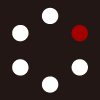
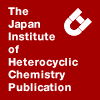
HETEROCYCLES
An International Journal for Reviews and Communications in Heterocyclic ChemistryWeb Edition ISSN: 1881-0942
Published online by The Japan Institute of Heterocyclic Chemistry
e-Journal
Full Text HTML
Received, 25th June, 2013, Accepted, 30th July, 2013, Published online, 2nd August, 2013.
DOI: 10.3987/COM-13-S(S)46
■ Synthesis of Tetrahydroquinolines through Intramolecular Carbolithiation Reactions
Oihane García-Calvo, Unai Martínez-Estíbalez, Esther Lete, and Nuria Sotomayor*
Departamento de Química Orgánica, Facultad de Ciencia y Tecnologia, Universidad del País Vasco, Barrio Sarriena s/n. Leioa (Bizkaia) Bilbao 48940, Spain
Abstract
Cyclization of aryllithiums obtained by iodine-lithium exchange reaction on N-alkenyl 2-iodoanilines allows the synthesis of 4- and 2,4-substituted tetrahydroquinolines. However, the alkene has to be substituted with a stabilizing group for the resulting organolithium to favor the intramolecular carbolithiation. When (–)-sparteine (4) or (+)-sparteine surrogate (5) are used as chiral ligands low levels of enantioselection are obtained. The carbolithiation is completely diastereoselective when an enantiomerically pure precursor is used.INTRODUCTION
The carbolithiation reaction of alkenes and alkynes has attracted considerable interest among synthetic organic chemists, as it offers an attractive pathway for the efficient construction of new carbon-carbon bonds with the possibility of introducing further functionalization by trapping the reactive organolithium intermediates with electrophiles. The intramolecular variant of this reaction has been applied mainly with alkyl- and alkenyllithiums, though there are also some examples of cycloisomerization of alkenyl substituted aryllithiums, generated by metal-halogen exchange.1 In particular, intramolecular carbolithiation is well suited for the diastereoselective construction of five-membered rings through a 5-exo-trig cyclization process, as has been shown in the synthesis of both carbocycles2 and heterocycles.3 When alkenes are used, up to two contiguous stereogenic centers may be generated, that may be controlled by using chiral ligands for lithium, and so opening new opportunities for its application in asymmetric synthesis. In this context, the chiral bidentate amine (–)-sparteine4 has been used in the formation of five membered rings with high levels of stereocontrol.5 However, to achieve a intramolecular 6-exo carbolithiation, the alkene should carry a stabilizing group for the resulting organolithium or a leaving group α to the double bond (SN2′ pathway). However, even in those cases, formation of six-membered cycles is not general.6 In this context, we have shown that intramolecular carbolithiation reactions of 2-alkenyl substituted N-benzylpyrroles constitutes an efficient route to pyrrolo[1,2-b]isoquinolines, though in this case, besides activation of the alkene, the use of mesityllithium (MesLi) as metalating agent is required to avoid 1,2 or 1,4-addition of the alkyllithium to the unsaturated system. The procedure is applicable to the construction of six, seven and eight membered rings, thus opening new routes to benzazepines, and benzazocines. Although the use of (–)-sparteine as a chiral ligand led to low levels of enantioselection, enantiomerically pure hexahydropyrrolo[1,2-b]isoquinolines could be synthesized by applying this protocol to the related pyrrolidines derived from proline, as the reactions proceed with complete diastereoselectivity.7
On the other hand, we were interested in the application of this type of intramolecular carbolithiation to the construction of the tetrahydroquinoline framework, present in many natural products and biologically active compounds.8 Therefore, the development of new synthetic procedures for the enantioselective synthesis of these heterocycles continues to be an intensely investigated field.9 More precisely, some 2-carboxytetrahydroquinoline derivatives are well-known antagonists of glycine at the NMDA receptor, and have received much attention as potential candidates for the treatment of nicotine craving.10 On the other hand, 2-aryloxymethyltetrahydroquinolines have been identified as PPARα agonists,11 while the 2-acyloxymethyl derivatives are enzyme inhibitors that constitute an important target in the therapy of Alzheimer’s disease.12
In this context, we have previously reported 2,4-disubstituted tetrahydroquinolines can been obtained through an intramolecular carbolithiation of aryllithiums generated from N-alkenyl-substituted o-iodoanilines when the alkene is substituted with an amide group. When the reaction is carried out in the presence of (–)-sparteine the substitution pattern of the carboxamide was relevant, as when the N,N-diethyl derivative was used the tetrahydroquinolines were obtained with moderate diastereoselectivity (in favor of the 2,4-trans isomer) and each of the diastereomers with low enantiomeric excess under all the conditions tested. However, with the Weinreb amide as substrate and n-BuLi as metalating agent at –95 ºC, the cyclization proceeded rapidly (10 minutes) with reasonable diastereoselectivity (dr 33:67) and excellent ee for each of the isolated diastereomers (94% ee and 92% ee) (Scheme 1).13
With these precedents in mind, we wanted to study the scope of this type of carbolithiation reaction for the synthesis of 2,4-disubstituted tetrahydroquinoline derivatives 2. For this purpose, we chose a series of butenylanilines 1,14 with different substitution patterns in the alkene and α to the nitrogen atom (Scheme 2), that would lead to tetrahydroquinolines 2 bearing different substituents on C-2. Here we present a full account of our investigations.
