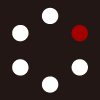
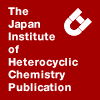
HETEROCYCLES
An International Journal for Reviews and Communications in Heterocyclic ChemistryWeb Edition ISSN: 1881-0942
Published online by The Japan Institute of Heterocyclic Chemistry
e-Journal
Full Text HTML
Received, 25th June, 2013, Accepted, 5th August, 2013, Published online, 7th August, 2013.
DOI: 10.3987/COM-13-S(S)45
■ Mosher's Amide-Based Assignment of the Absolute Configuration of Phantasmidine
Quan Zhou and Barry B. Snider*
Department of Chemistry MS 015, Brandeis University, 415 South Street, Waltham, MA 02454-9110, U.S.A.
Abstract
The absolute configuration of phantasmidine was assigned by analysis of the two conformers of the two Mosher amides. The expected shielding of the protons adjacent to the nitrogen and large long range shielding (~1 ppm) of the cyclobutane methylene protons in 16major and the pyridine protons of 16minor by the phenyl group of the Mosher amide allows the assignment of absolute configuration as shown in Schemes 4 and 5.In 1992 Daly isolated the much studied epibatidine (8) from the skin of the Ecuadorian poison frog Epipedobates anthonyi.1 In 2010 Fitch, Daly and co-workers isolated the minor, rigid, tetracyclic congener phantasmidine (7) from the same frog.2 The structure of phantasmidine was tentatively assigned spectroscopically from HPLC-purified 7 and the derived acetamide 17 with a total sample size of only ∼20 μg. We recently reported a short and efficient synthesis of racemic phantasmidine (7) that confirmed the structure assignment.3 Deprotonation of 2-fluoro-6-chloropyridine (1) at the 3-position with LDA and trapping of the resulting carbanion with DMF provided 2, which was elaborated to primary amide 3. Coupling of primary amide 3 with protected acyloin 4 gave racemic secondary amide 5, which was treated with potassium hydroxide in t-butanol to effect an intramolecular aldol reaction and nucleophilic aromatic displacement to afford tetracyclic pyrrolidinone 6, which was reduced with borane to give racemic phantasmidine (7) in 8 steps and 8% overall yield (see Scheme 1).
The coupling of amide 3 with 4 gave racemic 5, making this route unsuitable for the synthesis of enantiomerically pure phantasmidine. Fortunately, the two enantiomers were very readily separated by chiral HPLC on a Chiralcel OJ-H column with retention times of 29 and 44 minutes, making both enantiomers readily available for biological investigation.3 The optical rotation of the faster eluting enantiomer (+)-phantasmidine is [α]25D +70 (c 0.17, CH2Cl2) and that of the slower eluting enantiomer (-)-phantasmidine is [α]25D -77 (c 0.17, CH2Cl2). The two enantiomers of epibatidine (8) have very similar shapes and very similar biological activity.1 We expected that there would be a significant difference in the biological activity of the two enantiomers of the much more rigid tetracyclic congener phantasmidine making it important to assign the absolute configuration, which we report here.
Although (±)-phantasmidine is highly crystalline, we were unable to obtain suitable crystals of the pure enantiomers obtained by chiral HPLC. Initial attempts at resolving larger quantities and forming a crystalline salt suitable for X-ray crystallography by addition of 0.5 equivalent of various enantiomerically pure acids to racemic phantasmidine were also unsuccessful. We then turned our attention to the use of Mosher's amides as developed by Hoye, who prepared the diastereomeric Mosher's amides 9 and 10 from (2R,5R)-2,5-dimethylpyrrolidine and showed that the 1H NMR spectra are remarkably different as indicated in Scheme 2.4-6 Conformational studies indicated that the predominant conformer has the trifluoromethyl group syn to the carbonyl group as shown. The assignment was facilitated by the C2-symmetry of (2R,5R)-2,5-dimethylpyrrolidine which results in only a single amide conformer. One methyl group, presumably that adjacent to the carbonyl group absorbs at δ 1.30 and δ 1.32 in the two diastereomers. As expected, the methyl group shielded by the phenyl group in 10 (δ 0.10) is shifted upfield by 0.99 ppm from the methyl group adjacent to the methoxy group in 9 (δ 1.09). Similarly, the methine
hydrogen in 9 shielded by the phenyl group (δ 3.19) is shifted upfield by 1.24 ppm from the methine hydrogen adjacent to the methoxy group in 10 (δ 4.43).
