 22a to form the π-allylpalladium intermediate 25. The intermediate 25 is further subjected to intramolecular attack of the hydroxy anion to produce the cyclized product 23aa or 24aa. The observed regioselectivity depending on the reaction temperature would be the result of kinetic and thermodynamic control in the cyclization process. In the low reaction temperature, it is expected that the cyclization occurs via path A to provide 23aa as the kinetic product. On the other hand, there could be equilibrium between the products and π-allylpalladium intermediate 25 at the high temperature. As a result, the thermodynamically more stable 24aa would be selectively formed via path B.
Table 3 shows the examinations using various 4-hydroxy-2-pyrones 22b–f15 with 14a. When the substrate 22b having no substituent on the 3-position of the pyrone ring was subjected to the reaction at 25 ºC, the 2-phenyl-substituted furopyranone 23ab was produced in 47% yield (entry 1). Similarly, the substrates 22c–f containing an ethyl, a heptyl, an allyl and a benzyl group were transformed to the corresponding kinetic products 23ac–af in moderate yields, respectively (entries 2–5). On the other hand, the corresponding 3-benzylidene-subsituted furopyranones 24ab–af were solely produced as the thermodynamic products in each cases when the 4-hydroxy-2-pyrones 22b–f were subjected to the reaction at 120 ºC (entries 6–10).
2-4. 2-Oxocyclohex-3-enecarboxylates
As an application of the use of β-keto esters in the palladium-catalyzed reaction with propargylic esters, we focused on the nucleophilic activity of 2-oxocyclohex-3-enecarboxylates 26. We initially expected that the tetrahydrobenzofuran 27 could be obtained via the π-allylpalladium-dienolate intermediate 28 (Scheme 13). As the result, the unexpected bicyclo[3.2.1]octenone 30aa was produced in 81% yield as a single stereoisomer when 1-phenyl-2-propynyl acetate (29a) and 2-oxocyclohex-3-enecarboxylate 26a were treated with 5 mol % of Pd2(dba)3·CHCl3, 20 mol % DPPF and K3PO4 in DMSO at 120 ºC for 5 min.16
A proposed mechanism for the production of the bicyclo[3.2.1]octenone 30aa is shown in Scheme 14. After the formation of the π-propargylpalladium complex from 29a with palladium, the nucleophilic attack of the α-carbon of the 2-oxocyclohex-3-enecarboxylate 26a gave the π-allylpalladium intermediate 31. The complex 31 is then subjected to intramolecular attack of the γ-carbon of the enone moiety via the transition state 32 to produce the bicyclo[3.2.1]octenone 30aa.
Examinations using various substituted substrates are summarized in Table 4. When the reactions of the propargylic acetates 29b and 29c having a p-methoxyphenyl and a p-fluorophenyl group with 26a were carried out, the bicyclo[3.2.1]octenones 30ba and 30ca were produced in 69% and 80% yield, respectively (entries 1 and 2). The substrates 29d–f containing a 2-naphthyl, a 3-furanyl and a cyclohexyl group also reacted to produce the corresponding products 30da–fa in good yields (entries 3–5). When the reaction of the 2-oxocyclohex-3-enecarboxylate 26b containing a benzyl ester moiety with 29a was carried out, the bicyclo[3.2.1]octenone 30ab was produced in 82% yield (entry 6). The reactions of 26c having a methyl group on the α-position of the enone system also proceeded to give the corresponding product 30ac in 64% yield (entry 7). The substrate 26d, which has a phenyl group at the β-position, was successfully transformed to the bicyclo[3.2.1]octenone 30ad in 75% yield (entry 8). The process produces substituted bicyclo[3.2.1]octenones in a highly stereoselective manner. Since various natural products having a bicyclo[3.2.1]octane structure have been reported,17 our methodology would provide a new protocol for the synthesis of these compounds with high efficiency.
2-5. β-Keto Esters with Aryl Halides
As an application of the synthesis of substituted furans by palladium-catalyzed reaction of propargylic carbonates with β-dicarbonyl compounds, Liang reported that one-pot synthesis of tetrasubstituted furans by a three-component coupling–cyclization reaction (Scheme 15).18 When propargylcarbonate 33, acetoacetic acid methyl ester and iodobenzene were treated with 5 mol % Pd(PPh3)4 and K2CO3 in DMF at 100 ºC, the tetrasubstituted furan 34 was produced in 50% yield.