RESULTS AND DISCUSSION
We first studied the intramolecular carbolithiation of N-alkenyl substituted 2-iodoanilines 1a-c (Scheme 3). The reactions were carried out under the usual conditions, using 2 equivalents of the organolithium at low temperature in THF, with or without TMEDA (Table 1). As could be expected from our previous results,7,13 iodine-lithium exchange was fast at low temperature when 1a was treated with n-BuLi or t-BuLi, but the unsubstituted alkene was not reactive enough to participate in the cyclization, even in the presence of TMEDA (entries 2, 5) or when the reaction is allowed to warm up to room temperature for longer periods of time (entries 1, 3). In all cases, the deiodinated aniline 3a was isolated in high yield. On the other hand, cyclization of 2-iodoanilines 1b,c took place efficiently at low temperature in short reaction times (10 min) to afford the corresponding tetrahydroquinolines 2b,c. Cylization was fast even in the absence of TMEDA, but generally better yields were obtained with this additive, as has been described in related reactions.7,15 When n-BuLi or t-BuLi were used as metalating agents, 1,4 addition to the enamide moiety was observed as competitive reaction, lowering the isolated yield of 2b,c (entries 6-9, 11-14). The use of mesityllithium (MesLi), a more bulky and less nucleophilic reagent for the iodine–lithium exchange reaction,7 avoided these side reactions improving the isolated yields of tetrahydroquinolines 2b,c (entries 10, 15).
Our next step was to study the possibility of performing the carbolithiation reactions in an enantioselective fashion in the presence of a chiral ligand for lithium. In this context, as stated before, the chiral bidentate amine (–)-sparteine (4)4 has been used in the formation of five membered rings with high levels of stereocontol.5 Thus, 2-iodoanilines 1b and 1c were reacted with n-BuLi or t-BuLi in the presence of this chiral diamine. Several reaction conditions were used, some of which are summarized on Table 2 (Scheme 4). The reactions were carried out in toluene, to favor the coordination of the organolithium with the amine. For this reason, MesLi could not be used for these reactions. Although the preparation of MesLi from mesityl bromide in THF is straightforward, we were unable to generate it efficiently using toluene or hexane as solvents. In all cases, the tetrahydroquinolines 2b,c were obtained in moderate yields, but with low enantiomeric purity. No significant improvement was observed when different experimental procedures regarding the addition of reagents were used (Procedures A,16 B17 or C).
In our previous work13 we had observed a strong influence of the amide moiety on the enantioselection, obtaining excellent when Weinreb amides were used. However, no relevant difference on the behavior of 1b and 1c was observed under the conditions used. The enantioselection could be slightly improved when the temperature was lowered, and with shorter reaction times, although lowering the yield, with aniline 1c (entry 10). The use of other solvents, as pentane / Et2O (9/1 vol),18 gave similar results. A large set of chiral ligands have been studied to effect asymmetric intramolecular carbolithiations, although (–)-sparteine (4) generally gave the best results.17 In this context, we chose diamine 5, as it has been described to behave as an efficient (+)-sparteine surrogate in carbolithiation reactions.19 However, when it was used with aniline 1b, tetrahydroquinoline 2b was obtained with low ee (4%), in favor of the opposite enantiomer. Having obtained this low enantioselectivity level, no efforts were made to determine the configuration of the stereogenic centre, and no other ligands were tested.
We next focused on the synthesis on 2,4-substituted tetrahydroquinolines, starting from α-substituted alkenyl anilines. We first checked the possibility of performing an intramolecular carbolithiation reaction in the presence of an ester moiety. Not surprisingly, the reaction of amino ester 1d under various conditions led to a complex mixture of products. When n-BuLi was used (Scheme 5) indole 6 could be isolated as the major compound in low yield (20%), which would result from cyclization of the aryllithium with the ester moiety, and subsequent addition of n-BuLi. Under similar conditions, 1e led only to complex mixtures.
In view of these results, we chose a protected hydroxymethyl group. Thus, anilines 1f-h were treated with t-BuLi or n-BuLi under various reaction conditions (Scheme 6, Table 3). As in the previous examples, the cyclization did not take place onto the unsubstituted alkene of 1f, leading to the formation of deiodinated 3b (Table 3, entries 1,2). On the other hand, cyclization of 1g took place smoothly at –78 ºC to afford a mixture of 2,4-cis and 2,4-trans substituted tetrahydroquinolines 2g, with poor diastereoselectivity and moderate yield (entry 3). Various conditions were tested in order to improve the yield and the stereoselectivity. Loweing the temperature and the reaction time led to a slight improvement, but still in a range of 40:60 (entries 4, 5). Similar results were obtained with 1h (entries 8, 9). The use of t-BuLi/TMEDA gave the best results in terms of diastereoselectovity, but still in the 30:70 range. In the case of 1h, the use of MesLi avoided the formation of 1,4-addition by-products, but did not improve the yields or diastereoselectivities (entry 12 vs. 11). For that reason, it was not used with 1g. The diastereomeric pairs could not be separated by chromatographic methods, and have been characterized from the spectroscopic data of the mixture, and by comparison with the spectroscopic data of related 2,4-disubstituted tetrahydroquinolines previously described by our group, that bear a phenyl group on C2 (Scheme 1).13 Finally, we carried out the reactions in the presence of (–)-sparteine (4). In agreement with our previous results, the diastereoselectivity was inverted with respect to TMEDA, obtaining in this case the trans diastereomer as the major product. Unfortunately, the enantiomeric ratio of these diasteromeric pairs could not be determined by chiral HPLC.