We had some concerns regarding the application of this procedure to phantasmidine. (2R,5R)-2,5-Dimethylpyrrolidine is C2-symmetric so that there is only one amide conformer of 9 and 10. The Mosher's amides of phantasmidine 15 and 16 will exist in two amide conformers on the NMR time scale, as does N-acetylphantasmidine (17),2 making the assignment of absolute configuration more difficult. MMX calculations by PCMODEL 8.07 indicated that the major amide conformers 11major and 12major of the unsymmetrical (R)-2-methylpyrrolidine (R)-Mosher's amides 11 and (S)-Mosher's amide 12 with the large Ph(OMe)CF3C group adjacent to the smaller methylene carbon are about 2 kcal/mol more stable than the minor amide conformers 11minor and 12minor with the large Ph(OMe)CF3C group adjacent to the large methyl-substituted methine carbon (see Scheme 3). As expected, only one conformer corresponding to 11major and 12major was observed by 1H NMR spectroscopy for both diastereomers of the closely related triethylsilyloxymethylpyrrolidine Mosher's amides 13 and 14.4c The methine protons of 13 and 14 on C-2 have almost identical chemical shifts (Δδ = 0.02) whereas the methylene protons shifted significantly (Δδ = 0.23 and -0.41). This suggested that in both cases the carbonyl group is adjacent to the methyl-substituted methine proton whereas the major shielding factor, the phenyl group, is adjacent to the methylene group. Other 2-alkylpyrrolidine Mosher's amide spectroscopic data support this analysis.5
To our surprise, MMX calculations by PCMODEL 8.0 suggested that the large Ph(OMe)CF3C group is adjacent to the cyclobutane-substituted methine carbon in the major amide conformers (more stable by 0.9-1.2 kcal/mol) of phantasmidine Mosher's amides 15major and 16major rather than the methylene carbon as in 11major and 12major (see Scheme 4). Similar calculations indicate that the methyl group of phantasmidine acetamide (17) also prefers to be adjacent to the cyclobutane-substituted methine carbon by 1.1 kcal/mol. These calculations suggest that the methylene group of the cyclobutane fused to the pyrrolidine is smaller than a proton, whereas the C-2 methyl group of a pyrrolidine is obviously much larger than a proton. Apparently, the ~90° bond angles in the cyclobutane move the cyclobutane methylene carbon away from the nitrogen so that it is effectively smaller than a proton.
Acylation of readily available synthetic (±)-phantasmidine (7) with (R)-Mosher's acid chloride (prepared from the (S)-Mosher's acid)8 gave a mixture of diastereomers 15 and 16. The 1H NMR spectrum was complex with two amide conformers for each diastereomer. We were able to assign the peaks for each of the four amide conformers from the COSY spectrum of the mixture. Acylation of 0.5 mg of (-)-phantasmidine and 0.5 mg of (+)-phantasmidine with R-Mosher's acid chloride8 in CDCl3 gave the corresponding (-)-phantasmidine S-Mosher's amide 15 and (+)-phantasmidine S-Mosher's amide 16, respectively. The crude products were washed with 5% aq. HCl and used for spectroscopic analysis without further purification. The 1H NMR spectrum of the purified mixture of diastereomers 15 and 16 was used to exclude impurities due to unreacted Mosher's acid or Mosher's acid chloride.
A 3:1 mixture of amide conformers was observed for (-)-phantasmidine S-Mosher's amide 15. The methine hydrogen adjacent to the phenyl group in 15major (δ 4.30) is shifted upfield by 0.74 ppm from the methine hydrogen in 15minor (δ 5.04). The methylene hydrogen adjacent to the phenyl group in 15minor (δ 3.04) is shifted upfield by 0.91 ppm from the methylene hydrogen in 15major (3.95). These shifts allow us to assign the absolute configuration as shown and indicate that, as calculated, the methylene carbon is larger than the cyclobutane-substituted methine carbon, although the observed ΔG (0.65 kcal/mol) is smaller than the calculated ΔG (1.2 kcal/mol)).
