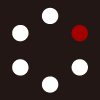
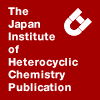
HETEROCYCLES
An International Journal for Reviews and Communications in Heterocyclic ChemistryWeb Edition ISSN: 1881-0942
Published online by The Japan Institute of Heterocyclic Chemistry
e-Journal
Full Text HTML
Received, 4th July, 2013, Accepted, 24th July, 2013, Published online, 30th July, 2013.
DOI: 10.3987/COM-13-S(S)82
■ Asymmetric Construction of Quaternary Stereogenic Centers via Auxiliary-Based SN2’ Reactions: A Case of 1,7-Relative Stereogenesis
Scott E. Denmark* and Lyndon K. Marble
245 Roger Adams Laboratory Box 18-5 , Department of Chemistry, University of Illinois at Urbana-Champaign, 600 South Mathews Avenue, Urbana, Illinois 61801, U.S.A.
Abstract
The stereoselective construction of quaternary stereogenic centers is described that employs an allylic substitution through the intermediacy of a chiral carbamate leaving group. Five carbamates with two substituents at the allylic terminus combined with phenylcopper or n-butylcopper to provide alkenes in moderate to good yields and with moderate to high enantioselectivities at the newly created quaternary stereogenic center. The E-carbamate with methyl and cyclohexyl substituents at the γ-terminus provided the highest yield (87%) and enantioselectivity (>99.9:0.1 er) of all the substitution patterns tested. The corresponding Z-carbamate gave the lowest enantioselectivity observed (80.0:20.0). Carbamates with other substituents (n-butyl and t-butyl) at the E-terminal position gave moderate yields and enantioselectivities of alkenes. Cinnamyl substrates also provided good yields of alkenes with moderate enantioselectivities. The origin of enantioselectivity is analyzed in the context of dimeric lithioheterocuprate structures.INTRODUCTION
The enantioselective synthesis of compounds containing quaternary stereogenic carbon centers continues to provide organic chemists with challenges for reaction design and development.1 The key issue in the construction of these centers is to identify reagent systems that can overcome the substantial steric encumbrance encountered in the carbon-carbon bond-forming event, while still providing an effective environment for obtaining high asymmetric induction. Although a number of elegant solutions have recently been devised for achieving this goal, certain types of reaction frameworks still present significant obstacles and provide platforms for novel reaction design and innovation in synthetic methods.
Because of their conformational flexibility and the need to control two-dimensional stereochemistry of double bonds, acyclic systems represent one of the most challenging substrates for the stereoselective introduction of quaternary carbons. Among the various methods for building acyclic quaternary stereocenters, two methods stand out that both involve allylic systems, namely carbonyl allylation (i.e. nucleophilic allyl partner)2 and allylic substitution (i.e. electrophilic allylic partner). Although successes have been recorded in both domains, the latter has been featured more prominently in recent years thanks to spectacular advances in organocopper chemistry3 and has shown greater versatility in substrate and reactant scope. As is so often the case in the development of new synthetic reactions, early investigations relied on substrate control of diastereoselectivity,4 which evolved to auxiliary control of diastereoselectivity with temporary modifiers5 which then gave way to catalyst control of enantioselectivity in achiral systems.6 In addition to the impressive advances in the enantioselective construction of quaternary stereogenic centers using copper-catalyzed allylic substitution reactions (SN2’ reactions),7 “copper-free” systems have been developed in recent years that employ chiral Lewis basic ligands that activate organozinc, -magnesium and -aluminum reagents for highly selective SN2’ reactions.8
In 1990 we reported the first example of copper-mediated, enantioselective SN2’ reactions that employed a chiral leaving group9 on the allylic system (Scheme 1).10 We demonstrated that three nucleophiles, methyllithium, n-butyllithium, and phenyllithium, could be delivered with complete site regioselectivity to the gamma position of chiral allylic carbamates to form tertiary carbon centers with 90:10 to 97.5:2.5 enantiomeric ratios. The ability to effect 1,7 stereoinduction was made possible by the use of a strongly coordinating carbamate that delivered the copper reagent to the γ-carbon of the allylic system.11 Since that time, the concept has been adopted by others and expanded to include phosphines as ligands for the copper on the leaving group.12 At that time we did not disclose our studies on the construction of quaternary stereogenic centers using the intramolecular delivery of a copper species. This paper describes the results from those studies.
To establish the utility of this process for the construction of quaternary stereogenic centers, six different substitution patterns at the gamma terminus of an allylic alcohol were examined in the reaction of chiral carbamates with n-butyl and phenylcopper reagents (Table 1). The E- and Z-carbamates (E)-(S)-1 and (Z)-(R)-1 with methyl and cyclohexyl substituents at the allylic terminus were made to test the influence of changing hydrogen to methyl at both the E- and Z-gamma positions. The carbamates (E)-(R)-2 and (E)-(R)-3 have the same Z-gamma substituent (methyl) as (E)-(S)-1 such that the effects of changing the E-gamma substituent could be examined. The final two carbamates to be used in this study were (E)-(S)-4 and (E)-(R)-5, both with an E-phenyl substituent. With optimized reaction protocols available for the reactions of methyl and n-butylcopper available from the previous study, the addition of organocopper reagents to cinnamyl substrates was investigated. The disubstituted carbamate (E)-(S)-4 was included as a test compound for studying the behavior of a cinnamyl system in this reaction before using the trisubstituted cinnamyl carbamate (E)-(R)-5.