A tentative mechanism for the production of 34 was shown in Scheme 16. Oxidative addition of iodobenzene to the palladium initially generates the phenylpalladium iodide, which would coordinate with the propargylcarbonate 33 to transform to the phenylalkynylpalladium 35 in the presence of base. The complex then undergoes decarboxylation to afford the π-propargylpalladium 36, which would further react with acetoacetic acid methyl ester to afford the tetrasubstituted furan 34.
2-6. Propargyl β-Keto Esters
Palladium-catalyzed allylic substitutions with nucleophiles have been extensively studied due to their versatile and specific reactivities,19 in which soft carbanions derived from β-dicarbonyl compounds are suitable as nucleophiles. On the other hand, examples of the reaction with non-stabilized carbanions such as monoketone enolates are limited.20 A solution to this problem highlights a decarboxylative allylation of allyl β-keto esters, in which the non-stabilized monoketone enolate is effectively formed in situ to afford an allylated product (Scheme 17).21
In planning our application about the reaction of propargylic esters with bis-nucleophiles, we focused on the reactivity of propargyl β-keto esters. In contrast to the extensive studies on the reaction of allyl β-keto esters, no examples have been reported on the reactivity of propargyl β-keto esters with palladium. When propargyl β-keto ester 37a was treated with 5 mol % Pd2(dba)3·CHCl3 and 20 mol % DPPF in dioxane at 65 ºC, the reaction proceeded to afford the cyclized tetrahydrobenzofuran 38a in 76% yield (Sceheme 18).22
A proposed mechanism for the formation of the tetrasubstituted furans 38a is shown in Scheme 19. The propargyl β-keto ester 37a undergoes decarboxylation by palladium to form the π-propargylpalladium enolate 39, which is in equilibrium with the ion pair 40. Then nucleophilic attack of the enolate on the π-propargylpalladium undergoes to lead to the π-allylpalladium intermediate 41. The intermediate 41 is subjected to an intramolecular attack by the enolate oxygen to produce the dihydrofuran 42, which further isomerizes to afford the substituted furan 38a.
The reactions using various propargyl β-keto esters 37b–h are summarized in Table 5. When the reactions of the substrates 37b and 37c having a 4-fluorophenyl and a 3,4-dimethoxyphenyl group were carried out, the tetrahydrobenzofurans 38b and 38c were obtained in moderate yields (entries 1 and 2). The reaction of the substrate 37d, which have a 3-furyl group, also proceeded to afford the tetrahydrobenzofuran 38d in 79% yield (entry 3). The reactions of the substrates 37e having a cyclooctanone ring successfully proceeded to give the cycloocta[b]furan 38e in 74% yield (entry 4). The substrate 37f, which have a 1-tetralone moiety, was also converted to the corresponding product 38f in 70% yield (entry 5). When the reaction of 37g having a phenyl group at the 4-position of the cyclohexane ring was carried out, the corresponding substituted tetrahydrobenzofuran 38g was obtained as the sole product (entry 6). This result indicates that the reaction proceeds via the regioselective formation of the corresponding enolate. The acyclic propargyl β-keto ester 37h also reacted with the palladium catalyst to produce the substituted furan 38h, but the yield was decreased to 21% (entry 7).
3. USE OF β-ENAMINO ESTERS AS A NUCLEOPHILE
Pyrroles are important components as a structural fragment of many biologically active natural products, pharmaceutical agents and electronic and magnetic materials.23 During the course of our study for the construction of various cyclic molecules, we took notice of the nucleophilic activity of β-enamino esters, which could be converted to the substituted pyrroles by the reaction with propargylic esters in the presence of palladium. When propargylic carbonate 43a and tosyl-substituted β-enamino ester 44 were treated with 5 mol % of Pd2(dba)3·CHCl3, 20 mol % DPPE in THF at 50 ºC, tetrasubstituted pyrroles 45a was obtained in 96% yield (Scheme 20).24
A plausible mechanism for the production of the pyrrole is shown in Scheme 21. The propargylic carbonate 43a reacts with palladium to transform to the π-propargylpalladium complex, which causes nucleophilic attack of the α-carbon of the β-enamino ester 44 followed by proton transfer affording the π-allylpalladium intermediate 46. Then the intramolecular nucleophilic attack of the sulfonamide anion to the π-allylpalladium followed by isomerization of the resulting 47 proceeds to give the tetrasubstituted pyrrole 45a.