In view of these results, we decided to use an enantiomerically pure 2-iodoaniline to perform a diastereoselective intramolecular carbolithiation. Thus, treatment of 1i with n-BuLi gave the tetrahydroquinoline 2i, as a single (2R,4S)-cis isomer.20 The stereochemical course of these anionic cyclizations is a consequence of a rigid transition state in which the lithium atom is coordinated to the remote π-bond. Therefore, attack of the arylllithium may take place by the Re face the alkene, as shown in scheme 7, through a pseudo-chair transition state in which both substituents would have a pseudo-equatorial disposition. The formation of the 2,4-cis substituted tetrahydroquinoline under these conditions is in agreement with the results obtained with a benzyloxymethyl substituent at C-2 (see Table 3) or with a phenyl group.13 In this case the complete diastereoselectivity could be attributed to the steric bulk of the dimethyl-1,3-dioxolanyl substituent. NOESY and COSY experiments confirmed the stereochemistry of 2i The most significant NOESY results obtained are shown in Scheme 8. In particular, 2D NOESY experiments showed an enhancement between the protons H-2 and H-4, and between H-4’ and the methylenic protons of the substituent at C-4. These data are consistent with a cis stereochemistry in the tetrahydroquinoline system. Thus, the configuration of C-4 was assigned as S.
In summary, the 6-exo intramolecular cyclization of aryllithiums obtained by iodine-lithium exchange reaction on N-alkenyl 2-iodoanilines allows the synthesis of 4-substituted tetrahydroquinolines. However, the alkene has to be substituted with a stabilizing group for the resulting organolithium to favor the cyclization. This high reactivity results in a low enantioselection when (–)-sparteine (4) or (+)-sparteine surrogate (5) are used as chiral ligands. The reaction conditions are not compatible with the presence of an ester moiety. For this case, the Mizoroki-Heck reaction constitutes an efficient alternative.14 The 2-benzyloxymethyl derivatives could be obtained, but with low stereoselectivity. Finally, the carbolithiation of an enantiomerically pure N-alkenyl 2-iodoaniline proceeded with complete diastereoselectivity, allowing the synthesis of an enantiomerically pure 2,4-substituted tetrahydroquinoline.
EXPERIMENTAL
General experimental methods: IR spectra were obtained in film over NaCl pellets. NMR spectra were recorded at 20-25 ˚C, at 300 MHz for 1H and 75.5 MHz for 13C or at 500 MHz for 1H and 125.7 MHz for 13C in CDCl3 solutions, unless otherwise stated. Assignments of individual 13C and 1H resonances are supported by DEPT experiments and 2D correlation experiments (COSY, HSQCed or HMBC). Mass spectra were recorded under electron impact (EI) at 70 eV, or under chemical ionization (CI) at 230 eV. Exact mass was obtained using a TOF detector. TLC was carried out with 0.2 mm thick silica gel plates. Visualization was accomplished by UV light. Flash column chromatography was performed on silica gel (230-400 mesh) or on alumina (70-230 mesh). Chiral stationary phase HPLC was performed using Chiralcel ASH or OD columns (0.46 cm × 25 cm). All solvents used in reactions were anhydrous and purified according to standard procedures. 21 n- and t-Butyllithium were titrated with diphenylacetic acid or N-benzyl benzamide periodically prior to use. All air- or moisture-sensitive reactions were performed under argon; the glassware was dried (130 ˚C) and purged with argon.
Starting Materials. Haloanilines 1a-i were prepared by the appropriate reported procedure.14 All other chemicals used in this study were commercially available.
N-(But-3-en-1-yl)-N-methylaniline (3a). n-BuLi (1.12 mL of a 1.19 M solution in hexanes, 1.34 mmol) and TMEDA (0.20 mL, 1.34 mmol) were added sequentially to a solution of 1a (170 mg, 0.61 mmol) in dry THF (10 mL) at –78 ºC. The resulting solution was stirred at –78 ºC for 16 h. The reaction was quenched by the addition of sat. aq. NH4Cl solution (10 mL) at –78 ºC. The organic layer was separated and the aqueous phase was extracted with Et2O (10 mL) and CH2Cl2 (2 × 10 mL). The combined organic extracts were dried with Na2SO4 and evaporated under reduced pressure to give deiodinated aniline 3a as an oil (90 mg, 90%): IR (film) 1631 cm-1; 1H NMR δ 2.30 – 2.41 (m, 2H), 2.95 (s, 3H), 2.34 (t, J = 7.1 Hz, 2H), 5.00 – 5.18 (m, 2H), 5.76 – 5.94 (m, 1H), 6.66 – 6.76 (m, 3H), 7.19 – 7.30 (m, 2H); 13C NMR δ 31.0, 38.3, 52.4, 112.1, 116.0, 116.4, 129.1, 135.9, 149.0.
N,N-Diethyl-2-(1-methyl-1,2,3,4-tetrahydroisoquinolin-4-yl)acetamide (2b) (Table 1, entry 10). To a solution of mesityl bromide (0.05 mL, 0.33 mmol) in dry THF (20 mL), t-BuLi (0.04 mL of a 0.99 M solution in pentane, 0.67 mmol) was added at –78 °C and the reaction mixture was stirred at –20 ºC for 1 h. The reaction mixture was cooled again to –78 °C, and a solution of the o-iodoaniline 1b (60 mg, 0.17 mmol) in dry THF (10 mL) was added and the reaction mixture was stirred for 10 min. The reaction was quenched by the addition of sat. aq. NH4Cl (10 mL) at –78 °C. The organic layer was separated and the aqueous phase was extracted with Et2O (10 mL) and CH2Cl2 (2 × 10 mL). The combined organic extracts were dried with Na2SO4 and concentrated in vacuo. Flash column chromatography (silica gel, 80% hexane/AcOEt) afforded tetrahydroquinoline 2b as an oil (40 mg, 85%): IR (film) 1631 cm-1; 1H NMR δ 1.09 (t, J = 7.1 Hz, 3H), 1.13 (t, J = 7.1 Hz, 3H), 1.86 – 1.90 (m, 1H), 2.07 – 2.12 (m, 1H), 2.50 (dd, J = 15.1, 9.3 Hz, 1H), 2.60 (dd, J = 15.1, 5.3 Hz, 1H), 2.90 (s, 3H), 3.16 – 3.22 (m, 3H), 3.23 – 3.27 (m, 1H), 3.28 – 3.36 (m, 1H), 3.43 – 3.49 (m, 2H), 6.56 – 6.64 (m, 2H), 7.00 – 7.13 (m, 2H); 13C NMR δ 13.1, 14.3, 26.8, 33.1, 38.9, 39.9, 40.2, 41.9, 47.5, 110.9, 116.1, 125.6, 128.4, 129.1, 146.1, 170.8; MS (EI) m/z (rel intensity) 260 (M+, 95), 160 (81), 146 (100), 144 (99), 131 (59), 91 (8), 77 (8); HRMS (EI) Calcd for C16H24N2O [M+]: 260.1889. Found: 260.1881.
