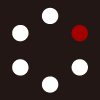
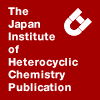
HETEROCYCLES
An International Journal for Reviews and Communications in Heterocyclic ChemistryWeb Edition ISSN: 1881-0942
Published online by The Japan Institute of Heterocyclic Chemistry
e-Journal
Full Text HTML
Received, 29th July, 2013, Accepted, 26th August, 2013, Published online, 28th August, 2013.
DOI: 10.3987/COM-13-S(S)97
■ Synthesis of Macrocyclic Dimer of Cyclic Hexaoxazole and Examination of Its Interaction with Telomeric Oligonucleotide
Keisuke Iida, Gen Tsubouchi, Takahiro Nakamura, and Kazuo Nagasawa*
Biotechnology and Life Science, Graduate School of Engineering, Tokyo University of Agriculture and Technology, 2-24-16, Naka-cho, Koganei, Tokyo 184-8588, Japan
Abstract
A telomestatin-type macrocyclic dimer of cyclic hexaoxazole, termed 6OTD-dimer (5), was synthesized as a new G-quadruplex (G4) ligand by incorporating a second linker into L2H2-6OTD-dimer (3). This macrocyclic-type G4 ligand (5) was found to interact with telomeric oligonucleotide, and stabilized telomeric G4 structure more effectively than did the corresponding non-macrocyclic L2H2-6OTD analog 4.INTRODUCTION
Telomestatin (TMS) (1) is a metabolite isolated by Shin-ya and Seto from Streptomyces anulatus in 2001 (Figure 1).1 It has a characteristic macrocyclic structure with polyoxazole and thiazoline components, and it shows potent telomerase-inhibitory activity with an IC50 value of 5 nM. This inhibitory activity of TMS (1) was suggested to derive from stabilization of telomeric G-quadruplex (G4), a unique DNA structure formed by stacking of G-quartets (Figure 1). TMS (1) has a similar size to the planar G-quartet, and was suggested to stabilize telomeric G4 by end-stacking through π-π interaction as shown in Figure 1.2 Inspired by the structure of TMS (1), we have recently reported a series of macrocyclic hexaoxazole compounds (6OTDs; six-oxazole telomestatin derivatives) as synthetic telomeric G4 ligands (Figure 2).3 These 6OTD ligands were suggested to interact with telomeric G4 in a similar manner to TMS (1). Indeed, the 2:1 complex of L2H2-6OTD (2) and telo24 was detected by means of ESI-mass spectrometry (Figure 2-(a)).3f Moreover, 6OTD-dimer with an appropriate linker length was found to effectively stabilize various lengths of telomeric DNAs: telo24, telo48, telo72 and telo96.4 A 1:1 complex of telo24 and L2H2-6OTD-dimer (3) was observed by ESI-mass spectrometry, suggesting a sandwich-type interacting mode (Figure 2-(b)).3a,3c,3d Based upon these observations, we envisaged that telomeric G4 structure might be irreversibly fixed by macrocyclization of 6OTD-dimer in the presence of telomeric DNA (Figure 3). This could be a powerful tool for investigating regulation of telomeric G4 functions,
as well as for elucidation of telomere-related factors.5 In this context, we first planned to synthesize macrocycle 5 by cyclization of 6OTD-dimer 4, as a first step towards examining the feasibility of macrocyclization of 6OTD-dimer in the presence of telomeric DNA. Thus, in the present study, we synthesized macrocyclic L2H2-6OTD-dimer 5, and evaluated its interaction with telo24 using CD spectroscopy and FRET melting assay.
RESULTS AND DISCUSSION
Synthesis of macrocyclic L2H2-6OTD-dimer (5) was planned by macrocyclization of 8 using ring-closing metathesis (RCM) reaction (Scheme 1).6,7 Thus, the Boc group in diamine 64d was selectively deprotected with hydrochloric acid, and the resulting amine was reacted with 4-pentenoic acid in the presence of DMT-MM8 to give bis-amide 7 in 99% yield from 6. After conversion of two Nosyl (Ns) groups into Boc groups by treatment with thiophenol followed by (Boc)2O, the resulting dimer 8 was subjected to the RCM reaction using first-generation Grubbs catalyst (50 mol%) to give macrocycle 9 as an 1:1 mixture of E/Z isomers. After hydrogenation of the double bond of these isomers with dipotassium azodicarboxylate, the Boc group was removed with TFA to give macrocyclic L2H2-6OTD-dimer (5) in 23% yield from 9. The structure of 5 was confirmed by the 1H and 13C NMR spectra as well as ESI-mass spectrum. We also prepared L2Peta2-6OTD-dimer (4) from 8 for comparison of its interaction of telo24 with that of 5. The two olefins in 8 were hydrogenated with hydrogen in the presence of Raney Nickel, and the two Boc groups were removed with TFA to give 4 in 52% yield from 8.