The reactions of various propargylic carbonates 43b–h with β-enamino ester 44 are summarized in Table 6. When the reactions of the substrates 43b and 43c containing a p-methoxyphenyl and a p-fluorophenyl group at the propargylic position were attempted, tetrasubstituted pyrroles 45b and 45c were produced in 91% and 90% yield, respectively (entries 1 and 2). The substrate 43d having a 3-furyl a group also reacted with 44 to give the product 45d in 76% yield (entry 3). When the propargylic carbonate 43e containing a phenyl group on the alkynyl moiety was subjected to the reaction, the tetrasubstituted pyrrole 45a, which was the same product from the reaction of 43a, was obtained in 92% yield (entry 4). The result indicates that the reaction proceeds via the formation of a common π-allylpalladium intermediate 46 in Scheme 21. Similarly, the corresponding products 45f and 45g were obtained in high yields from the reactions using pentyl- and ethyl-substituted substrates 43f and 43g (entries 5 and 6). The diphenyl-substituted substrate 43h uneventfully reacted with 44 to deliver the corresponding product 45h in 82% yield (entry 7).
4. USE OF CATECHOLS AS A NUCLEOPHILE
Synthesis of 1,4-benzodioxanes have attracted considerable attention because of their interesting biological activities.25 Sinou reported the synthesis of 2-alkylidene-1,4-benzodioxanes by utilizing a catechol as a bis-nucleophile in the palladium-catalyzed cyclization with propargylic carbonates. When propargylic carbonate 14a and catechol were treated with 2.5 mol % Pd2(dba)3·CHCl3 and 20 mol % DPPB in THF at 25 °C for 24 h, 3-phenyl-2-methylene-1,4-benzodiozane 48 and 2-benzylidene- 1,4-benzodiozane 49 were produced in 60% and 40% yields, respectively (Scheme 22).26
Scheme 23 shows a predicted mechanism for the formation of 48 and 49. The π-propargylpalladium complex, resulting from propargylic carbonate 14a with palladium, would cause the nucleophilic attack of the phenolic oxygen of a catechol to afford the π-allylpalladium intermediate 50. Then, intramolecular nucleophilic attack of the another phenolic oxygen via 51 or 51’ would occur to afford the 1,4-benzodioxanes 48 and 49.
Although the regioselectivity of this reaction was not high, Sinou reported the regiocontrolled synthesis of substituted 1,4-benzodioxanes by the use of substituted propargylic carbonates (Scheme 24).27 Thus, when the reaction of a propargylic carbonate 52 containing a hydroxyethyl group with catechol was carried out, 3-substituted-2-methylene-1,4-benzodioxane 53 was selectively produced in 85% yield in accordance with its regioisomer 54 in 5% yield. Furthermore, the 2-methoxycarbonylmethylidene-1,4-benzodioxane 56 was produced in 84% yield as a sole product when the substrate 55 having an ester moiety at the alkynyl position was subjected to the reaction.
As a reason for the observed regioselectivity depending on the functional group on the propargylic substrate, Sinou proposed directing effects of the substituents on the propargylic carbonates (Scheme 25). In case of the hydroxyethyl-substituted substrate 52, it is expected that the cyclization occurs via the intermediate 57, which forms a hydrogen bond with the hydroxyl function, to provide 53 as a major product. On the other hand, there could be complexation of the ester to the palladium in the reaction of 55. As a result, the 1,4-benzodioxane 56 would be regioselectively formed via the intermediate 58.
As an application of this cyclization process, Sinou examined to synthesize optically active 1,4-benzodioxanes in the presence of chiral palladium catalyst. As a result, when the reaction of (±)-59 with catechol was carried out in the presence of 2.5 mol % Pd2(dba)3·CHCl3 and 10 mol % (R)-BINAP in THF at 25 °C for 24 h, the optically active 3-phenyl-2-benzylidene-1,4-benzodioxane (+)-60 was obtained in 98% yield with 96% ee (Scheme 26).28 In this reaction, the achiral π-allylpalladium intermediate 61 would be formed from the racemic substrate 59, and it is presumed that the enantioselective cyclization from 61 would occur in the presence of chiral phosphine ligand.