N-Methoxy-N-methyl-2-(1-methyl-1,2,3,4-tetrahydroisoquinolin-4-yl)acetamide (2c) (Table 1, entry 15). According to the above procedure, 1c (110 mg, 0.28 mmol) was treated with MesLi [0.57 mmol, prepared from mesityl bromide (0.09 mL, 0.57 mmol) and t-BuLi (1.53 mL of a 0.74 M solution in pentane, 1.13 mmol)] at –78 ºC and the reaction mixture was stirred for 10 min. After work up, flash column chromatography (silica gel, 80% hexane/AcOEt) afforded 2c as an oil (50 mg, 71%): IR (film) 1659 cm-1; 1H NMR δ 1.84 – 1.89 (m, 1H), 2.04 – 2.12 (m, 1H), 2.66 – 2.78 (m, 2H), 2.91 (s, 3H), 3.16 – 3.19 (m, 1H), 3.20 (s, 3H), 3.30 (td, J = 11.2, 3.7 Hz, 1H), 3.39 – 3.44 (m, 1H), 3.59 (s, 3H), 6.60 – 6.64 (m, 2H), 7.04 – 7.13 (m, 2H); 13C NMR δ 26.7, 32.0, 32.5, 38.9, 39.0, 47.3, 61.1, 110.9 , 116.1, 125.4, 127.5, 128.4, 146.1, 173.2; MS (EI) m/z (rel intensity) 248 (M+, 17), 158 (9), 144 (100), 118 (6), 91 (4), 77(4); HRMS (EI) Calcd for C14H20N2O2 [M+]: 248.1525. Found: 248.1517.
n-BuLi/(–)-sparteine mediated cyclization of 1b. Synthesis of enantioenriched 2b (Table 2, entry 1). n-BuLi (0.85 mL of a 0.77 M solution in hexane, 0.65 mmol) was added to a solution of (–)-sparteine (0.15 mL, 0.63 mmol) in dry toluene (10 mL) at –90 °C, and the reaction mixture was stirred at this temperature for 30 min. Then a solution of o-iodoaniline 1b (110 mg, 0.30 mmol) in dry toluene (5 mL) was added dropwise at this temperature, and the reaction mixture was stirred for 30 min. The reaction was quenched by the addition of sat. aq. NH4Cl (10 mL) at –90 °C, and allowed to reach rt The organic layer was separated and the aqueous phase was extracted with Et2O (10 mL) and CH2Cl2 (2 × 10 mL). The combined organic extracts were washed with brine (2 × 10 mL), dried (Na2SO4) and concentrated in vacuo. Flash column chromatography (silica gel, 80% hexane/AcOEt) afforded 2b as an oil (38 mg, 49%). The spectroscopic data were identical to those of the racemic mixture. The enantiomeric excess was determined by HPLC to be 10%. [Chiralcel OJH, 2% hexane: 2-propanol, 0.8 mL/min, tr = 21.5 min (55%), tr= 24.8 min (45%)].
n-BuLi/(–)-sparteine mediated cyclization of 1c. Synthesis of enantioenriched 2c (Table 2, entry 10). According to the previous procedure, 1c (110 mg, 0.28 mmol) was treated with n-BuLi (0.86 mL of a 0.71 M solution in hexane, 0.61 mmol) and (–)-sparteine (0.16 mL, 0.44 mmol) at –95 ºC and the reaction mixture was stirred for 10 min. After work up, flash column chromatography (silica gel, 80% hexane/AcOEt) afforded 2c as an oil (23 mg, 33%). The spectroscopic data were identical to those of the racemic mixture. The enantiomeric excess was determined by HPLC to be 28%. [Chiralcel OJH, 2% hexane: 2-propanol, 0.8 mL/min, tr = 23.6 min (64%), tr= 25.3 min (36%)].