With the macrocyclic L2H2-6OTD-dimer (5) and the corresponding non-macrocyclic 6OTD-dimer 4 in hand, the interaction of these ligands with telomeric oligonucleotide telo24 was investigated by CD titration analysis. Telomeric oligonucleotide is known to form G4 structures with multiple topologies, e.g. parallel, anti-parallel and hybrid types. Each of these G4 conformations has characteristic CD signals.9
For example, anti-parallel G4, formed in Na+ solution, has a positive Cotton effect at 295 nm and a negative Cotton effect at 260 nm. On the other hand, the spectra of telomeric oligonucleotide in K+ solution shows a positive Cotton effect at 290 nm, a shoulder at 268 nm, and a negative Cotton effect at 240 nm, which are consistent with a hybrid-type G4 structure. In the CD analysis, telo24 in K+ solution was titrated with the ligand 4 or 5 from 0 to 5 equivalents, each resulting mixture was incubated for 12 h at room temperature, and then the CD spectra were measured. We observed characteristic spectral changes at 295 and 260 nm with both 4 and 5 (Figure 4). Thus, these ligands were suggested to interact with telo24 in a similar manner, and to induce conformational change from hybrid to anti-parallel type G4 structure.
Since both 4 and 5 were confirmed to interact with telo24, we compared their abilities to stabilize telomeric G4 structure by using a fluorescence resonance energy transfer (FRET) melting assay.10 For the assay, we used fluorescence-labeled telo21 {5’-FAM-d[GGG(TTAGGG)3]-TAMRA-3’}, which corresponds in size to telo24. The FRET melting assay was carried out in the presence of KCl (60 mM), and we used five equivalents of the ligands (1.0 µM) with respect to telo21.11 Under these conditions, the ΔTm values of 4 and 5 were 8.0 °C and 11.9 °C, respectively (Table 1), so that 5 was found to be more effective for stabilization of telo24 than 6OTD-dimer 4.
We had initially expected that macrocyclic L2H2-6OTD-dimer (5) would interact poorly with telomeric G4 because of its less flexible structure compared to 6OTD-dimer 4, suggesting that complex formation with telomeric G4 would be less favorable. Contrary to our expectation, however, macrocycle 5 interacted well with telomeric G4. Although the complexation pathway of telomeric G4 with macrocycle 5 is not clear, we speculate that it may be as shown in Figure 5. Thus, telomeric oligonucleotide may form G4 structure IV in the presence of K+ from random coil I through the hairpin-type and triplex-type intermediates II and III, respectively, which are in equilibrium with each other.12 Direct complexation of macrocycle 5 with the G4 structure IV of telo24 seems unlikely, and one of the unfolded structures of I or II seems more likely to be involved, followed by refolding into anti-parallel type G4 structure VIII through possible intermediates V-VII. The reason why telomeric G4 is stabilized more effectively by 5 than by the corresponding dimer 4 may be that formation of the telomeric G4 complex with 5 would be entropically favored.
In conclusion, we have synthesized macrocyclic L2H2-6OTD-dimer (5) by installing an additional linker at the open side of 6OTD-dimer 3. Interestingly, 5 was found to interact efficiently with telomeric oligonucleotide telo24 to induce anti-parallel type G4 structure. The resulting complex of telomeric G4 with 5 was more stable than the corresponding complex with 6OTD-dimer 4. Elucidation of the interaction pathway of 5 and telo24 is in progress.