A 4:1 mixture of amide conformers was observed for (+)-phantasmidine S-Mosher's amide 16. The methylene hydrogens of the cyclobutane adjacent to the phenyl group in 16major (δ 0.68, δ 0.87) are shifted upfield by 0.95 and 1.62 ppm from those in 16minor (δ 1.63, δ 2.49). The pyrrolidine methylene proton and two aryl protons that are adjacent to the phenyl group in 16minor (δ 3.78, δ 6.41, and δ 6.56) are also shifted upfield significantly (0.63, 1.07, and 0.35 ppm) from those in 16major (δ 4.41, δ 7.48, and δ 6.91). These shifts allow us to assign the absolute configuration as shown and indicate that, as calculated, the methylene carbon is larger than the cyclobutane-substituted methine carbon, although the observed ΔG (0.82 kcal/mol) is smaller than the calculated ΔG (0.9 kcal/mol).
The exceptionally large Δδ values established the absolute configuration of (-)-phantasmidine as shown in 15 and (+)-phantasmidine as shown in 16. The remarkably large shielding of the cyclobutane methylene group of 16major and the pyridine protons of 16minor by the Mosher's amide phenyl group is consistent with the MMX-minimized structures shown in Figure. 1. The close agreement of calculations and experiment on the relative amide conformer energies provides further support for this assignment.
Phantasmidine acetamide (17) showed a 2:1 mixture of amide conformers in the 1H NMR spectrum whose conformations were assigned from NOE data.2 The methine proton (δ 4.79) adjacent to the carbonyl group in the minor amide conformer 17minor is deshielded by the carbonyl group and shifted downfield 0.07 ppm from the methine proton adjacent to the methyl group in the major amide conformer 17major (δ 4.70). The methylene protons adjacent to the nitrogen were assigned based on the cis vicinal coupling constant (7.0-8.4 Hz, 20-30°) being larger than the trans vicinal coupling constant (3.2 Hz, 95-110°). The pseudoequatorial methylene hydrogen is in the deshielding cone of the carbonyl group and is shifted downfield to δ 4.08 in the major amide conformer from δ 3.96 in the minor amide conformer. The pseudoaxial methylene hydrogen is in the shielding cone of the carbonyl group and is shifted upfield to δ 4.02 from δ 4.32 in the minor amide conformer. The 2:1 mixture of amide conformers indicates that 17major is more stable by 0.41 kcal/mol, which is somewhat smaller than the calculated value of 1.1 kcal/mol, but does again indicate that the methylene group is larger than the cyclobutane-substituted methine carbon.
Both enantiomers of epibatidine have comparable biological activity.1 The absolute configuration of epibatidine was established as shown in 8 by chiral HPLC comparison with synthetic material of known absolute configuration.9 The biosynthetic pathways to epibatidine and phantasmidine are not known nor is it clear how they are biosynthetically related. The slower eluting (-)-phantasmidine ((-)-7) has the same absolute configuration as natural epibatidine at the asterisked carbons (see Scheme 5). We are unable to determine the absolute configuration of natural phantasmidine because a sample is no longer available. Surprisingly, preliminary results indicate that faster eluting (+)-phantasmidine ((+)-7) is considerably more biologically active than slower eluting (-)-phantasmidine.10 We believe that the assignment of the absolute configuration of phantasmidine based on the Mosher's amides is secure because of the magnitude of the chemical shift differences and we were carful to deal with the formal change that occurs when (S)-Mosher's acid is converted to the (R)-Mosher's chloride and back to the (S)-Mosher's amide. This raises several possibilities that can't be distinguished between at this time. Nature could make epibatidine and (+)-phantasmidine with opposite absolute configuration at the asterisked carbons, nature could make the less biologically active (-)- or racemic phantasmidine, or the absolute configuration of epibatidine could be misassigned.
In conclusion, the absolute configuration of phantasmidine was assigned by MMX analysis of the two conformations of Mosher amides 15 and 16 and their 1H NMR spectra. The expected shielding of the protons adjacent to the nitrogen and large long range shielding (~1 ppm) of the cyclobutane methylene protons in 16major and the pyridine protons of 16minor by the phenyl group of the Mosher amide allows the assignment of absolute configuration as shown in Schemes 4 and 5.
EXPERIMENTAL
Racemic Phantasmidine (S)-Mosher's Amide (15 and 16). A mixture of (S)-Mosher's acid (8.5 mg, 36 µmol) in 1 mL of hexanes was treated with 2 µL of DMF and then 10 µL (14.8 mg, 116 µmol) of oxalyl chloride at 25 °C. The reaction was kept at 25 °C for 30 min and filtered. The filtrate was concentrated to give crude (R)-(-)-Mosher's acid chloride as colorless oil, which was used directly without further purification.
