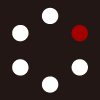
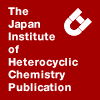
HETEROCYCLES
An International Journal for Reviews and Communications in Heterocyclic ChemistryWeb Edition ISSN: 1881-0942
Published online by The Japan Institute of Heterocyclic Chemistry
e-Journal
Full Text HTML
Received, 3rd July, 2013, Accepted, 28th August, 2013, Published online, 6th September, 2013.
DOI: 10.3987/COM-13-S(S)81
■ A Catalytic C-C Bond-Formation with Minimal Use of Protecting Groups: Construction of Functionalized Isotetronic Acid Derivatives
Yohei Shimizu,* Kouji Yasuda, and Motomu Kanai*
Graduate School of Pharmaceutical Sciences, The University of Tokyo, 7-3-1 Hongo, Bunkyo-ku, Tokyo 113-0033, Japan
Abstract
A catalytic method to construct multi-functionalized isotetronic acids was developed using cyclic hemiacetals and pyruvic acid derivatives as substrates. The key to success was the combination of catalytic amounts of iron(III) trifluoroacetate and 2-mercaptopyridine. A catalytic asymmetric variant was also developed using 6,6’-dimethoxy-binaphthyl phosphate instead of 2-mercaptopyridine.Isotetronic acids are an important class of natural compounds (Figure 1.) that exhibit various biological activities.1 For example, butyrolactone I is a cyclin-dependent kinase inhibitor,1a and WF-3681 exhibits aldose reductase-inhibiting activity.1b This structural motif is also widely used as a versatile intermediate in the synthesis of complex natural products,2 and there are several approaches to constructing the isotetronic acid skeleton.3-5 The cross aldol reaction of aldehydes and pyruvic acid derivatives6 is straightforward, and a highly efficient method of accessing various isotetronic acids. Organocatalysts are especially effective for the cross aldol reaction, affording high enantioselectivity even when only water is used as the solvent.5b-d To our knowledge, however, all reported examples used simple aldehydes as the substrate, giving products with non-functionalized alkyl or aromatic side chains. Here we report the first catalytic addition of pyruvic acid derivatives to cyclic hemiacetals,7 affording isotetronic acids containing functionalized side chains. This method will be effective for synthesizing biologically active isotetronic acids, because natural products and biologically active compounds usually have polar functional groups on their side chains.
We chose the reaction between phenylpyruvic acid (5a) and 6-membered lactol 6a for optimization of the conditions. Considering the effectiveness of organocatalysts for isotetronic acid synthesis, we first examined L-proline and diphenylboric acid as catalysts (Table 1, entries 1 and 2). Diphenylboric acid gave only a trace amount of product 7aa, however, and L-proline afforded no product at all. We then turned our attention to metal-based Brønsted base catalysts. Although lithium acetate and zinc acetate did not give promising results (entries 3 and 5), copper acetate and iron acetate afforded the desired product in 5% yield (entries 4 and 6). We then studied the counterion effects of the iron catalyst and found that an iron trifluoroacetate complex8 gave superior reactivity (entry 7). To realize higher reactivity, several
catalytic bases were added to increase the concentration of the aldehyde form from the hemiacetal form.9 The addition of 2,6-di-tert-butylpyridine increased the reactivity (entry 8), and the addition of 2-hydroxypyridine further improved the reactivity (entry 9). Finally, we found that 2-mercaptopyridine was the best additive to afford 7aa in 99% yield (entry 10).10 Although reactivity decreased dramatically, the reaction could be conducted in water, which highlighted the tolerance of the catalytic system to free OH groups (entry 11). We assumed that the significant improvement in reactivity following the addition of 2-mercaptopyridine was due to its ambiphilic acid/base nature. The pyridine moiety of 2-mercaptopyridine can function as a Brønsted base to deprotonate the anomeric hydroxy group of 6a, and the thiol moiety can function as a proton donor for the endocyclic oxygen atom (A). This synchronous activation of the lactol would facilitate the formation of the aldehyde form from the lactol form.11
Under these optimized conditions, we next investigated the substrate scope (Table 2).12 Non-substituted 5-, 6-, and 7-membered simple lactols13 were good substrates for this reaction, and the corresponding products were obtained in moderate to high yield (entries 1-3). Our examination of substituted lactols (entries 4-7) revealed that the position of the hydroxy groups markedly affected the reactivity. When an additional hydroxy group was introduced at the C4 position (6e), the reaction proceeded smoothly to afford product 7ae in 64% yield (entry 5). A hydroxy group at the C2 position (6f), however, significantly hampered the reaction, and product 7af was obtained in only 21% yield (entry 6). Notably, erythrose 6g could be utilized as a substrate for this reaction, producing multi-hydroxylated product 7ag in 24% yield with 11:1 diastereoselectivity. This result indicated that the current reaction conditions could be applied to a facile synthesis of multi-functional isotetronic acids, although the yield requires further improvement. We also investigated oligomerized aldehydes (entries 8-10), and the products were obtained in moderate yield. The scope of the pyruvic acid derivatives was also examined. A free phenol moiety did not affect the reaction (entry 11), which again supported the functional group tolerance of this reaction. The sterically demanding substrate 5c containing an ortho-nitrophenyl group also reacted without problem. An α-methylpyruvic acid derivative, however, did not react at all under the optimized conditions. Further investigation of iron sources indicated that the use of Fe(OH)314 instead of the iron trifluoroacetate complex afforded the product in low yield (entry 13). The use of pyruvic acid itself did not afford the desired product even in the presence of Fe(OH)3.