EXPERIMENTAL
General
Flash chromatography was performed on Silica gel 60 (spherical, particle size 0.040 ~ 0.100 mm; Kanto) and chromatorex NH–DM1020 (Fuji Silysia Chemical Ltd.). Optical rotations were measured on a JASCO P 2200 polarimeter, using the sodium lump (589 nm). 1H and 13C NMR spectra were recorded on JEOL JNM-ECX 300, 400 and 500. The spectra are referenced internally according to residual solvent signals of CDCl3 (1H NMR; δ = 7.26 ppm, 13C NMR; δ = 77.0 ppm), DMSO-d6 (1H NMR; δ = 2.50 ppm, 13C NMR; δ = 39.5 ppm). Data for NMR are recorded as follows; chemical shift (δ, ppm), multiplicity (s, singlet; d, doublet; t, triplet; m, multiplet; br, broad), integration, coupling constant (Hz). Data for 13C NMR are reported in terms of chemical shift (δ, ppm). Mass spectra were recorded on JEOL JMS-T100LC spectrometer with ESI-MS mode using MeOH.
Synthesis of 7: A solution of 6 (135 mg, 67.4 µmol) in MeOH:THF (1:1, 20 mL) was added 12 M HCl (4 mL), and mixture was stirred at room temperature for 4 h. The resulting mixture was concentrated in vacuo, and the residue was dissolved in DMF (5 mL). The DMF solution was added N-methylmorpholine (148 µL, 1346 μmol), DMT-MM (369 mg, 1350 µmol) and 4-pentenoic acid (68 µL, 674 μmol). After the mixture was stirred at room temperature for 10 h, the reaction mixture was added H2O, and aqueous layer extracted with CHCl3. The extracts were dried over MgSO4, filtered, and concentrated in vacuo. The residue was purified by column chromatography (EtOAc:MeOH = 9:1) to give 7 (132 mg, 67.1 μmol, 99%).
Spectral data for 7: [α]25D −19.7 (c 0.8, CHCl3); 1H NMR (400 MHz, CDCl3) δ 8.48-8.42 (m, 4H), 8.34 (d, J = 7.4, 2H), 8.27-8.21 (m, 12H), 8.16 (d, J = 7.4, 2H), 7.89 (d, J =6.9, 2H), 7.60 (t, J = 7.8, 2H), 5.97-5.92 (m, 2H), 5.79-5.68 (m, 2H), 5.44-5.32 (m, 4H), 5.01-4.88 (m, 4H), 3.58-3.04 (m, 20H), 2.34-2.16 (m, 8H), 2.15-1.87 (m, 8H), 1.66-0.75 (m, 16H); 13C NMR (100 MHz, CDCl3) δ 172.4, 164.9, 164.2, 159.8, 159.5, 156.1, 156.0, 154.8, 154.7, 147.6, 141.1, 141.0, 139.7, 139.5, 138.7, 137.2, 136.7, 136.6, 135.3, 132.7, 131.7, 130.8, 130.7, 130.2, 129.5, 129.2, 125.3, 115.3, 77.2, 70.2, 69.5, 63.9, 53.6, 50.8, 47.7, 47.1, 45.3, 44.4, 39.0, 35.7, 34.5, 33.5, 29.6, 28.6, 26.9, 21.8, 20.4; HRMS (ESI, M+Na) calcd for C88H88N22O30S2Na 1987.5478, found 1987.5496.
Synthesis of 8: A solution of 7 (132 mg, 67.1 μmol) in DMF (5 mL) was added K2CO3 (931 mg, 6710 μmol) and PhSH (73 µL, 671 μmol), and the mixture was stirred at room temperature for 1 h. The reaction mixture was added (Boc)2O (293 mg, 1342 µmol), and the mixture was stirred at room temperature for 14 h. The reaction mixture was added H2O, and aqueous layer was extracted with CHCl3. The extracts were dried over MgSO4, filtered, and concentrated in vacuo. The residue was purified by column chromatography (EtOAc:MeOH = 9:1) to give 8 (111 mg, 61.8 µmol, 92%).
Spectral data for 8: [α]25D −10.1 (c 1.4, CHCl3); 1H NMR (400 MHz, CDCl3) δ 8.60-8.50 (m, 4H), 8.25-8.19 (m, 12H), 5.93-5.86 (br, 2H), 5.83-5.69 (m, 2H), 5.46-5.37 (m, 4H), 5.02-4.89 (m, 4H), 3.64-3.06 (m, 20H), 2.37-2.16 (m, 8H), 2.15-1.89 (m, 8H), 1.62-0.79 (m, 26H); 13C NMR (100 MHz, CDCl3) δ 172.3, 164.7, 162.5, 159.9, 159.7, 156.0, 154.7, 154.6, 140.9, 139.2, 138.5, 137.2, 136.9, 136.8, 130.9, 129.6, 115.3, 79.3, 77.2, 70.5, 69.7, 47.9, 47.6, 46.8, 46.7, 39.1, 36.5, 35.8, 34.6, 29.6, 28.7, 28.4, 21.9; HRMS (ESI, M+Na) calcd for C86H98N20O24Na 1817.6961, found 1817.6918.