A solution of racemic phantasmidine (7, 4 mg, 18 µmol) and diisopropylethylamine (30 µL, 170 µmol) in 0.2 mL of anhydrous CH2Cl2 was treated with a solution of the crude (R)-(-)-Mosher's acid chloride in 0.5 mL of anhydrous CH2Cl2 at 25 °C under N2. The reaction was stirred at 25 °C for 1 h and quenched with 2 mL of 5% aqueous Na2CO3. The resulting mixture was extracted with CH2Cl2 (3 mL × 3) and the combined organic extracts were dried (MgSO4) and concentrated. Flash chromatography of the residue on silica gel (CH2Cl2 to 100:1 CH2Cl2/MeOH) gave 6.1 mg (77%) of a 1:1 mixture of (±)-phantasmidine (S)-Mosher's amide diastereomers 15 and 16. One diastereomer exists as a 3:1 mixture of amide conformers and the other as a 4:1 mixture.
(S)-Mosher's Amide of the Slower Eluting (-)-Phantasmidine Enantiomer (15). The identical procedure using ~0.5 mg of (-)-phantasmidine gave a mixture of 15major and 15minor whose 1H NMR spectrum showed a 3:1 mixture of amide conformers. The spectral data described below were obtained by analysis of the spectrum of this crude diastereomer and the spectrum of the purified mixture of both diastereomers described above.
Partial data not including the phenyl protons for 15major: 1H NMR δ 7.49 (dd, 1, J = 7.6, 1.2), 6.93 (d, 1, J = 7.6), 4.74 (dd, 1, J = 12.8, 1.2), 4.30 (dd, 1, J = 8.4, 6.8), 3.95 (dd, 1, J = 12.8, 7.2), 3.75 (br d, 1, J = 7.2), 3.59 (q, 3, JH,F = 1.8, OMe), 2.42-2.32 (m, 2), 2.21 (br dd, 1, J = 12, 10.4), 1.74-1.66 (m, 1).
Partial data not including the phenyl protons for 15minor: 1H NMR δ 7.37 (presumed d, 1, assigned from COSY spectrum), 6.91 (d, 1, J = 7.6), 5.04 (dd, 1, J = 7.6, 7.6), 3.91 (dd, 1, J = 12.8, 2,1), 3.67 (br d, 1, J = 7.6), 3.28 (q, 3, JH,F = 1.8, OMe), 3.04 (dd, 1, J = 12.8, 7.6), 2.64 (br ddd, 1, J = 12, 10, 10), 2.54-2.46 (m, 1), 2.35-2.27 (m, 1), 1.44-1.36 (m, 1).
(S)-Mosher's Amide of the Faster Eluting (+)-Phantasmidine Enantiomer (16). A solution of (+)-phantasmidine (~0.5 mg, 2.2 µmol) and dry pyridine (1 µL, 12.5 µmol) in anhydrous 100 µL of CDCl3 was treated with (R)-(-)-Mosher's acid chloride (6.4 µmol, prepared as described above from the (S)-(-)-Mosher's Acid) in 100 µL of CDCl3 at 25 °C under N2. The reaction was kept at 25 °C for 1 h and diluted with 0.3 mL of dry CDCl3. The entire CDCl3 solution was transferred to an NMR tube for spectroscopic analysis. The 1H NMR spectrum of this diastereomer showed a 4:1 mixture of Mosher's amide conformers 16major and 16minor. The spectral data described below were obtained by analysis of the spectrum of this crude diastereomer and the spectrum of the purified mixture of both diastereomers described above.
Partial data not including the phenyl protons for 16major: 1H NMR δ 7.48 (dd, 1, J = 7.6, 1.2), 6.91 (d, 1, J = 7.6), 4.71 (dd, 1, J = 7.6, 7.2), 4.41 (dd, 1, J = 13.2, 1.7), 3.98 (dd, 1, J = 13.2, 8.0), 3.80 (br d, 1, J = 8.0), 3.34 (q, 3, JH,F =1.8, OMe), 2.23 (ddd, 1, J = 12.8, 11.2, 10.4), 2.02 (ddd, 1, J = 12.8, 9.2, 4), 0.91-0.83 (m, 1), 0.73-0.63 (m, 1).