5. USE OF 2-HYDROXYPHENYLACETIC ACID ESTERS AS A NUCLEOPHILE
We next focused on the reactivity of 2-(2-hydroxyphenyl)acetates as a bis-nucleophile. By introducing phenolic oxygen and an α-carbon of ester moiety as the nucleophilic part within the substrate, we expected that substituted chromans, common structures in many pharmaceutical and agricultural compounds,29 could be synthesized. When propargylic carbonate 59 and 2-(2-hydroxyphenyl)acetate (62a) were subjected to the reaction in the presence of 5 mol % Pd2(dba)3·CHCl3 and 20 mol % DPPF in DMSO at 120 °C for 5 min, the reaction successfully proceeded to afford the substituted chroman 63a having the Z-alkenyl moiety with trans-stereochemistry in 99% yield as a single stereoisomer (Scheme 27).30
A plausible mechanism for this diastereoselective cyclization is shown in Scheme 28. The π-propargylpalladium complex, derived from the propargylic carbonate 59 with palladium, is subjected to the nucleophilic attack of the 2-(2-hydroxyphenyl)acetate 62a leading to the π-allylpalladium intermediate 64. The complex 64 further causes the intramolecular attack of the α-carbon of the ester moiety to produce the chroman 63a. In the cyclization step, it is expected that there are two transition states, 65 and 65’. The desired trans-product 63a would be produced via 65, which would have lower energy because of the absence of steric repulsion between the ester and aryl groups that is present in 65’. As another possibility, the epimerization of the initially produced cis-product to the thermodynamically stable trans-product could occur during the reaction.
Attempts using various 2-(2-hydroxyphenyl)acetates 62b–e are summarized in Table 7. The reaction of benzyl 2-(2-hydroxyphenyl)acetate (62b) with 59 proceeded to afford the substituted chroman 63b in 99% yield (entry 1). The substrates 62c and 62d having a methoxy group at the 2- and 4-position on the benzene ring also reacted to give the corresponding products 63c and 63d in 80% and 88% yields, respectively (entries 2 and 3). When the naphthyl-substituted substrate 62e was subjected to the reaction, the corresponding product 63e was produced in 51% yield (entry 4). Since the resulting products 63a–e had been obtained as a single stereoisomer in all cases, it was clear that the reaction proceeded in a highly stereoselective manner.
We attempted applying this process to an enantioselective reaction. In the synthesis of chromans, an asymmetric center is formed via the achiral π-allylpalladium intermediate 64 as shown in Scheme 28, and it is presumed that the absolute configuration of the newly formed stereogenic center could be controlled by chiral palladium catalysts. After several attempts using various chiral phosphine ligands, we found that the optically active chroman (3R,4S)-63b was produced in 59% yield with 96% ee when (S)-SEGPHOS was employed at 60 ºC in the reaction of (±)-59 with 62b (Scheme 29).
A plausible mechanism for the appearance of enantioselectivity is shown in Scheme 30. The enantioselectivity is determined during the cyclization of the corresponding π-allylpalladium intermediate, and there are two possible transition states 66 and 66’. It is presumed that the observed selectivity is related to the thermodynamic stability of the resulting Pd(olefin) complexes which are postulated as primary products.31 Assuming a pseudo-square planar coordination geometry, the palladium complex 67 is expected to be more stable than 67’, based on steric considerations. Therefore, it is plausible that the reaction would take place via the favored transition state 67 to provide (3R,4S)-63b.