Synthesis of 9: A solution of 8 (103 mg, 57.5 µmol) in CH2Cl2 (12 mL) was added benzylidene-bis(tricyclohexylphosphine)-dichlororuthenium (24 mg, 28.8 µmol), and mixture was stirred at room temperature under nitrogen gas (balloon). After 23 h, the reaction mixture was added brine, and extracted with CH2Cl2. The organic layer was dried over MgSO4, filtrated, and concentrated in vacuo. The residue was purified by column chromatography on silica gel (EtOAc:MeOH = 9:1) to give 5 as a 1:1 miture of E/Z isomers by 1H NMR (39 mg, 22.2 µmol, 37%).
Synthesis of reduced compound 10: Dipotassium azodicarboxylate (12 mg, 59.4 µmol) was added to a solution of 9 (35 mg, 19.8 µmol) in MeOH (2 mL). Glacial AcOH (14 µL) was added and the reaction mixture was heated to 40 °C open to the atmosphere. As the yellow color of the solution started to fade, more DAPA (12 mg) and AcOH (14 µL) were added. This addition procedure was repeated several times over the course of 15 h. Upon completion of the reaction, the reaction mixture was cooled and then a saturated aqueous solution of NaHCO3 was added. The reaction mixture was added H2O, and aqueous layer was extracted with CHCl3. The extracts were dried over MgSO4, filtered, and concentrated in vacuo. After evaporation of the solvent, the clear residue was purified by preparative TLC (CHCl3:EtOAc:MeOH = 3:2:1) to give reduced compound 10 (11 mg, 30%).
Spectral data for reduced compound 10: [α]25D −3.4 (c 0.9, CHCl3); 1H NMR (400 MHz, CDCl3) δ 8.61-8.49 (m, 4H), 8.28-8.17 (m, 12H), 6.27-6.04 (br, 2H), 5.47-5.38 (m, 4H), 3.55-3.45 (m, 8H), 3.33-3.08 (m, 12H), 2.36-1.90 (m, 12H), 1.58-0.82 (m, 42H); 13C NMR (100 MHz, CDCl3) δ 173.2, 172.6, 164.9, 164.8, 164.7, 159.8, 159.6, 156.0, 155.5, 155.3, 154.7, 154.6, 130.8, 130.7, 129.7, 129.5, 79.4, 77.2, 70.5, 70.3, 69.6, 47.9, 47.7, 46.9, 39.1, 39.0, 36.3, 34.7, 29.7, 28.8, 28.6, 28.4, 27.8, 25.4, 21.9, 21.7; HRMS (ESI, M+Na) calcd for C84H96N20O24Na 1791.6804, found 1791.6796.
Synthesis of 5:A solution of reduced compound 10 (8 mg, 4.3 µmol) in CH2Cl2-TFA (2:1, 1.5 mL) was stirred at room temperature for 4 h. The resulting mixture was concentrated in vacuo. The residue was purified by NH-preparative TLC (CHCl3-EtOAc-MeOH = 3:2:1) to give 5 (6 mg, 3.2 µmol, 75%).
Spectral data for 5: [α]25D +5.2 (c 0.6, DMSO); 1H NMR (500 MHz, DMSO-d6) δ 9.13-9.10 (m, 8H), 8.93 (s, 2H), 8.91 (s, 2H), 8.35 (d, J = 7.45 Hz, 6H), 8.24 (d, J = 6.87 Hz, 2H), 7.64 (t, J = 5.73, 2H), 5.51-5.41 (m, 4H), 3.60-3.42 (m, 8H), 3.05-2.78 (m, 12H), 2.20-1.77 (m, 12H), 1.65-0.70 (m, 24H); 13C NMR (125 MHz, DMSO-d6) δ 172.7, 165.3, 165.1, 159.6, 159.5, 156.5, 155.4, 143.4, 142.8, 142.1, 136.9, 130.7, 130.6, 129.4, 129.3, 79.6, 70.7, 70.2, 66.3, 48.2, 47.7, 47.0, 38.9, 36.2, 29.7, 29.1, 26.1, 26.0, 21.7; HRMS (ESI, M+H) calcd for C74H81N20O20 1569.5936, found 1569.5966.