2-Allyl-2-butyl-1-methyl-1H-indole (6). n-BuLi (0.70 mL of a 0.98 M solution in hexanes, 0.68 mmol) was added to a solution of 1d (96 mg, 0.31 mmol) in dry THF (8 mL) at –78 ºC. The resulting solution was stirred at –78 ºC for 30 min. The reaction was quenched by the addition of sat. aq. NH4Cl solution (5 mL) at –78 ºC, and allowed to warm up to rt. Et2O (10 mL) was added and the organic layer was separated. The aqueous phase was extracted with CH2Cl2 (2 × 10 mL). The combined organic extracts were washed with brine (15 mL), dried (Na2SO4) and concentrated in vacuo. Flash column chromatography (silica gel, 90% hexane/AcOEt) afforded indole 6 as an oil (14 mg, 20%): IR (film) 992, 913 cm-1; 1H NMR δ 0.93 (t, J = 7.2 Hz, 3H), 1.32 – 1.45 (m, 2H), 1.55 – 1.65 (m, 2H), 2.71 (t, J = 7.4 Hz, 2H), 3.53 (d, J = 5.6 Hz, 2H), 3.64 (s, 3H), 4.95 (dd, J = 17.7, 1.1 Hz, 1H), 5.08 (dd, J = 10.1, 1.1 Hz, 1H), 5.87 – 5.99 (m, 1H), 7.08 (t, J = 7.6 Hz, 1H), 7.17 (t, J = 7.6 Hz, 1H), 7.25 (d, J = 7.8 Hz, 1H), 7.55 (d, J = 7.8 Hz, 1H); 13C NMR δ 14.0, 22.8, 24.1, 28.8, 29.6, 33.6, 108.6, 112.7, 116.0, 118.4, 118.5, 120.7, 127.8, 133.5, 135.3, 136.8; MS (EI) m/z (rel intensity) 227 (M+, 6), 184 (50), 85 (98), 83 (100); HRMS (EI) Calcd for C16H21N [M+]: 227.1674. Found: 227.1674. Anal. Calcd for C16H21N: C, 84.53; H, 9.31; N, 6.16. Found: C, 84.09; H, 9.45; N, 5.70.
N-[(1-Benzyloxy)pent-4-en-2-yl]-N-methylaniline (3b) (Table 3, entry 2). t-BuLi (0.81 mL of a 1.10 M solution in pentane, 0.89 mmol) was added to a solution of 1f (160 mg, 0.41 mmol) in dry THF (10 mL) at –78 ºC. The resulting solution was stirred at –78 ºC for 5 min. The reaction was quenched by the addition of sat. aq. NH4Cl solution (5 mL) at –78 ºC. Et2O (10 mL) was added and the organic layer was separated. The aqueous phase was extracted with CH2Cl2 (2 × 10 mL). The combined organic extracts were washed with brine (15 mL), dried (Na2SO4) and concentrated in vacuo. Flash column chromatography (silica gel, 90% hexane/AcOEt) afforded deiodinated aniline 3b as an oil (100 mg, 91%): IR (film) 1638, 1111.1, 747, 694 cm-1; 1H NMR δ 2.41 – 2.46 (m, 2H), 2.82 (s, 3H), 3.52 – 3.67 (m, 2H), 4.09 – 4.19 (m, 1H), 4.52 (s, 2H), 5.00 – 5.15 (m, 2H), 5.74 – 5.86 (m, 1H), 6.73 (t, J = 7.1 Hz, 1H), 6.83 (d, J = 7.9 Hz, 2H), 7.23 – 7.35 (m, 7H);13C NMR δ 31.1, 34.1, 57.9, 70.7, 73.0, 113.0, 116.4, 116.6, 127.4, 127.5, 128.3, 129.0, 135.6, 138.3, 150.6; MS (EI) m/z (rel intensity) 281 (M+, 9), 240 (52), 160 (90), 91 (40), 85 (100), 77 (11); HRMS (EI) Calcd for C19H23NO [M+]: 281.1780. Found: 281.1773. Anal. Calcd for C19H23NO: C, 81.10; H, 8.24; N, 4.98. Found: C, 81.28; H, 8.38; N, 4.98.
(2SR, 4RS)- and (2RS, 4RS)-2-[2-(Benzyloxymethyl)-1-methyl-1,2,3,4-tetrahydroquinolin-4-yl]-N,N-diethylacetamide (2g) (Table 3, entry 4). n-BuLi (0.44 mL of a 1.10 M solution in hexanes, 0.49 mmol) was added to a solution of 1g (110 mg, 0.22 mmol) in dry THF (10 mL) at –105 ºC. The resulting solution was stirred at –105 ºC for 5 min. The reaction was quenched by the addition of sat. aq. NH4Cl solution (5 mL) at –105 ºC. Et2O (10 mL) was added and the organic layer was separated. The aqueous phase was extracted with CH2Cl2 (2 × 10 mL). The combined organic extracts were washed with brine (15 mL), dried (Na2SO4) and concentrated in vacuo. Flash column chromatography (silica gel, 50% hexane/AcOEt) afforded tetrahydroquinoline 2g as a 39:61 mixture of (2RS,4RS)-trans and (2SR,4RS)-cis diastereomers (48 mg, 57%): IR (film) 1637, 1597 cm-1; 1H NMR δ 1.09 – 1.15 (m, 12 H, both diast.), 1.75 – 1.81 (m, 2H, both diast.), 2.11- 2.16 (m, 1H, minor diast.), 2.23 – 2.29 (m, 1H, major diast.), 2.43 – 2.47 (m, 2H, both diast.), 2.81 – 2.86 (m, 2H, both diast.), 2.97 (s, 3H, major diast.), 2.99 (s, 3H, minor diast.), 3.21 – 3.35 (m, 4H, major diast.), 3.33 – 3.47 (m, 1H major diast., 5H, minor diast.), 3.48 – 3.57 (m, 1H major diast., 3H, minor diast.), 3.61 – 3.66 (m, 2H, major diast.), 4.51 (s, 4H, both diast.), 6.58 (d, J = 8.2 Hz, 1H, minor diast.), 6.61 – 6.66 (m, 2H major diast., 1H, minor diast.), 7.01 (d, J = 7.4 Hz, 1H, major diast.), 7.03 (d, J = 7.4 Hz, 1H, minor diast.), 7.11 (t, J = 7.6 Hz, 2H, both diast.), 7.26 – 7.37 (m, 10H, both diast.); 13C NMR δ 13.1 (major diast.), 14.4 (minor diast.), 30.4 (minor diast.), 30.9 (minor diast.), 31.9 (major diast.), 32.1 (major diast.), 37.5 (major diast.), 38.2 (minor diast.), 38.3 (minor diast.), 38.4 (major diast.), 40.3 (major diast.), 41.9 (minor diast.), 57.3 (minor diast.), 58.0 (major diast.), 72.3 (minor diast.), 72.8 (major diast.), 73.1 (major diast.), 73.3 (minor diast.), 111.1 (minor diast), 112.0 (major diast.), 115.8 (minor diast.), 116.3 (major diast.), 125.5 (minor diast.), 125.8 (major diast.), 126.4 (minor diast.), 127.1 (major diast.), 127.2 (both diast.), 127.3 (major diast.), 127.4 (minor diast.), 127.5 (minor diast.), 127.6 (major diast.), 128.3 (both diast.), 138.2 (major diast.), 138.3 (minor diast.), 145.4 (minor diast.), 146.3 (major diast.), 170.93 (minor diast.), 170.99 (major diast.); MS (EI) m/z (rel intensity) 272 (M+ – OCH2Ph, 6), 158 (20), 144 (100), 115 (10), 97 (13), 91 (15), 83 (11), 77 (11); HRMS (EI) Calcd for C24H32N2O2 [M+]: 380.2464. Found: 380.2471. Anal. Calcd for C24H32N2O2: C, 75.75; H, 8.48; N, 7.36. Found: C, 75.46; H, 8.62; N, 7.51.