We next turned our attention to the development of an asymmetric variant. Based on the assumed mechanism of 2-mercaptopyridine to facilitate the conversion of the lactol form to the aldehyde form (Table 1, A), the resulting aldehyde would coordinate to 2-mercaptopyridine during the subsequent C-C bond-formation step. Hence, chiral 2-mercaptopyridine analogs would lead to asymmetric induction. We selected binaphthyl phosphates as candidates for such analogs.15 A simple binaphthyl phosphate 8a was examined first (Table 3). Although the yield was low, enantioselectivity was high (78% ee).16 In contrast, the use of (2R,3R)-tartaric acid, (R)-BINOL, and (R)-camphorsulfonic acid as asymmetric catalysts produced no significant enantioselectivity (entries 2-4). Both reactivity and enantioselectivity were dramatically affected by the electronic characteristics of the chiral phosphates. Introduction of electron-withdrawing bromide atoms at the 6,6’-positions of the binaphthyl core (8b) dramatically decreased the yield and enantioselectivity to 10% yield and 25% ee, respectively (entry 5). In contrast, introduction of electron-donating methoxy groups (8c) improved the yield and enantioselectivity (entry 6).
The substrate scope of the asymmetric reaction was examined under the optimized conditions (Table 4).12 Consistent with the results of the racemic variant, 5-membered lactol 6b exhibited high reactivity and enantioselectivity (70% yield and 91% ee). In the case of 7-membered lactol 6c,13 however, the enantiomeric excess was significantly decreased to 22% ee. The sharp difference in enantioselectivity depending on the substrate ring size is likely due to the relative concentration between the lactol form and the aldehyde form. The 7-membered lactol 6c preferred the aldehyde form rather than the lactol form. This preference was opposite that of the 5- and 6-membered lactols 6a and 6b. Thus, the background reaction without interaction of the chiral phosphate proceeded smoothly in the case of 6c, resulting in low enantioselectivity. It is noteworthy that 3-phenyl substituted lactol 6d reacted more smoothly under the asymmetric reaction conditions than under the racemic reaction conditions (Table 2, entry 4 vs. Table 4, entry 4). This result indicates that proper selection of the lactol-activating agent based on each substrate is important to realize high reactivity. The scope of pyruvic acid derivatives was also examined using 5-membered lactol 6b. Pyruvates 5b and 5c containing p-OH and o-NO2 substituents on the aromatic rings, respectively, exhibited high reactivity, but only moderate enantiomeric excess (entries 5 and 6). When α-methyl substituted pyruvic acid 5d was used, the yield was only 19% and the enantiomeric excess was 32% (entry 7).
In conclusion, we developed a novel catalytic method to construct functionalized isotetronic acid derivatives. The combined use of iron complex and 2-mercaptopyridine was crucial to obtain high reactivity. In addition, the developed method could be extended to an asymmetric variant by using chiral binaphthyl phosphate 8c instead of 2-mercaptopyridine. Further investigation to expand the substrate scope of this protecting group-minimal C–C bond-formation is on-going in our laboratory.
ACKNOWLEDGEMENTS
This work was supported by a Grant-in-Aid for Scientific Research on Innovative Areas ‘Advanced Molecular Transformations by Organocatalysts’ from MEXT (MK) and Grant-in-Aid for Young Scientists (B) from JSPS (YS).