RESULTS
Preparation of Carbamates. Carbamates (E)-(S)-1 and (Z)-(R)-1. Reformatsky reaction of cyclohexyl methyl ketone13 and ethyl bromoacetate followed by dehydration of the resulting hydroxy ester afforded the known14 ethyl 3-cyclohexyl acrylate in 65% yield as a 4/1 E/Z mixture. The esters (E)-6 and (Z)-6 were separated by exhaustive chromatography and were then reduced separately with diisobutylaluminum hydride to afford alcohols (E)-7 and (Z)-7 as colorless liquids. The alcohols were coupled with 2-methoxy-1-(1-naphthyl)ethyl isocyanate 8 in refluxing toluene to yield the E and Z carbamates (E)-(S)-1 and (Z)-(R)-1 in 88% and 92% yield, respectively (Scheme 2). The configurational purity was established for the esters and alcohols by capillary gas chromatography to be 99% for the E isomer and 96% for the Z isomer. The configurational assignment was determined by 1H and 13C NMR chemical shifts.15
Carbamates (E)-(R)-2 and (E)-(R)-3. The syntheses of carbamates (E)-(R)-2 and (E)-(R)-3 are shown in Scheme 3. Reaction of 1-hexyne with trimethylaluminum in the presence of zirconocene dichloride16 followed by trapping the intermediate vinylalane ethyl chloroformate afforded ester 9 as a 97:3 E/Z mixture (capillary GC analysis) in 62% yield. Reduction of 9 with DIBAL-H and coupling of alcohol 10 to isocyanate (R)-8 provided carbamate (E)-(R)-2 in 97% yield. Ethyl 3,4,4-trimethylpentenoate was synthesized by literature procedures17 in 35% yield (E/Z, 99:1) from pinacolone. Reduction of the ester with DIBAL-H afforded alcohol18 11 in 99% yield and coupling to (R)-8 provided the carbamate (E)-(R)-3 in 90% yield. The configurational purity of the esters and alcohols was established by capillary gas chromatography and the configurational assignments for the esters was made by comparison of 13C NMR chemical shift data as described above.
Carbamates (E)-(S)-4 and (E)-(R)-5. Carbamate (E)-(S)-4 was synthesized from cinnamyl 4-nitrophenyl carbonate and amino ether (S)-12 in refluxing toluene in 70% yield. Carbamate (E)-(R)-5 was synthesized from (E)-3-phenyl-2-buten-1-ol 13 and isocyanate (R)-8 in refluxing toluene in 93% yield (Scheme 4). Alcohol 13 was obtained from acetophenone by a Horner-Emmons reaction14c and DIBAL-H reduction of the resulting ester, ethyl (E)-3-phenyl-2-butenoate.19
Cuprate Reactions. Cuprate Reactions of (E)-(S)-1 and (Z)-(R)-1 with Phenylcopper. Carbamates (E)-(S)-1 and (Z)-(R)-1 were allowed to react with two equivalents phenylcopper in the normal addition sequence (lithio carbamate added to freshly prepared phenylcopper) and the results are listed in Table 2.20 The reaction with (E)-(S)-1 was the fastest and most complete to be observed among all of the substrates, affording (R)-14 in 87% yield with 100% conversion (entry 1). Remarkably, the reaction was completely selective for the R enantiomer (>99.9:0.1)! The challenge associated with many manipulations is seen in the lower yield for the duplicate run with (E)-(S)-1 which afforded (R)-14 in only 67% yield, but still with excellent enantioselectivity (entry 2). The reasons for the discrepancies in yield between the two runs was due to a change in the nature of the phenylcopper reagent for entry 3 as indicated by a change in its appearance from the usual yellow-black slurry to a green-grey mixture.
The two reactions of (Z)-(R)-1 with two equivalents phenylcopper provided (R)-14 in 68% and 45% yields, respectively. The same isomer of 14 was obtained as in the reactions with (E)-(S)-1 because of the switch of both double bond geometry and auxiliary configuration. The enantiomeric excess was lower than with the E-isomer (avg. = 80:20 er). This was the first indication that the nature of R1 also has a significant effect on the enantioselectivity.
Cuprate Reactions of Carbamates (E)-(R)-2 and (E)-(R)-3. The results of the cuprate reactions of carbamates (E)-(R)-2 and (E)-(R)-3 with two equivalents of phenylcopper under normal addition conditions are listed in Table 3. The reaction of (E)-(S)-1 is included for reference and the results of the cuprate reactions are listed by increasing order of substitution at the delta carbon (primary for R1 = n-butyl; secondary for R1 = cyclohexyl; tertiary for R1 = t-butyl).