Partial data not including the phenyl protons for 16minor: 1H NMR δ 6.56 (d, 1, J = 7.6), 6.41 (dd, 1, J = 7.6, 1.2), 5.25 (dd, 1, J = 7.6, 7.2), 3.88 (dd, 1, J = 12.8, 7.6), 3.78 (br d, 1, J = 12.8), 3.77 (q, 3, JH,F = 1.8, OMe), 3.63 (br d, 1, J = 7.6), 2.66 (ddd, 1, J = 12.8, 11.2, 10.4), 2.54-2.45 (m, 1), 2.35-2.27 (m, 1), 1.67-1.59 (m, 1).
ACKNOWLEDGEMENTS
We are grateful to the National Institutes of Health (GM-50151) for support of this work.
References
1. J. W. Daly, H. M. Garraffo, T. F. Spande, M. W. Decker, J. P. Sullivan, and M. Williams, Nat. Prod. Rep., 2000, 17, 131; CrossRef (b) F. I. Carroll, Biorg. Med. Chem. Lett., 2004, 14, 1889; CrossRef (c) J. W. Daly, Cell. Mol. Neurobiol., 2005, 25, 513; CrossRef (d) H. M. Garraffo, T. F. Spande, and M. Williams, Heterocycles, 2009, 79, 207. CrossRef
2. R. W. Fitch, T. F. Spande, H. M. Garraffo, H. J. C. Yeh, and J. W. Daly, J. Nat. Prod., 2010, 73, 331. CrossRef
3. Q. Zhou and B. B. Snider, Org. Lett., 2011, 13, 526. CrossRef
4. (a) T. R. Hoye and M. K. Renner, J. Org. Chem., 1996, 61, 2056; CrossRef and 2006, 71, 1754; CrossRef (b) T. R. Hoye and M. K. Renner, J. Org. Chem., 1996, 61, 8489; CrossRef (c) T. R. Hoye, M. K. Renner, and T. J. Vos-DiNardo, J. Org. Chem., 1997, 62, 4168. CrossRef
5. For applications to monosubstituted pyrrolidines and piperidines, see: (a) A. Jossang, A Melhaoui, and B. Bodo, Heterocycles, 1996, 43, 755; CrossRef (b) H. M. L. Davies, J. J. Matasi, L. M. Hodges, N. J. S. Huby, C. Thornley, N. Kong, and J. H. Houser, J. Org. Chem., 1997, 62, 1095; CrossRef (c) C. J. Dinsmore and C. B. Zartman, Tetrahedron Lett., 2000, 41, 6309; CrossRef (d) D. C. Beshore and C. J. Dinsmore, Tetrahedron Lett., 2000, 41, 8735; CrossRef (e) H. M. L. Davies and L. M. Hodges, J. Org, Chem., 2002, 67, 5683; (f) C.-Q. Kang, H.-Q. Guo, X.-P. Qiu, X.-L. Bai, H.-B. Yao, and L.-X. Gao, Mag. Reson. Chem., 2006, 44, 20; CrossRef (g) P. Vidal, C. Pedregal, N. Díaz, H. Broughton, J. L. Aceña, A. Jiménez, and J. F. Espinosa, Org. Lett., 2007, 9, 4123; CrossRef (h) D. T. Tran, H. D. T. Mai, V. C. Pham, V. H. Nguyen, M. Litaudon, F. Guéritte, Q. V. Nguyen, T. A. Tran, and V. M. Chau, Phytochem. Lett., 2013, 6, 79. CrossRef
6. For the related analysis of a 2-substituted pyrrolidine with a methoxyphenylacetamide, see: J. Gao, H. Haas, K. Y. Wang, Z. Chen, W. Breitenstein, and S. Rajan, Mag. Reson. Chem., 2008, 46, 17. CrossRef
7. PCMODEL 8 from Serena Software, Bloomington, IN was used for MMX calculations.
8. D. E. Ward and C. K. Rhee, Tetrahedron Lett., 1991, 32, 7165. CrossRef
9. (a) S. R. Fletcher, R. Baker, M. S. Chambers, R. H. Herbert, S. C. Hobbs, S. R. Thomas, H. M. Verrier, A. P. Watt, and R. G. Ball, J. Org. Chem., 1994, 59, 1771; CrossRef (b) A. P. Watt, H. M. Verrier, and D. O'Connor, J. Liq. Chromatogr., 1994, 17, 1257. CrossRef
10. A. A. Pandya, K. E. Panter, B. B. Snider, Y. Xiao, J. L. Yakel, Q. Zhou, and R. W. Fitch, manuscript in preparation.