6. CONCLUSION
Examples about the palladium-catalyzed cyclization of propargylic compounds with bis-nucleophiles were presented, in which various functionalized molecules were synthesized depending on the type of bis-nucleophiles. By using 2-substituted cyclic 1,3-diketones as bis-nucleophiles, we have developed a diastereoselective synthesis of tetrahydrobenzofuranones by a palladium-catalyzed reaction of propargylic carbonates. The reaction using cyclic β-keto esters has also proceeded to produce the substituted tetrahydrobenzofuran having the trans-stereochemistry in a highly stereoselective manner. The regioselective synthesis of substituted furo[3,2-c]pyran-4-one derivatives have been achieved by a cyclization of propargylic carbonates with 4-hydroxy-2-pyrones. The regioselectivity of the reaction can be controlled depending on the reaction temperature. We also observed the palladium-catalyzed reaction of propargylic acetates with 2-oxocyclohex-3-enecarboxylates. The process produces substituted bicyclo[3.2.1]octenones in a highly stereoselective manner. One-pot synthesis of tetrasubstituted furans has been developed by a three-component coupling–cyclization reaction of propargylic carbonate, β-keto esters and aryl halide. We have established a palladium-catalyzed reaction of propargyl β-keto esters, in which tetrasubstituted furans having a variety of substituents were produced via a decarboxylative [3+2] cyclization pathway. By using β-enamino esters as bis-nucleophiles, we have developed a synthesis of tetrasubstituted pyrroles by a palladium-catalyzed reaction of propargylic carbonates. This process regioselectively produces tetrasubstituted pyrroles having a variety of substituents via a successive nucleophilic cyclization. Substituted 1,4-benzodioxanes were produced by the reaction of a catechol with propargylic carbonates. In this reaction, the regiocontrolled synthesis of substituted 1,4-benzodioxanes have been accomplished by the use of substituted propargylic carbonates. Optically active 1,4-benzodioxanes were obtained by carrying out the reaction in the presence of chiral ligand. The palladium-catalyzed reaction of propargylic carbonates with 2-(2-hydroxyphenyl)acetates has been achieved. The process produces substituted chromans having the trans-stereochemistry with the Z-alkenyl moiety in a highly stereoselective manner. Enantioselective reactions proceeded successfully in the presence of chiral ligand to give the optically active chromans with high enantioselectivity. We believe that these reactions could provide useful methodologies in the field of synthetic organic chemistry.
ACKNOWLEDGEMENTS
I am very grateful to Professor Emeritus Kozo Shishido of The University of Tokushima for valuable advice on this research. I would like to express my sincere appreciation to all students whose names are acknowledged on our publications cited in this review for their dedication and excellent creativity. This study was supported in part by a Grant-in-Aid for the Encouragement of Young Scientists (B) from the Japan Society for the Promotion of Science (JSPS).
References
1. a) H.-J. Attenbach, Comprehensive Organic Synthesis Vol. 6; ed. by B. M. Trost and I. Fleming, Pergamon Press: Oxford, 1991, pp. 829–871; b) M. J. Curtis-Long and Y. Aye, Chem. Eur. J., 2009, 15, 5402; CrossRef c) H. Yamamoto, Comprehensive Organic Synthesis, Vol. 2; ed. by B. M. Trost and I. Fleming, Pergamon Press: Oxford, 1991, pp. 81–98; d) T. J. J. Müller, Eur. J. Org. Chem., 2001, 2021; CrossRef e) J. Marco-Contelles and E. Soriano, Chem. Eur. J., 2007, 15, 1350; CrossRef f) A. Fürstner, Chem. Soc. Rev., 2009, 38, 3208; CrossRef g) N. Marison and S. P. Nolan, Angew. Chem. Int. Ed., 2007, 46, 2750. CrossRef
2. a) J. Tsuji, Palladium Reagents and Catalysts: Innovations in Organic Synthesis; Wiley: New York, 1995, pp. 453–471; b) J. Tsuji, Palladium Reagents and Catalysts: New Perspectives for the 21st Century; Wiley: England, 2004, pp. 543–563; CrossRef c) J. Tsuji and T. Mandai, Angew. Chem. Int. Ed., 1995, 34, 2589; CrossRef d) L.-N. Guo, X.-H. Duan, and Y.-M. Liang, Acc. Chem. Res., 2011, 44, 111; CrossRef e) M. Yoshida, Chem. Pharm. Bull., 2012, 60, 285. CrossRef
3. It is also known that other transition metal complex react with propargylic compounds, see ref. 1c–g.
4. a) M. W. Baize, P. W. Blosser, V. Plantevin, D. G. Schimpff, J. C. Gallucci, and A.Wojcicki, Organimetallics, 1996, 15, 164; CrossRef b) K. Tsutsumi, T. Kawase, K. Kakiuchi, S. Ogoshi, Y. Okada, and H. Kurosawa, Bull. Chem. Soc. Jpn., 1999, 72, 2687; CrossRef c) T. Murahashi, S. Ogoshi, and H. Kurosawa, Chem. Record, 2003, 3, 101. CrossRef
5. Tsuji proposed another reaction pathway in which formation of a palladium-carbene intermediate is preceded by nucleophilic addition to the allenylpalladium complex. (ref. 2a-c).