The reaction of (E)-(R)-2 with two equivalents of phenylcopper yielded alkene (R)-16 with high site selectivity in 74% and 66% yields, respectively, for the two experiments listed in entries 1 and 2. The reaction conversions were 82% and 76%, respectively for the two runs with percent recovery of the auxiliary (R)-12 at 80% and 64%, in close agreement with the yields of alkene. The enantiomeric ratios were 85.0:15.0 and 88.5:11.5 favoring the R-isomer, considerably lower than for the reaction of (E)-(S)-1 in which R = cyclohexyl (entry 3). The configuration of the product alkene 16 was R rather than S simply because of a priority change between the R1 group and the vinyl substituent; but delivery to the Re face of the carbamate was still predominant.
The yields of alkene (R)-1821 for the reactions of carbamate (E)-(R)-3 were 45% to 46% for two individual runs; the difference in reaction concentrations had no effect on the results. Recoveries of the auxiliary coincided well with the yields of alkenes; however, the carbamate was not recovered in pure form. The enantioselectivities for the reactions were 84.0:16.0 and 82.5:17.5 for the R-isomer, again because of a priority change.
Cuprate Reaction of Carbamate (E)-(S)-4. Carbamate (E)-(S)-4 was allowed to react with two equivalents methylcopper according to the normal addition protocol to afford (R)-3-phenyl-1-butene, (R)-20 in 76% yield with a 79% recovery of the auxiliary (Scheme 5). Not all of the carbamate was deprotonated (the anion was very insoluble in ether) so the yield was based on the amount of lithiocarbamate transferred to the methylcopper slurry. The enantiomeric composition of (R)-20 was 89.5:10.5, somewhat lower than the 95.0:5.0 selectivities generally observed in methylcopper additions to aliphatic allylic carbamates previously.10 The facial selectivity was the same as that seen in methylcopper additions described previously which demonstrated that (S)-1-naphthylglycinol auxiliary (S)-12 delivered methyl to the Re face of the olefin.
Cuprate Reaction of Carbamate (E)-(S)-5. The reaction of carbamate (E)-(R)-5 with two equivalents n-butylcopper was designed to compare the addition of an n-butyl group to a conjugated substrate with addition of a phenyl group to a non-conjugated substrate, (E)-(R)-2. The two reactions were expected to produce enantiomers of alkene 16 because the auxiliary configuration was R for both 2 and 5. Reaction of (E)-(R)-5 with two equivalents n-butylcopper by the normal addition protocol afforded (S)-16 in 41% and 52% yield for the two experiments. The recoveries of carbamate were 27% and 30% with 62% and 70% of recovered auxiliary, respectively, for the two experiments. The γ-selectivity was somewhat diminished (90:10 and 94:6) due to difficulty in forming the anion, Li+(R)-5–, which was particularly insoluble in ether even at 10 ˚C.
The enantiomeric excesses were 86.0:14.0 and 84.5:15.5 favoring the S-enantiomer for the two experiments. These results are similar to the delivery of phenylcopper to carbamate (E)-(R)-2, 85.0:15.0 and 88.5:11.5 (see Table 2), but with the opposite configuration. For the reactions of both (E)-(R)-2 and (E)-(R)-5, Si face addition was the predominant direction for nucleophile delivery. Thus, both enantiomers of 16 can be formed by the proper combination of nucleophile and substrate (Scheme 6).
DISCUSSION
Cuprate Additions. The reaction of carbamate (E)-(S)-1 was the fastest and most selective of any cuprate reaction observed in this study project, affording alkene (R)-14 in 87% yield with complete enantioselectivity selectivity for the R isomer. Furthermore, the Z-carbamate (Z)-(R)-1 gave the a considerably lower selectivity in the delivery of the phenyl nucleophile.
Changing the R1 group from cyclohexyl to n-butyl and t-butyl decreased the enantioselectivities dramatically. Replacement of cyclohexyl must have changed the nature of the reactive complexes such that there was less difference between the most accessible cuprate complex and the next most favored structure. The presence of t-butyl apparently hindered approach of the side chain to the cuprate cluster slowing the reaction. One observation about the use of phenylcopper in the section on quaternary center synthesis is that the yields varied considerably with some substrates due to the inconsistent formation of the reagent. Even though all experimental details were carefully controlled and the reagent was prepared in exactly the same fashion each time, there were differences in the appearance of some mixtures. The color of the phenylcopper is usually yellow-black (suspension in Et2O) but on occasion it would be a green-grey slurry, indicative that the reagent had not formed in the same fashion.
The reactions of the cinnamyl substrates gave moderate yields of the respective alkenes. The delivery of n-butyl to carbamate (E)-(R)-5 was slower than the corresponding addition of phenyl to the heptenyl substrate (R1 = n-Bu, (E)-(R)-2) perhaps due to the lower reactivity of the cinnamyl substrates.