References
1. (a) M. Suzuki, Y. Hosaka, H. Matsushima, T. Goto, T. Kitamura, and K. Kawabe, Cancer Lett., 1999, 138, 121; CrossRef (b) T. Namiki, M. Nishikawa, Y. Itoh, I. Uchida, and M. Hashimoto, J. Antibiot., 1987, 40, 1400; CrossRef (c) I. Cutzach, P. Chatonnet, and D. Dubourdieu, J. Agric. Food Chem., 1999, 47, 2837; CrossRef (d) A. C. S. Ferreira, J. C. Barbe, and A. Bertrand, J. Agric. Food Chem., 2003, 51, 4356; CrossRef (e) G. A. Schiehser, J. D. White, G. Matsumoto, J. O. Pezzanite, and J. Clardy, Tetrahedron Lett., 1986, 27, 5587; CrossRef (f) A. J. Pallenberg and D. White, Tetrahedron Lett., 1986, 27, 5591. CrossRef
2. (a) P. Lu and T. Bach, Angew. Chem., 2012, 124, 1287; CrossRef (b) Angew. Chem. Int. Ed., 2012, 51, 1261; CrossRef (c) A. Hinman and J. Du Bois, J. Am. Chem. Soc., 2003, 125, 11510. CrossRef
3. (a) D. Enders, H. Dyker, and F. R. Leusink, Chem. Eur. J., 1998, 4, 311; CrossRef (b) Y. Sun, H. Chen, Z. Li, Q. Wang, and F. Tao, Synthesis, 2008, 589; CrossRef (c) X. Ma, Z. Li, and Q. Wang, Heterocycles, 2010, 81, 1485; CrossRef (d) R. Dede, L. Michaelis, and P. Langer, Tetrahedron Lett., 2005, 46, 8129. CrossRef
4. Catalytic asymmetric dimerizations of pyruvic acid derivatives: (a) K. Juhl, N. Gathergood, and K. A. Jørgensen, Chem. Commun., 2000, 2211; CrossRef (b) P. Dambruoso, A. Massi, and A. Dondoni, Org. Lett., 2005, 7, 4657. CrossRef
5. Catalytic asymmetric cross aldol reactions using pyruvic acid derivatives: (a) N. Gathergood, K. Juhl, T. B. Poulsen, K. Thordrup, and K. A. Jørgensen, Org. Biomol. Chem., 2004, 2, 1077; CrossRef (b) J.-M. Vincent, C. Margottin, M. Berlande, D. Cavagnat, T. Buffeteau, and Y. Landais, Chem. Commun., 2007, 4782; CrossRef (c) D. Lee, S. G. Newman, and M. S. Taylor, Org. Lett., 2009, 11, 5486; CrossRef (d) B. Zhang, Z. Jiang, X. Zhou, S. Lu, J. Li, Y. Liu, and C. Li, Angew. Chem. Int. Ed., 2012, 51, 13159. CrossRef
6. Catalytic asymmetric reactions using pyruvic acid derivatives as nucleophiles: (a) K. Juhl, N. Gathergood, and K. A. Jørgensen, Angew. Chem. Int. Ed., 2001, 40, 2995; CrossRef (b) K. Juhl and K. A. Jørgensen, J. Am. Chem. Soc., 2002, 124, 2420; CrossRef (c) G. Lu, H. Morimoto, S. Matsunaga, and M. Shibasaki, Angew. Chem. Int. Ed., 2008, 47, 6847; CrossRef (d) A. Nakamura, S. Lectard, D. Hashizume, Y. Hamashima, and M. Sodeoka, J. Am. Chem. Soc., 2010, 132, 4036. CrossRef
7. Catalytic C-C bond forming reactions using unprotected sugars have been reported: (a) B. Voigt, U. Scheffler, and R. Mahrwald, Chem. Commun., 2012, 48, 5304; CrossRef (b) B. Voigt, A. Matviitsuk, and R. Mahrwald, Tetrahedron, 2013, 69, 4302; CrossRef (c) S. Kobayashi, T. Wakabayashi, and H. Oyamada, Chem. Lett., 1997, 26, 831; CrossRef (d) K. Toshima, G. Matsuo, T. Ishizuka, Y. Ushiki, M. Nakata, and S. Matsumura, J. Org. Chem., 1998, 63, 2307; CrossRef (e) K. Toshima, G. Matsuo, T. Ishizuka, M. Nakata, and M. Kinoshita, J. Chem. Soc., Chem. Commun., 1992, 1641; CrossRef (f) K. Toshima, G. Matsuo, and M. Nakata, J. Chem. Soc., Chem. Commun., 1994, 997; CrossRef (g) Y. Kimura, S. Ito, Y. Shimizu, and M. Kanai, Org. Lett., 2013, 15, 4130. CrossRef
8. E. Ertürk, M. Göllü, and A. S. Demir, Tetrahedron, 2010, 66, 2373. CrossRef
9. S.-L. Shi, X.-F. Wei, Y. Shimizu, and M. Kanai, J. Am. Chem. Soc., 2012, 134, 17019. CrossRef
10. Products derived from self-aldol reaction of 6a were not observed. This is likely due to low concentration of an enolizable aldehyde form of 6a compared to phenylpyruvic acid (5a) even in the presence of 2-mercaptopyridine. In addition, when methyl phenylpyruvate was used as a nucleophile, only a trace amount of the product was obtained. This result indicates that the carboxylic acid group is essential for the reaction.