Synthesis of reduced compound 11: A solution of 8 (52 mg, 28.8 µmol) in MeOH (10 mL) was added Raney Ni and the reaction mixture was stirred at room temperature under hydrogen gas (balloon). After 24 h, the reaction mixture was filtered through a pad of celite, and filtrates were concentrated in vacuo to give reduced compound 11 (43 mg, 23.7 µmol, 82%).
Spectral data for reduced compound 11: [α]25D −6.8 (c 0.6, CHCl3); 1H NMR (400 MHz, CDCl3) δ 8.62-8.52 (m, 4H), 8.24-8.20 (m, 12H), 5.86-5.79 (br, 2H), 5.47-5.38 (m, 4H), 3.55-3.46 (m, 8H), 3.35-3.11 (m, 12H), 2.36-1.91 (m, 12H), 1.60-1.28 (m, 42H), 0.85 (t, J = 7.3, 6H); 13C NMR (100 MHz, CDCl3) δ 173.3, 164.9, 164.8, 159.9, 159.7, 156.1, 156.0, 155.5, 155.3, 154.7, 154.6, 140.9, 140.8, 139.2, 138.5, 136.9, 136.8, 79.4, 77.2, 70.5, 70.3, 70.2, 69.7, 69.4, 47.9, 47.7, 46.7, 39.1, 36.5, 34.6, 31.9, 29.7, 28.8, 28.4, 27.8, 22.7, 22.4, 21.9, 14.1, 13.7; HRMS (ESI, M+Na) calcd for C86H102N20O24Na 1821.7274, found 1821.7309.
Synthesis of 4: A solution of reduced compound 11 (31 mg, 17.1 µmol) in CH2Cl2-TFA (1:1, 10.0 mL) was stirred at room temperature for 5 h. The resulting mixture was concentrated in vacuo. The residue was purified by NH-silica preparative TLC (CHCl3-EtOAc-MeOH = 3:2:1) to give 4 (18 mg, 10.9 µmol, 64%).
Spectral data for 4: [α]25D +8.7 (c 1.1, DMSO); 1H NMR (400 MHz, DMSO-d6) δ 9.16-9.10 (m, 8H), 8.95-8.92 (m, 4H), 8.43-8.34 (m, 4H), 7.83-7.74 (m, 2H), 5.49-5.38 (m, 4H), 3.43-3.36 (m, 8H), 3.04-2.95 (m, 4H), 2.59-2.41 (m, 8H), 2.16-1.89 (m, 12H), 1.51-1.03 (m, 24H), 0.77 (dt, J = 2.3, 7.33 Hz, 6H); 13C NMR (100 MHz, DMSO-d6) δ 172.8, 165.5, 165.4, 159.6, 156.5, 155.4, 143.4, 142.7, 142.0, 137.0, 130.7, 129.4, 71.0, 70.3, 50.0, 49.7, 49.5, 48.4, 39.0, 36.0, 34.5, 34.2, 30.2, 29.6, 28.4, 22.6, 22.0, 14.4; HRMS (ESI, M+Na) calcd for C76H86N20O20Na 1621.6225, found 1621.6233.
CD experiments
CD spectra were recorded with a JASCO-810 spectropolarimeter (Jasco, Easton, MD) in a quartz cell of 1 mm optical path length and with an instrument scanning speed of 100 nm min-1 and a response time of 1 s, over the wavelength range of 220–320 nm. CD spectra are representative of ten averaged scans taken at 25 °C. The nucleotide, telo24 (5’-d[TTAGGG]4-3’, purified, FASMAC Co., Ltd. Kanagawa, Japan), was dissolved as a stock solution (1 mM) in MilliQ water to be used without further purification. The nucleotide was further diluted with KCl (100 mM) and Tris–HCl buffer (pH 7.2, 50 mM) from 1 mM stock solutions to give a concentration of 10 µM. The solution was annealed by heating at 96 °C for 2 min and then allowed to cool slowly to room temperature, and titrated into the oligonucleotide samples up to 5 mol equiv using ligands of 5 or 6. All CD spectra are baseline-corrected for signal contributions due to the buffer for the samples containing 5 and 6.