Enantioselectivity. The reactions of the lithio carbamates of disubstituted allylic alcohols in the preceding study provided enantioselectivities in the delivery of methyl, n-butyl and phenyl in the range of 94.0:6.0 to 97.5:2.5.10 Changing the nucleophile and thus necessarily changing the nature of the reactive species had only a minor effect on the selectivity (Scheme 1). Reactions of the trisubstituted allylic alcohols in the synthesis of quaternary centers gave an even wider variation of enantioselectivities in additions of phenyl and n-butyl nucleophiles. Thus it would appear that the structure of the reactive cuprate and thus the observed enantioselectivities must be different for each substrate. In all cases, the R-configured auxiliary always led to front face attack and the S-configured auxiliary, led to products from predominant back-face attack regardless of the nature of either R1, R2 or double bond configuration (Figure 1). The only difference between the reactions of E- and Z-carbamates with the same configuration of auxiliary is in the absolute configuration (and degree of enantiomeric purity) of the product alkene. This reversal of configuration on the change from E to Z is a result of the interchange of the group at R1 and R2, and not from reversal of facial selectivity.
Mechanistic Hypotheses. The following discussion about the mechanism is based on the current status of mechanistic thinking for the conjugate addition of organocuprate reagents to enones in as well as SN2’ reactions22 combined with recent structural studies on organocopper reagents.23 The initial interaction between an unsaturated carbonyl compound and a dialkylcuprate has been proposed to be a d-π* copper-olefin interaction. This proposal is experimentally supported by reports that describe copper complexes with olefins that have been isolated and characterized.24 This type of association has been observed in solution by spectroscopic methods (1H and 13C NMR) in the reactions of dimethylcuprate with cinnamate esters.25 An initial complex is seen at -90 ˚C in the 13C spectrum when the cuprate and enoate are mixed. This complex is proposed to be the copper-olefin association on the basis of significant upfield shifts of the resonance of carbons 2 and 3 in the cinnamate ester (Scheme 7). The direction of change in chemical shifts is in the same as those observed for the isolated copper-olefin complexes24 but the magnitude was much greater due to the more electron rich nature of the copper atom in the cuprate. Additionally, NMR and rate studies26 implicated the presence of a fleeting intermediate which has been assigned to the β-copper-(III) intermediate i (Scheme 7). Recently Rapid Injection NMR structural evidence for the existence of this intermediate has been provided.27
The mechanism for the SN2’ reaction proposed by Goering11b for coupling of N-phenyl carbamates with organocopper reagents involves an intramolecular delivery of the nucleophile (Scheme 8). This simplistic picture used to describe the intramolecular delivery of the copper reagent could also accommodate an initial d-π* interaction as the first association between the olefin and the copper atom. Similar to steps shown in Scheme 7, a β-copper-(III) intermediate could be produced in the first bond forming step. Next, reductive elimination from this intermediate would then give product. The carboxylate expulsion is intimately coupled with the formation of the copper(III) intermediate. This synchronous motion imposes conformational constraints on the approach of the copper atom and it ligands since proper orbital alignment of the breaking C-O bond is required for addition to occur. Thus, any explanation of the stereochemical outcome of these cuprate reactions must involve structures that have the proper orbital alignment and take into account the interactions between the substituents about the nitrogen and those on the olefin since this type of mechanism places them in close proximity. The discussion of the next section proposes a cuprate structure for the reactions of chiral allylic carbamates based on this intermolecular delivery mechanism. A π-allylcopper(III) species has been proposed in displacement of allylic acetates wherein the leaving group is not associated with the copper.22c
Proposed Cuprate Structure. Structures of diorganocuprate(I) species determined either crystallographically of computationally invariably display a linear C–Cu–C coordination geometry. Moreover, cuprates are generally found as dimeric or higher oligomeric structures. Calculations by Whangbo on the structure of lithium dimethylcuprate have indicated that a dimeric structure with a square planar geometry is 39 kcal/mol more stable than the tetrahedral alternative.28a The complex contains a tetrametallic central cluster of alternating copper and lithium atoms in a planar array. The methyl groups are bound to copper with no interaction with the lithium atoms. Ashby and Watkins28b have shown by ebullioscopic measurements and 1H NMR studies that lithium dimethylcuprate is dimeric in diethyl ether and THF solutions and is not in equilibrium with other species in these solvents.
van Koten has published numerous reports on the structures of aryl-metal complexes with copper(I) salts.23 The X-ray analyses revealed that these structures contain a central tetrametallic cluster of alternating copper and lithium atoms. The structure of the complex of 2-(dimethylaminomethyl)phenyllithium with copper iodide is shown in Figure 2. The aryl group is bound to copper by the sp2 orbital of the ipso carbon of the benzene ring with significant p-orbital interaction with the lithium atom.29 The dimethylaminomethyl ligand is bound to one lithium atom coordination site and the side chain of another dimethylamino group is bound at the other site on lithium. Thus, both copper and lithium are tetracoordinate in which lithium is approximately tetrahedral and the ligands at copper are approximately in the same plane. The central cluster is not square, due to the longer Cu – Li distance and a shorter Cu – Cu distance. Several different complexes of aromatic lithium compounds containing heteroatomic side chains with copper iodide have been examined by van Koten and have similar structures in solution,29b with the aryl ring bound to two metal atoms (one copper and one lithium) and the coordinating side chain bound to lithium.