6. M. Yoshida, Y. Morishita, M. Fujita, and M. Ihara, Tetrahedron, 2005, 61, 4381. CrossRef
7. a) M. Yoshida and M. Ihara, Angew. Chem. Int. Ed., 2001, 40, 616; CrossRef b) M. Yoshida, M. Fujita, T. Ishii, and M. Ihara, J. Am. Chem. Soc., 2003, 125, 4874; CrossRef c) M. Yoshida and M. Ihara, Chem. Eur. J., 2004, 10, 2886. CrossRef
8. J. Tsuji, I. Watanabe, I. Minami, and I. Shimizu, J. Am. Chem. Soc., 1985, 107, 2196. CrossRef
9. a) M. Yoshida, H. Nemoto, and M. Ihara, Tetrahedron Lett., 1999, 40, 8583; CrossRef b) M. Yoshida, M. Fujita, and M. Ihara, Org. Lett., 2003, 5, 3325; CrossRef c) M. Yoshida, Y. Komatsuzaki, H. Nemoto, and M. Ihara, Org. Biomol. Chem., 2004, 2, 3099; CrossRef d) M. Yoshida, Y. Morishita, and M. Ihara, Tetrahedron Lett., 2005, 46, 3669; CrossRef e) M. Yoshida, H. Ueda, and M. Ihara, Tetrahedron Lett., 2005, 46, 6705; CrossRef f) M. Yoshida, T. Murao, K. Sugimoto, and M. Ihara, Synlett, 2007, 575. CrossRef
10. M. Yoshida, M. Higuchi, and K. Shishido, Tetrahedron Lett., 2008, 49, 1678. CrossRef
11. M. Yoshida, M. Higuchi, and K. Shishido, Tetrahedron, 2010, 66, 2675. CrossRef
12. C. J. Aiba, R. B. Filho, and O. R. Gottlieb, Phytochemistry, 1973, 12, 413. CrossRef
13. For recent representative examples: a) J.-Y. Cho, Y.-J. Kwon, M.-J. Sohn, S.-J. Seok, and W.-G. Kim, Bioorg. Med. Chem. Lett., 2011, 21, 1716; CrossRef b) I.-K. Lee, M.-S. Han, M.-S. Lee, Y.-S. Kim, and B.-S. Yun, Bioorg. Med. Chem. Lett., 2010, 20, 5459; CrossRef c) Y.-L. Yang, W.-Y. Liao, W.-Y. Liu, C.-C. Liaw, C.-N. Shen, Z.-Y. Huang, and S.-H. Wu, Chem. Eur. J., 2009, 15, 11573; CrossRef d) I.-K. Lee, S.-J. Seok, W.-K. Kim, and B.-S. Yun, J. Nat. Prod., 2006, 69, 299; CrossRef e) S. Mo, S. Wang, G. Zhou, Y. Yang, Y. Li, X. Chen, and J. Shi, J. Nat. Prod., 2004, 67, 823. CrossRef
14. M. Yoshida, T. Nakagawa, K. Kinoshita, and K. Shishido, J. Org. Chem., 2013, 78, 1687. CrossRef
15. M. Yoshida, H. Takai, and K. Shishido, Heterocycles, 2010, 82, 881. CrossRef
16. M. Yoshida, C. Sugimura, and K. Shishido, Org. Lett., 2011, 13, 3482. CrossRef
17. For recent representative examples: a) S.-F. Chang, L.-M. Yang, C.-H. Lo, J.-H. Liaw, L.-H. Wang, and S.-J. Lin, J. Nat. Prod., 2008, 71, 87; CrossRef b) K. Tiefenbacher and J. Mulzer, Angew. Chem., Int. Ed., 2008, 47, 2548; CrossRef c) E. D. Coy, L. E. Cuca, and M. Sefkow, J. Nat. Prod., 2009, 72, 1245. CrossRef
18. X.-H. Duan, X.-Y. Liu, L.-N. Guo, M.-C. Liao, W.-M. Liu, and Y.-M. Liang, J. Org. Chem., 2005, 70, 6980. CrossRef
19. a) B. M. Trost, Pure Appl. Chem., 1981, 53, 2357; CrossRef b) J. Tsuji, Pure Appl. Chem., 1982, 54, 197; CrossRef c) B. M. Trost and T. R. Verhoeven, In Comprehensive Organometallic Chemistry, Vol. 8; ed. by G. Wilkinson, F. G. A. Stone, and E. W. Abel, Pergamon: Oxford, 1982, pp. 799–938; d) J. A. Davies, In Comprehensive Organometallic Chemistry II, Vol. 9; ed. by E. W. Abel, F. G. A. Stone, and G. Wilkinson, Pergamon: Oxford, 1995, pp. 323–350.