11. C. G. Swain and J. F. Brown, Jr., J. Am. Chem. Soc., 1952, 74, 2538. CrossRef
12. Typical procedure of the nucleophilic addition of α−keto acids to lactols;
racemic reaction: Tetrahydrofuran-2-ol (6b) (12.2 µL, 150 µmol) was added to a suspension of iron trifluoroacetate complex (3.1 mg, 3.3 µmol), phenylpyruvic acid (5a) (16.4 mg, 100 µmol), and 2-mercaptopyridine (1.1 mg, 10 µmol) in dry DCE (400 µL). This suspension was stirred for 24 h at 60 ºC. To this mixture, DCE (2 mL), MeOH (500 µL) and DOWEX 50WX8 (400 mg) were added, and the mixture was stirred for additional 30 min. The mixture was filtered, and the filtrate was evaporated. The crude product was purified by flash chromatography (silica gel, DCM/MeOH = 100/1 to 50/1) to afforded 7ab (18.3 mg, 78% yield) as white solid.
asymmetric reaction: Tetrahydrofuran-2-ol (6b) (12.2 µL, 150 µmol) was added to a suspension of iron trifluoroacetate complex (3.1 mg, 3.3 µmol), phenylpyruvic acid (5a) (16.4 mg, 100 µmol) and 8c (8.2 mg, 20 µmol) in dry DCE (400 µL). This suspension was stirred for 24 h at 60 ºC. To this mixture, DCE (2 mL), MeOH (500 µL) and DOWEX 50WX8 (400 mg) were added, and the mixture was stirred for additional 30 min. The mixture was filtered, and the filtrate was evaporated. The crude product was purified by flash chromatography (silica gel, DCM/MeOH = 100/1 to 50/1) to afford 7ab (16.4 mg, 70% yield) as white solid. Enantiomeric excess was determined to be 91% by chiral HPLC analysis. DICEL CHIRALPAK IC, hexane/2-propanol 9/1, 1 mL/min. tR 28.2 min. (major), 34.9 min. (minor). [α]D25 -84.4 (c 0.91, MeOH) for 76% ee.
13. 7-Membered (6c) lactol mainly exists as aldehyde form.
14. Fe(OH)3 was prepared according to the following paper. H. Vrubel, T. Hasegawa, E. de Oliveira, and F. S. Nunes, Inorg. Chem. Commun., 2006, 9, 208. CrossRef
15. For representative reviews, see: (a) T. Akiyama, J. Itoh, and K. Fuchibe, Adv. Synth. Catal., 2006, 348, 999; CrossRef (b) T. Akiyama, Chem. Rev., 2007, 107, 5744; CrossRef (c) X. Yu and W. Wang, Chem. Asian J., 2008, 3, 516; CrossRef (d) M. Terada, Synthesis, 2010, 1929. CrossRef
16. Absolute configuration was determined by the Mosher ester analysis (I. Ohtani, T. Kusumi, Y. Kashman, and H. Kakisawa, J. Am. Chem. Soc., 1991, 113, 4092) of 11, which was derivatized from 7ab in 4 steps as shown below. Esterification of 10 with (R)- and (S)-MTPA-Cl gave the (S)-and (R)-MTPA esters (11a and 11b) respectively.