This general dimeric structure has been proposed by Dieter for the reaction of modified methylcuprate(I) reagents with enones (Scheme 9).30 Chiral ligands derived from L-proline were examined which contained side chains with oxygen, nitrogen and sulfur atoms. Of those tested, (S)-2-(methoxymethyl)pyrrolidine was the best ligand for obtaining good enantioselectivities. A C2-symmetric dimeric complex is proposed to rationalize the high enantioselectivity observed in the addition of methylheterocuprate to 2-cyclohexenone. This selectivity is rationalized by invoking a face to face approach of the enone to the plane of the metallic cluster so as to minimize non-bonded interactions between the hydrogens at C(5) and C(6) of 2-cyclohexenone with the pyrrolidine ring.
Rossiter employed chiral α-aminomethylbenzenemethanamines as auxiliaries in the additions of organocopper reagents to enones.31 Examination of several chiral amines and chiral diamines revealed (S)-N-methyl-2-piperidinyl-1-phenylethylamine provides the highest selectivity (98.5:1.5 er) in the addition of phenylcopper to 2-cyclohexenone (Scheme 10). The proposed structure for the chiral reagent is very similar to that proposed by Dieter. The amine anion is bound to copper in a dimeric complex which is C2-symmetric. The piperidine nitrogen occupies a coordination site of lithium with a solvent molecule presumably located at the other coordination site. The ketone approaches the complex in a face-to-face approach with prior coordination of the oxygen to lithium.
On the basis of these precedents the proposal for the heterocuprate structure for the intramolecular reactions with chiral allylic carbamates discussed in this work is shown in Figure 3. The key features that correspond to those in the previously proposed structures are the following: (1) the anionic nitrogen is bound to copper that has the transferable ligand as the other anionic group, (2) the methoxy group is coordinated to lithium, with the fourth site at lithium occupied by a solvent molecule, (3) the aromatic ring is shown occupying a pseudo-equatorial position on the chelate ring which places it in a position to block the underside of the complex and prevent olefin approach to that surface of the cuprate cluster, (4) the complex is drawn with C2-symmetry although it need not take up this exact form in either the ground state or reactive conformations. This is not necessarily the reactive species but is a proposal for the cuprate cluster which could precede it on the reaction pathway.
The wide variation in observed enantioselectivities indicates that the reactive complex must be more complicated than the simple picture of the mixed heterocuprate shown in Schemes 9 and 10 and probably more complex than the dimeric cluster depicted in Figure 3 as well. For each substrate, the enantioselectivity arises from differential complexation of the faces of the olefin with the different surfaces of the cuprate cluster (Figure 4, only half of the dimeric cluster is shown for clarity). The olefin approaches the cluster to initiate a d-π* interaction between the copper atom and the double bond as proposed in Scheme 8. Combining the stabilizing effect of this orbital overlap with the least sterically demanding approach to the allylic system gives rise to the differences in the rates and enantioselectivities observed in this reaction.
The enantioselectivity afforded in each reaction is dependent on the relative energies of the reactive complexes and each can be different depending upon the substitution pattern on the alkene. The four limiting diastereomeric complexes A-D for carbamate (E)-(S)-1 are shown in Figure 4. The factors that contribute to the energy of these complexes must be evaluated to understand which is most likely to lead to the observed product. The two defining interactions that contribute to the energy of the complexes are highlighted in these depictions: (1) the d-π* interaction which has a stereoelectronic component (bolded Ph–Cu moiety) and steric interactions of the alkene substituents with the ligand framework. From a stereoelectronic perspective, the π* orbital of the alkene must achieve a parallel alignment with the d symmetry orbital of the HOMO of the cuprate.22 This condition is met only in structures A and D because conformational restrictions of the tethered allylic alcohol limit the ability of the alkene to orient with optimal overlap. From a steric perspective, placing the smaller substituent (Me) over or under the lithio cuprate chelate ring minimizes unfavorable non-bonded interactions which is achieved in structures A and D. Thus, a first order analysis easily eliminates structures B and C but cannot differentiate structures A and D. For this purpose, one needs to consider the steric congestion above and below the lithio cuprate chelate ring. The bottom face of the Cu-Li-Cu-Li rhombus contains the hydrogens on the critical stereogenic center of the ligand whereas the top face contains the naphthyl substituent. Although depicted as a chair, this ring is likely much more flattened thus bringing the naphthyl substituent more effectively into the upper half space of the plane. This difference would disfavor approach of the alkene to the top face of the lithio cuprate thus allowing the conclusion that structure A should be the most favorable arrangement. On the basis of this stereochemical model, the S enantiomer of carbamate (E)-1 should lead to the formation of the R enantiomer of the product 14 which is in fact observed experimentally.
The same first order analysis can be done for each of the other substitution patterns of olefin substitution (in both the disubstituted and trisubstituted substrates) to explain the observed enantioselectivities. However, the choice of the lowest energy diastereomeric complex can only be done after careful study of all four diastereomeric complexes available to each substrate. In fact the same complex does not have to be the favored for similar carbamates (i. e., compare results with (E)-(R)-3 and (E)-(S)-1) since the enantioselectivity is directly related to the energy distribution of the four complexes for each substrate.