(2SR, 4RS)- and (2RS, 4RS)-2-[2-(Benzyloxymethyl)-1-methyl-1,2,3,4-tetrahydroquinolin-4-yl]-N-methoxy-N-methylacetamide (2h) (Table 3, entry 11). t-BuLi (0.57 mL of a 0.89 M solution in pentane, 0.51 mmol) and TMEDA (0.08 mL, 0.51 mmol) were added sequentially to a solution of 1h (114 mg, 0.23 mmol) in dry THF (10 mL) at –105 ºC. The resulting solution was stirred at –105 ºC for 5 min. The reaction was quenched by the addition of sat. aq. NH4Cl solution (10 mL) at –105 ºC. Et2O (10 mL) was added and the organic layer was separated. The aqueous phase was extracted with CH2Cl2 (2 × 10 mL). The combined organic extracts were washed with brine (15 mL), dried (Na2SO4) and concentrated in vacuo. Flash column chromatography (silica gel, 70% hexane/AcOEt) afforded tetrahydroquinoline 2h as a 33:67 mixture of (2RS,4RS)-trans and (2SR,4RS)-cis diastereomers (45 mg, 53%): IR (film) 1656, 1599 cm-1; 1H NMR δ 1.75 – 1.85 (m, 2H, both diast.), 2.10 – 2.18 (m, 1H, major diast.), 2.21 – 2.29 (m, 1H, minor diast.), 2.59 – 2.67 (m, 2H, both diast.), 2.93 – 2.98 (m, 2H, both diast.), 2.98 (s, 3H, minor diast.), 2.99 (s, 3H, major diast.), 3.20 (s, 3H, minor diast.), 3.21 (s, 3H, major diast.), 3.32 – 3.41 (m, 2H, both diast.), 3.50 – 3.56 (m, 4H, both diast.), 3.58 – 3.66 (m, 2H, both diast.), 3.61 (s, 3H, minor diast.) 3.62 (s, 3H, major diast.) 4.50 (s, 2H, minor diast.), 4.52 (s, 2H, major diast.), 6.57 – 6.69 (m, 4H, both diast.), 7.02 – 7.14 (m, 4H, both diast.), 7.26 – 7.34 (m, 10H, both diast.); 13C NMR δ 29.8 (both diast.), 30.7 (major diast.), 30.8 (minor diast.), 31.1 (both diast.), 37.4 (major diast.), 37.5 (minor diast.), 38.1 (both diast.), 57.1 (major diast.), 58.0 (minor diast.), 61.1 (major diast.), 61.2 (minor diast.), 72.1 (major diast.), 72.7 (minor diast.), 73.1 (minor diast.), 73.5 (major diast.), 111.2 (major diast.), 112.0 (minor diast.), 115.8 (major diast.), 116.3 (minor diast.), 125.4 (minor diast.), 125.5 (major diast.)125.8 (minor diast.), 126.4 (major diast.), 126.9 (minor diast.), 127.3 (major diast.), 127.4 (minor diast.) 127.5 (both diast.), 127.6 (major diast.), 128.3 (both diast.), 138.2 (major diast.), 138.3 (minor diast.), 145.4 (major diast.), 146.2 (minor diast.), 173.2 (both diast.); MS (EI) m/z (rel intensity) 368 (M+, 12), 247 (86), 229 (13), 217 (60), 158 (61), 145 (100), 130 (34), 128 (11), 97 (12), 91 (78), 77 (10), 71 (17), 57 (23); HRMS (EI) Calcd for C22H28N2O3 [M+]: 368.2100. Found: 368.2094.