20. M. Braun and T. Meier, Angew. Chem. Int. Ed., 2006, 45, 6952. CrossRef
21. a) I. Shimizu, T. Yamada, and J. Tsuji, Tetrahedron Lett., 1980, 21, 3199; CrossRef b) T. Tsuda, Y. Chujo, S. Nishi, K. Tawara, and T. Saegusa, J. Am. Chem. Soc., 1980, 102, 6381. CrossRef
22. M. Yoshida, S. Ohno, and K. Shishido, Chem. Eur. J., 2012, 18, 1604. CrossRef
23. For recent representative examples and reviews: a) C. T. Walsh, S. Garneau-Tsodikova, and A. R. Howard-Jones, Nat. Prod. Rep., 2006, 23, 517; CrossRef b) A. Fürstner, Angew. Chem., Int. Ed., 2003, 42, 3582; CrossRef c) G. Balme, Angew. Chem. Int. Ed., 2004, 43, 6238; CrossRef d) P. G. Baraldi, M. C. Nunez, M. A. Tabrizi, E. De Clercq, J. Balzarini, J. Bermejo, F. Estérez, and R. Romagnoli, J. Med. Chem., 2004, 47, 2877; CrossRef e) S. K. Srivastava, S. Shefali, C. N. Miller, M. D. Aceto, J. R. Traynor, J. W. Lewis, and S. M. Husbands, J. Med. Chem., 2004, 47, 6645; CrossRef f) J. L. Sessler, S. Camiolo, and P. A. Gale, Coord. Chem. Rev., 2003, 240, 17; CrossRef g) P. A. Gale, Acc. Chem. Res., 2006, 39, 465. CrossRef
24. M. Yoshida and C. Suguimura, Tetrahedron Lett., 2013, 54, 2082. CrossRef
25. For representative examples: a) P. Bosseray, G. Guillaumet, G. Coudert, and H. Wasserman, Tetrahedron Lett., 1989, 30, 1387; CrossRef b) S. L. Debenedetti, E. L. Nadinic, J. D. Coussio, N. de Kimpe, J. F. Dupont, and J. P. Declercq, Phytochemistry, 1991, 30, 2757; CrossRef c) S. Tsukamoto, H. Kato, H. Hirota, and N. Fusetani, Tetrahedron, 1994, 50, 13583; CrossRef d) G. P. Fagan, C. P. Chapleo, A. C. Lane, M. Myers, C. F. C. Roach, M. R. Stillings, and A. P. Welbourn, J. Med. Chem., 1988, 31, 944; CrossRef e) Y. Satoh, C. Powers, L. M. Toledo, T. J. Kowalski, P. A. Peters, and E. F. Kimble, J. Med. Chem., 1995, 38, 68. CrossRef
26. a) J. R. Labrosse, P. Lhoste, and D. Sinou, Tetrahedron Lett., 1999, 40, 9025; CrossRef b) J.-R. Labrosse, P. Lhoste, and D. Sinou, J. Org. Chem., 2001, 66, 6634. CrossRef
27. N. Dominczak, P. Lhoste, B. Kryczka, and D. Sinou, J. Mol. Catal. A., 2007, 264, 110. CrossRef
28. a) J.-R. Labrosse, P. Lhoste, and D. Sinou, Org. Lett., 2000, 2, 527; CrossRef b) J.-R. Labrosse, P. Lhoste, and D. Sinou, Eur. J. Org. Chem., 2002, 1966; CrossRef c) C. Damez, J.-R. Labrosse, P. Lhoste, and D. Sinou, Tetrahedron Lett., 2003, 44, 557. CrossRef
29. a) S. T. Saengchantara and T. W. Wallace, Nat. Prod. Rep., 1986, 3, 465; CrossRef b) F. M. Dean, Naturally Occurring Oxygen Ring Compounds, Butterworths: London, 1963; c) G. P. Ellis and I. M. Lockhart, Chromans and Tocopherols, Wiley: New York, 1977.
30. M. Yoshida, M. Higuchi, and K. Shishido, Org. Lett., 11, 2009, 4752. CrossRef
31. P. By von Matt, G. C. Lloyd-Jones, A. B. E. Minidis, A. Pfaltz, L. Macko, M. Neuburger, M. Zehnder, H. Rüegger, and P. S. Pregosin, Helv. Chim. Acta, 1995, 78, 265. CrossRef