The course of reaction is very sensitive to the exact nature of the olefin substitution and since the selectivities are the result of manifold complex interactions between the two parts of the substrate, it is difficult to say with any certainty what is the actual structure of the reactive intermediate. Furthermore, altering the nucleophile necessarily changes the solvent system for the reaction and this may be responsible for some of the variations observed in the enantioselectivities. Because these reactions are always heterogeneous mixtures, any selectivity may be the result of interactions of higher oligomers on the surface of the alkylcopper and interpretations about the origins of enantioselectivity would be difficult at best. Much more study is required to determine the effects of other changes in substrate structure so that a better understanding of the important interactions may be gained.
CONCLUSIONS
The reactions of carbamates (E)-(S)-1 through (E)-(R)-5 with either phenylcopper or n-butylcopper afforded alkenes containing quaternary centers with modest to high enantiomeric enrichments depending on the substitution pattern at the alkene. This process represented the first example of the construction of stereogenic quaternary centers in which the auxiliary is expelled during the addition step. Carbamate (E)-(S)-1 provided the highest yield (87%) and the highest enantioselectivity of all substrates examined. The corresponding Z-carbamate (Z)-(R)-1 gave the lowest selectivity of the trisubstituted carbamates surveyed. Other substituents about the double bond did not affect the enantioselectivity to a significant degree.
The reactions of chiral carbamates is proposed to occur through a dimeric cuprate cluster in which the olefinic side chain can approach the cluster through one of four diastereomeric orientations. The selectivity of the reaction is dependent on the relative energies of the possible complexes available to the substrate. Nothing more definite can be concluded at this time about the structure of the complex since no structural data are available. However, the origin of selectivity depends on the size of the substituent at the stereogenic carbon atom and the presence of a methoxy group beta to the amino nitrogen.
EXPERIMENTAL SECTION
Experimental
General Experimental. 1H-NMR and 13C-NMR spectra were recorded on either a Varian XL-200 (200 MHz 1H, 50.4 MHz 13C), General Electric QE-300 (300 MHz 1H, 75.5 MHz 13C), Nicolet NTC-360 (360 MHz 1H, 90.6 MHz 13C), or General Electric GN500 (500 MHz 1H, 125.8 MHz 13C) spectrometers in deuterochloroform with tetramethylsilane as internal standard (δ = 0.00 ppm for 1H) or chloroform (δ = 7.26 ppm for 1H, 77.0 for 13C), acetone-d6 (δ = 2.04 ppm for 1H, δ = 206 ppm for 13C), dimethyl sulfoxide-d6 (δ = 2.50 ppm for 1H) or methanol-d4 (δ = 3.30 ppm for 1H; δ = 49.0 ppm for 13C) with the solvents as internal standards. 19F NMR spectra were recorded on the Nicolet NTC-360 spectrometer (339 MHz 19F) with an external standard of fluorotrichloromethane. Chemical shifts are given in ppm (d); multiplicities are indicated by s (singlet), d (doublet), t (triplet), q (quadruplet), m (multiplet), or br (broadened). Coupling constants, J, are reported in hertz (Hz). Assignments of 1H and 13C resonances for most compounds containing the 1-naphthyl ring are supported by APT, HETCOR and/or COSY spectra. Infrared spectra were obtained on an IBM Instruments Inc. IR/32 FT-IR spectrometer in carbon tetrachloride solution, chloroform solution, neat or as a KBr pellet; on rare occasions a Nicolet 700 Series FT-IR equipped with Kennedy Model 9000 data station was used and is so noted. Peaks are reported in cm-1 with the following relative intensities: s (strong, 67-100%), m (medium, 34-67%), w (weak, 0-33%). Mass spectra were recorded on a Finnegan MAT CH-5 spectrometer for electron impact (EI) with ionization voltages of 10 and 70 eV, or on a Finnegan MAT-731 spectrometer for field ionization (FI) mass spectra. Data are reported in the form m/z (intensity relative to base = 100). Elemental analyses were performed by the University of Illinois Microanalytical Services Laboratory. Optical rotations were obtained on a Jasco DIP-360 Digital Polarimeter and are reported as [α]D24, concentration (g/dL) and solvent.