(–)-2-[(2R,4S)-2-[(S)-2,2-Dimethyl-1,3-dioxolan-4-yl]-1-methyl-1,2,3,4-tetrahydroquinolin-4-yl]-N,N-diethylacetamide (2i). n-BuLi (0.70 mL of a 1.0 M solution in hexanes, 0.69 mmol) was added to a solution of 1i (150 mg, 0.31 mmol) in dry THF (10 mL) at –78 ºC. The resulting solution was stirred at –78 ºC for 10 min. The reaction was quenched by the addition of sat. aq. NH4Cl solution (10 mL) at –78 ºC. The organic layer was separated and the aqueous phase was extracted with Et2O (10 mL) and CH2Cl2 (2 × 10 mL). The combined organic extracts were washed with brine (2 × 10 mL), dried (Na2SO4) and concentrated in vacuo. Flash column chromatography (silica gel, 80% hexane/AcOEt) afforded 2i as an oil (70 mg, 60%): [α ]D20 –46.3 (c 0.5, CH2Cl2); IR (film) 1659 cm-1; 1H NMR δ 1.12 – 1.18 (m, 6H), 1.30 (s, 3H), 1.40 (s, 3H), 1.83 – 1.88 (m, 1H), 2.18 – 2.23 (m, 1H), 2.64 (dd, J = 15.7, 8.6 Hz, 1H), 2.78 (dd, J = 15.7, 5.2 Hz, 1H), 2.99 (s, 3H),3.23 – 3.36 (m, 2H), 3.37 – 3.41 (m, 1H), 3.41 – 3.46 (m, 2H), 3.48 – 3.52 (m, 1H), 3.76 (t, J = 7.3 Hz, 1H), 4.03 – 4.11 (m, 2H), 6.62 (d, J = 7.9 Hz, 1H), 6.67 (t, J = 7.4 Hz, 1H), 7.05 (d, J = 7.4 Hz, 1H), 7.10 (t, J = 7.9 Hz, 1H); 13C NMR δ 13.2, 14.3, 25.2, 26.5, 30.3, 30.8, 39.5, 39.6, 40.4, 41.9, 60.5, 67.9, 76.9, 108.6, 113.1, 116.9, 127.2, 127.6, 128.1, 145.9, 171.2. Anal. Calcd for C21H32N2O3: C, 69.97; H, 8.95; N, 7.77. Found: C, 69.86; H, 8.88; N, 7.57.
ACKNOWLEDGEMENTS
We wish to thank the Ministerio de Ciencia e Innovación (CTQ2009-07733), the Gobierno Vasco (IT-623-13) and the Universidad del País Vasco / Euskal Herriko Unibertsitatea UPV/EHU (UFI11/22, GIU 09/46) for their financial support. Technical and human support provided by Servicios Generales de Investigación SGIker (UPV/EHU, MICINN, GV/EJ, ERDF and ESF) is gratefully acknowledged.
References
1. For selected reviews, see: I. Marek, J. Chem. Soc., Perkin Trans. 1, 1999, 535; CrossRef J. Clayden, 'Organolithiums: Selectivity for Synthesis', Pergamon Press, New York, 2002, pp. 282–335; M. J. Mealy and W. F. Bailey, J. Organomet. Chem., 2002, 646, 59; CrossRef J. F. Normant, Top. Organomet. Chem., 2003, 287; F. J. Fañanás and R. Sanz, in 'The Chemistry of Organolithium Compounds, Patai Series: The Chemistry of Functional Groups', Vol. 2, ed. by Z. Rappoport and I. Marek, Wiley, Chichester, 2006, pp. 295–379; CrossRef A. M. L. Hogan and D. F. O’Shea, Chem. Commun., 2008, 3839; CrossRef U. Martínez-Estíbalez, A. Gómez-SanJuan, O. García-Calvo, S. Arrasate, N. Sotomayor, and E. Lete, in 'Targets in Heterocyclic Systems', Vol. 14, ed. by O. Attanasi and D. Spinelli, Italian Society of Chemistry, Rome, 2010, pp. 124–149; N. Sotomayor and E. Lete, in 'Science of Synthesis. Knowledge Updates 2011/4', ed. by D. G. Hall, K. Ishikara, J. J. Li, I. Marek, M. North, E. Schaumann, S. M. Weinreb, and M. Yus, Thieme, Stuttgart, 2011, pp. 191–251.
2. For selected examples, see: A. Krief, B. Remacle, and J. Mercier, Synlett, 2000, 1443; CrossRef K. Deng, A. Bensari, and T. Cohen, J. Am. Chem. Soc., 2002, 124, 12106; CrossRef W. F. Bailey, T. Daskapan, and S. Rampalli, J. Org. Chem., 2003, 68, 1334; CrossRef R. Ortiz and M. Yus, Tetrahedron, 2005, 61, 1699; CrossRef K. Deng, A. Bensari-Bouguerra, J. Whetstone, and T. Cohen, J. Org. Chem., 2006, 71, 2360; CrossRef T. Liu, X. Zhao, L. Lu, and T. Cohen, Org. Lett., 2009, 11, 4576. CrossRef
3. For selected examples, see: F. J. Fañanás, A. Granados, R. Sanz, J. M. Ignacio, and J. Barluenga, Chem. Eur. J., 2001, 7, 2896; CrossRef J. Barluenga, F. J. Fañanás, R. Sanz, and J. M. Ignacio, Eur. J. Org. Chem., 2003, 771; CrossRef I. Coldham, K. N. Price, and R. E. Rathmell, Org. Biomol. Chem., 2003, 1, 2111; CrossRef G. Christoph, C. Stratmann, I. Coldham, and D. Hoppe, Org. Lett., 2006, 8, 4469; CrossRef W. F. Bailey, P. D. Salgaonkar, J. D. Brubaker, and V. Sharma, Org. Lett., 2008, 10, 1071. CrossRef
4. For reviews on the use of (–)-sparteine as chiral ligand, see: P. Beak, D. R. Anderson, M. D. Curtis, J. M. Laumer, D. J. Pippel, and G. A. Weisenburger, Acc. Chem. Res., 2000, 33, 715; CrossRef D. Hoppe and G. Christoph, in 'The Chemistry of Organolithium Compounds, Patai Series: The Chemistry of Functional Groups', ed. by Z. Rappoport and I. Marek, Wiley, Chichester, 2004, pp. 1055–1164. CrossRef