FRET melting assay
It was performed with an excitation wavelength of 470–505 nm and a detection wavelength of 523–543 nm and use of the DNA Engine Opticon 2 Real-Time Cycler PCR detection system (BioRad). The dual fluorescently labeled oligonucleotides Flu-ss-telo21 {5’-FAM-d[GGG(TTAGGG)3]-TAMRA-3’} and Flu-ss-ds-26-mer {5’-FAM-d[(TA)2GC(TA)2T6(TA)2GC(TA)2]-TAMRA-3’} were used in this protocol. The donor fluorophore was 6-carboxyfluorescein (FAM) and the acceptor fluorophore was 6-carboxytetramethylrhodamine (TAMRA). All purified nucleotides (Sigma Genosys) were dissolved as stock solutions (100 µM) in MilliQ water to be used without further purification. Further dilutions of the oligonucleotides were performed with potassium cacodylate buffer (pH 7.4, 60 mM), and FRET experiments were carried out with oligonucleotide solutions (0.4 µM). Dual-labeled DNA was annealed by heating at 96 °C for 2 min and was allowed to cool to room temperature. Ligands of 5 or 6 were prepared as DMSO stock solutions (10 mM) and diluted to 2 µM with FRET (cacodylate) buffer. Next, the annealed DNA (20 µL, 0.4 µM) and the compound solution (20 µL, 2 µM) were distributed across 96-well plates (Bio-Rad), with a total volume of 40 µL, with labeled oligonucleotide (0.2 µM) and the compound (1.0 µM). The plates were incubated at 25 °C for 12h. Subsequent experiments used the following temperature procedure in RT-PCR, finishing as follows : 25 °C for 30 min, then a stepwise increase of 1 °C every minute from 25 °C until 99 °C. During the procedures, we measured the FAM fluorescence after each step. The change in melting temperature at 1.0 µM compound concentration—ΔTm (1.0 µM)—was calculated from at least three experiments by subtraction of the blank from the averaged melting temperature of each compound (1.0 µM).
ACKNOWLEDGEMENTS
This work was supported in part by a Grant-in-Aid for Scientific Research (B) from JSPS (No. 23310158), a Grant-in Aid for Challenging Exploratory Research from JSPS (No. 21655060), and a Grant-in-Aid for Scientific Research on Innovative Areas (No. 23105013) from The Ministry of Education, Culture, Sports, Science and Technology, Japan. K. N. is grateful for financial support from the Mukai Science and Technology Foundation, Tokyo, Japan, and Mochida Memorial Foundation for Medical and Pharmaceutical Research, Tokyo, Japan. K. I. and T. N. are grateful for financial support in the form of JSPS Predoctoral Fellowships for Young Scientists.
References
1. K. Shin-ya, K. Wierzba, K. Matsuo, T. Ohtani, Y. Yamada, K. Furihata, Y. Hayakawa, and H. Seto, J. Am. Chem. Soc., 2001, 123, 1262. CrossRef
2. Involvement of two molecules of TMS was indicated by the results of molecular dynamics calculations, mass spectrometry, SPR analysis, and CD titration experiments, see: (a) M.-Y. Kim, H. Vankayalapati, K. Shin-ya, K. Wierzba, and L. H. Hurley, J. Am. Chem. Soc., 2002, 124, 2098; CrossRef (b) F. Rosu, V. Gabelica, K. Shin-ya, and E. D. Pauw, Chem. Commun., 2003, 2702; CrossRef (c) E. M. Rezler, J. Seenisamy, S. Bashyam, M.-Y. Kim, E. White, W. D. Wilson, and L. H. Hurley, J. Am. Chem. Soc., 2005, 127, 9439. CrossRef