Melting points (mp) were obtained on a Thomas Hoover capillary melting point apparatus and are corrected. Bulb to bulb distillations were performed using a Buchi GKR-50 Kugelrohr, with boiling points (bp) refer to air bath temperatures which are uncorrected. Analytical TLC was performed on 0.2 mm Merck Kieselgel 60 glass backed silica plates with F-254 indicator. Visualization was accomplished with UV light, I2, ninhydrin solution, 10% ethanolic PMA solution or bromocresol green solution. Flash column chromatography was performed using 32-63mm silica gel (Woelm) by the method of Still.32 Dry column chromatography was performed according to the method of Harwood.33 Solvents for extraction and chromatography were technical grade and were distilled from the indicated drying agents: hexane, pentane, dichloromethane (CaCl2); diethyl ether, methyl t-butyl ether (CaSO4/FeSO4); ethyl acetate (K2CO3). Ozonations were performed with a Welsbach Model T-816 Ozonator. Analytical gas chromatography was performed on a Varian 3700 Gas Chromatograph or a Hewlett Packard 5890A Gas Chromatograph each fitted with a flame ionization detector and programmable temperature control. The carrier gas was H2 (30 mL/min) and the columns employed were: A) HP-5 (5% phenyl methyl silicone), 50 m or B) HP-1 (crosslinked methyl silicone), 50 m. High pressure liquid chromatography (HPLC) was performed on a Hewlett-Packard HP 1090 Liquid Chromatograph with a Perkin-Elmer LC-75 variable wavelength UV detector. Retention times (tR) and integrals were obtained from a Hewlett Packard 3390A recorder. The columns used were: A) 25 cm by 4.5 mm, 5mm Pirkle Covalent L-N-2-Naphthylalanine reversible chiral column purchased from Regis Chemical Company, and B) 25 cm by 4.5 mm, 5mm non-commercial Pirkle Covalent column derived from (S)-6,7-dimethyl-1-naphthalenemethanamine. All determinations with column B were performed by Dr. W. H. Pirkle. Constant temperature baths (n-butylcopper additions) were maintained by a Neslab Cryocool Immersion Cooler CC-100 II cooling system with recirculating pump.
Ethereal methyllithium was titrated by the method of Gilman.34 Phenyllithium and n-butyllithium were titrated against dry diphenylacetic acid to the yellow dianion endpoint35 in dry THF at 0 °C. Copper(I) iodide was purified by the method of Sum and Wieler.36 Copper(I) bromide dimethyl sulfide complex was prepared according to the procedure of Wuts.37 All reactions were performed in an oven-dried (125 °C) or flame dried apparatus under dry N2 (P2O5). All solutions were magnetically stirred unless otherwise stated. Brine refers to a saturated solution of sodium chloride; NH4Cl refers to a saturated solution whose pH had been adjusted to 7.0 with concentrated NH4OH.
Compounds Prepared by Literature Procedures. The following compounds were prepared by literature procedures. 2-Cyclohexyl-phenylacetic acid,38 methyl (Z)-3-cyclohexyl-2-propenoate,39 ethyl 3-cyclohexyl-3-hydroxybutanoate,13 ethyl (E)-3-cyclohexyl-2-butenoate,14 ethyl (E)-3-methyl- 2-heptenoate,40 ethyl 3-hydroxy-3,3,4-trimethylpentanoate,18 ethyl (E)-3,4,4-trimethyl-2-heptenoate,18 ethyl 3-acetoxy-3,4,4-trimethylheptanoate,18 ethyl (E)-3-phenyl-2-butenoate (ethyl b-methylcinnamate)14c (E)-3-phenyl-2-buten-1-ol,21 (S)-(-)-2-methoxy-1-(1-naphthyl)ethyl isocyanate.10
Preparation of Ethyl (E)-3-Cyclohexyl-2-butenoate (E)-6) and Ethyl (Z)-3-Cyclohexyl-2-butenoate ((Z)-6). A 100-mL, three-necked flask equipped with a stirring bar, thermometer, addition funnel and gas control valve was flame-dried under vacuum (0.10 mm Hg) and filled with dry nitrogen (P2O5). The flask was charged with sodium hydride (0.516 g, 21.5 mmol, hexane washed 2x) and evacuated and filled with dry nitrogen again. Dry THF (25 mL) was added to the flask and the addition funnel was charged with triethyl phosphonoacetate (4.82 g, 21.5 mmol) and dry THF (20 mL). The phosphonoacetate was added to the hydride suspension at +6 ˚C over 40 min (1 mL THF rinse). The resulting solution was stirred for 1 h at room temperature and a solution of cyclohexyl