5. For a review, see: A. Gómez-SanJuan, N. Sotomayor, and E. Lete, Beilstein J. Org. Chem., 2013, 9, 313, and references therein. CrossRef
6. R. Pedrosa, C. Andrés, J. M. Iglesias, and A. Pérez-Encabo, J. Am. Chem. Soc., 2001, 123, 1817; CrossRef S. D. Rychnovsky and L. R. Takaoka, Angew. Chem. Int. Ed., 2003, 42, 818; CrossRef T. E. La Cruz and S. D. Rychnovsky, J. Org. Chem., 2006, 71, 1068; CrossRef R. J. Bahde and S. D. Rychnovsky, Org. Lett., 2008, 10, 4017. CrossRef
7. S. Lage, I. Villaluenga, N. Sotomayor, and E. Lete, Synlett, 2008, 3188; CrossRef O. García-Calvo, N. Sotomayor, E. Lete, and I. Coldham, ARKIVOC, 2011, v, 57; O. García-Calvo, E. Coya, S. Lage, I. Coldham, N. Sotomayor, and E. Lete, Eur. J. Org. Chem., 2013, 1460. CrossRef
8. For selected recent reviews, see: K. Kaur, M. Jain, R. P. Reddy, and R. Jain, Eur. J. Med. Chem., 2010, 45, 3245; CrossRef V. R. Solomon and H. Lee, Curr. Med. Chem., 2011, 18, 1488; CrossRef A. Garrido Montalbán, in 'Heterocycles in Natural Products Synthesis', ed. by K. C. Majumdar and S. K. Chattopadhyay, Wiley-VCH, Weinheim, 2011, pp. 299-339; CrossRef V. Sridharan, P. A. Suryavanshi, and J. C. Menéndez, Chem. Rev., 2011, 111, 7157; CrossRef see also, J. P. Michael, Nat. Prod. Rep., 2008, 25, 166, and previous reports in this series. CrossRef
9. A. Mitchinson and A. Nadin, J. Chem. Soc., Perkin Trans. 1, 2000, 2862. See also ref. 8.
10. R. W. Carling, P. D. Leeson, A. M. Moseley, J. D. Smith, K. Saywell, M. D. Tricklebank, J. A. Kemp, G. R. Marshall, A. C. Foster, and S. Grimwood, Bioorg. Med. Chem. Lett., 1993, 3, 65; CrossRef M. Rowley, J. J. Kulagowski, A. P. Watt, D. Rathbone, G. L. Stevenson, R. W. Carling, R. Baker, G. R. Marshall, J. A. Kemp, A. C. Foster, S. Grimwood, R. Hargreaves, C. Hurley, K. L. Saywell, M. D. Tricklebank, and P. D. Leeson, J. Med. Chem., 1997, 40, 4053; CrossRef G. Dannhardt, M. V. Gruchalla, B. K. Kohl, and C. G. Parsons, Arch. Pharm. Pharm. Med. Chem., 2000, 333, 267; CrossRef R. Di Fabio, E. Tranquillini, B. Bertani, G. Alvaro, F. Micheli, F. Sabbatini, M. D. Pizzi, G. Pentassuglia, A. Pasquarello, T. Messeri, D. Donati, E. Rattti, R. Arban, G. Dal Forno, A. Reggiani, and R. J. Barnaby, Bioorg. Med. Chem. Lett., 2003, 13, 3863; CrossRef R. Di Fabio, G. Alvaro, B. Bertani, D. Donati, D. M. Pizzi, G. Gentile, G. Pentassuglia, S. Giacobbe, S. Spada, E. Ratti, M. Corsi, M. Quartaroli, R. J. Barnaby, and G. Vitulli, Bioorg. Med. Chem. Lett., 2007, 17, 1176. CrossRef
11. H. Kim, H. Gim, M. Yang, J.-H. Ryu, and R. Jeon, Heterocycles, 2007, 71, 2131. CrossRef
12. T. Guo, H. Gu, D. W. Hobbs, L. L. Rokosz, T. M. Stauffer, B. Jacob, and J. W. Clader, Bioorg. Med. Chem. Lett., 2007, 17, 3010; CrossRef T. Asberom, T. A. Bara, J. W. Clader, W. J. Greenlee, H. S. Guzik, H. B. Josien, W. Li, E. M. Parker, D. A. Pissarnitski, L. Song, L. Zhang, and Z. Zhao, Bioorg. Med. Chem. Lett., 2007, 17, 205. CrossRef
13. U. Martínez-Estíbalez, N. Sotomayor, and E. Lete, Org. Lett., 2009, 11, 1237. CrossRef
14. For the synthesis of these anilines see, U. Martínez-Estíbalez, O. García-Calvo, V. Ortiz-de-Elguea, N. Sotomayor, and E. Lete, Eur. J. Org. Chem., 2013, 3013. CrossRef
15. W. F. Bailey and X. L. Jiang, J. Org. Chem., 1996, 61, 2596. CrossRef
16. G. Sanz and U. M. Groth, J. Am. Chem. Soc., 2000, 122, 6789. CrossRef
17. M. J. Mealy, M. R. Luderer, W. F. Bailey, and M. B. Sommer, J. Org. Chem., 2004, 69, 6042. CrossRef
18. W. F. Bailey and M. J. Mealy, J. Am. Chem. Soc., 2000, 122, 6787. CrossRef
19. P. O’Brien, Chem. Commun., 2008, 655; CrossRef M. J. Dearden, M. J. McGrath, and P. O’Brien, J. Org. Chem., 2004, 69, 5789. CrossRef
20. The (2R,4R)-trans diastereomer was not detected by NMR spectroscopy.
21. D. D. Perrin and W. L. F. Armarego, 'Purification of Laboratory Chemicals', 4th ed., Pergamon Press, Oxford, 1997; D. B. G. Williams and M. Lawton, J. Org. Chem., 2010, 75, 8351. CrossRef