3. (a) M. Tera, Y. Sohtome, H. Ishizuka, T. Doi, M. Takagi, K. Shin-ya, and K. Nagasawa, Heterocycles, 2006, 69, 505; CrossRef (b) M. Tera, H. Ishizuka, M. Takagi, M. Suganuma, K. Shin-ya, and K. Nagasawa, Angew. Chem. Int. Ed., 2008, 47, 5557; CrossRef (c) S. Majima, M. Tera, K. Iida, K. Shin-ya, and K. Nagasawa, Heterocycles, 2011, 82, 1345; CrossRef (d) K. Iida, S. Majima, T. Ohtake, M. Tera, K. Shin-ya, and K. Nagasawa, Heterocycles, 2012, 84, 401; CrossRef (e) T. Nakamura, K. Iida, M. Tera, K. Shin-ya, H. Seimiya, and K. Nagasawa, ChemBioChem, 2012, 13, 774; CrossRef (f) K. Iida, G. Tsubouchi, T. Nakamura, S. Majima, H. Seimiya, and K. Nagasawa, Med. Chem. Commun., 2013, 4, 260; CrossRef Rice and co-workers independently reported the same class of compounds as G4 ligands, see; (g) G. S. Minhas, D. S. Pilch, J. E. Kerrigan, E. J. LaVoie, and J. E. Rice, Bioorg. Med. Chem. Lett., 2006, 16, 3891; CrossRef (h) S. G. Rzuczek, D. S. Pilch, E. J. LaVoie, and J. E. Rice, Bioorg. Med. Chem. Lett., 2008, 18, 913; CrossRef (i) M. Satyanarayana, S. G. Rzuczek, E. J. LaVoie, D. S. Pilch, A. Liu, L. F. Liu, and J. E. Rice, Bioorg. Med. Chem. Lett., 2008, 18, 3802; CrossRef (j) M. Satyanarayana, Y.-A. Kim, S. G. Rzuczek, D. S. Pilch, A. A. Liu, L. F. Liu, J. E. Rice, and E. J. LaVoie, Bioorg. Med. Chem. Lett., 2010, 20, 3154. CrossRef
4. (a) K. Iida, M. Tera, T. Hirokawa, K. Shin-ya, and K. Nagasawa, Chem. Commun., 2009, 6481; CrossRef (b) K. Iida, M. Tera, T. Hirokawa, K. Shin-ya, and K. Nagasawa, J. Nucleic Acids, 2010, 217627; (c) Y. Amemiya, Y. Furunaga, K. Iida, M. Tera, K. Nagasawa, K. Ikebukuro, and C. Nakamura, Chem. Commun., 2011, 47, 7485; CrossRef (d) K. Iida, S. Majima, T. Nakamura, H. Seimiya, and K. Nagasawa, Molecules, 2013, 18, 4328. CrossRef
5. (a) T. de Lange, Genes Dev., 2005, 19, 2100; CrossRef (b) W. Palm and T. de Lange, Annu. Rev. Genet., 2008, 42, 301. CrossRef
6. For a recent review see: S. Monfette and D. E. Fogg, Chem. Rev., 2009, 109, 378. CrossRef
7. (a) G. C. Fu, S. T. Nguyen, and R. H. Grubbs, J. Am. Chem. Soc., 1993, 115, 9856; CrossRef (b) P. Schwab, M. B. France, J. W. Ziller, and R. H. Grubbs, Angew. Chem. Int. Ed., 1995, 34, 2039. CrossRef
8. M. Kunishima, C. Kawachi, F. Iwasaki, K. Terao, and S. Tani, Tetrahedron Lett., 1999, 40, 5327. CrossRef
9. (a) S. Paramasivan, I. Rujan, and P. H. Bolton, Methods, 2007, 43, 324; CrossRef (b) A. I. Karsisiotis, N. M. Hessari, E. Novellino, G. P. Spada, A. Randazzo, and M. Webba da Silva, Angew. Chem. Int. Ed., 2011, 50, 10645. CrossRef
10. (a) J. L. Mergny and J.-C. Maurizot, ChemBioChem, 2001, 2, 124; CrossRef (b) A. D. Cian, L. Guittat, M. Kaiser, B. Sacca, S. Amrane, A. Bourdoncle, P. Alberti, M. P. Teulade-Fichou, L. Lacroix, and J. L. Mergny, Methods, 2007, 42, 183. CrossRef
11. Incubation was conducted for 12 h at room temperature.
12. (a) T. Mashimo, H. Yagi, Y. Sannohe, A. Rajendran, and H. Sugiyama, J. Am. Chem. Soc., 2010, 132, 14910; CrossRef (b) A. Y. Q. Zhang and S. Balasubramanian, J. Am. Chem. Soc., 2012, 134, 19297. CrossRef