methyl ketone (1.37 g, 10.9 mmol) in THF (11 mL) was added at room temperature over 15 min. After 18 h at room temperature, the reaction was poured into Et2O (100 mL) and adding H2O (50 mL). The layers were separated and the aqueous phase extracted with Et2O (2x30 mL). The organic layers were combined, dried (Na2SO4) and concentrated in vacuo. The residue was purified by column chromatography on silica gel with 2% Et2O in pentane to afford 490 mg (25%) of (E)-6 and 250 mg (16%) of the (Z)-6. Each was distilled separately to afford analytically pure colorless oils. Analytical data from (E)-6: bp 95 ˚C (0.4 mm Hg, air bath temperature); 1H NMR (300 MHz, CDCl3): 5.58 (s, 1 H, HC(2)), 4.07 (quart, J = 7.5, 2 H, H2C(9)), 2.07 (s, 3 H, H3C( 8)), 1.90 (m, 1 H, HC(4)), 1.70 (m, 5 H, HeqC6H11), 1.21 (t, J = 7.5, 3 H, H3C(10)), 1.29-1.06 (m, 5 H, HaxC6H11); 13C NMR (75.5 MHz, CDCl3): 167.08 C(1), 164.67 C(3), 113.75 C(2), 59.23 C(9), 48.55 C(4), 31.19 C(5), 26.27 C(6), 25.98 C(7), 17.18 C(8), 14.18 C(10); IR (neat): 2979w, 2928s, 2855m, 1715s, 1642m, 1447m, 1395w, 1370w, 1341w, 1306w, 1264w, 1211s, 1148s, 1094w, 1046m, 855m; MS (70 eV): 196 (M+, 57), 181 (30), 151 (71), 150 (19), 149 (10), 135 (29), 128 (12), 123 (52), 122 (34), 121 (20), 115 (18), 111 (11), 109 (28), 108 (64), 107 (25), 102 (17), 95 (34), 93 (29), 91 (11), 87 (29), 82 (19), 81 (82), 80 (12), 79 (42), 77 (14), 69 (50), 68 (15), 67 (97), 65 (11), 55 (59), 54 (16), 53 (22), 43 (21), 41 (100); Anal. Calcd for C12H20O2: C, 73.43; H, 10.27. Found: C, 73.46; H, 10.31; TLC: Rf 0.25 (hexane/EtOAc, 19:1); GC: tR: 20.64 min (HP-1; 75 ˚C (5 min), 10 ˚C/min, 200 ˚C (10 min)). Analytical data from (Z)-6: M. W.: 196.29; bp 65 ˚C (0.3 mm Hg, air bath temperature); 1H NMR (300 MHz, CDCl3): 5.58 (s, 1 H, HC(2)), 4.13 (q, J = 7, 2 H, H2C(9)), 3.62 (tt, J = 11.5, 3.2, 1 H, HC(5)), 1.80 (s, 3 H, H3C( 4)), 1.80-1.50 (m, 5 H, HeqC6H11), 1.50-1.09 (m, 5 H, HaxC6H11), 1.27 (t, J = 7, 3 H, H3C(10)); 13C NMR (75.5 MHz, CDCl3): 166.23 C(1), 165.15 C(3), 115.18 C(2), 59.31 C(9), 40.09 C(5), 30.82 C(6), 26.21 C(7), 26.11 C(8), 20.86 C(4), 14.27 C(10); IR (neat): 2979m, 2926s, 2853m, 1717s, 1636m, 1580w, 1559w, 1539w, 1507w, 1447m, 1379m, 1352w, 1348w, 1300w, 1270w, 1250m, 1210s, 1150s, 1096w, 1049m, 1030w, 955w, 893w, 855m; MS (70 eV): 196 (M+, 83), 181 (19), 151 (58), 150 (15), 149 (17), 135 (23), 123 (40), 122 (33), 121 (37), 115 (11), 111 (13), 109 (29), 108 (66), 107 (27), 102 (11), 95 (32), 93 (36), 91 (15), 87 (23), 82 (15), 81 (67), 80 (13) 79 (50), 77 (18), 69 (42), 68 (11), 67 (100), 65 (11), 55(50), 54 (13), 53 (24), 43 (26), 41 (86), 39 (35); Anal. Calcd for C12H20O2: C, 73.43; H, 10.27. Found: C, 73.41; H, 10.29; TLC: Rf 0.40 (2% Et2O in pentane); GC: tR: 19.98 min (HP-5; 70 ˚C (5 min); 10 ˚C/min; 200 ˚C (7 min)).
Preparation of (E)-3-Cyclohexyl-2-buten-1-ol ((E)-7). A dry, 25-mL, three-necked, round-bottomed flask fitted with an addition funnel, stirring bar and thermometer was charged with diisobutylaluminum hydride (1M in CH2Cl2, 5.25 mL, 5.25 mmol) and was cooled to -62 ˚C. A solution of ethyl (E)-3-cyclohexyl-2-butenoate (E)-6 (E/Z: 97/3, 0.490 g, 2.5 mmol) in dry CH2Cl2 (5 mL) was added at -62 ˚C to the DIBAL solution over 18 min. After 40 min, the reaction was quenched with 1 N HCl (50 mL; T = -20 ˚C to +10 ˚C) and diluted with CH2Cl2 (50 mL). The clear layers were separated and the aqueous layer extracted with CH2Cl2 (2x25 mL). The organic extracts were combined, dried (Na2SO4), concentrated in vacuo and the residue was distilled to afford 0.360 g (94%) of alcohol (E)-7. A sample was purified by column chromatography on silica gel (hexane/EtOAc, 4:1) and redistilled to provide analytically pure (E)-7. Analytical data from (E)-7: bp 75 - 80 ˚C (0.4 mm Hg, air bath temperature); 1H NMR (300 MHz, CDCl3): 5.37 (t, J = 6.8, 1 H, HC(2)), 4.15 (m, 2 H, H2C(1)), 1.87-1.57 (m, 6H, HaxC(5), 5HeqC6H11), 1.63 (s, 3 H, H3C( 4)), 1.31-1.09 (m, 5 H, 5HaxC6H11); 13C NMR (75.5 MHz, CDCl3): 144.67 C(3), 121.50 C(2), 59.37 C(1), 47.12 C(5), 31.70 C(6), 26.64 C(7), 26.31 C(8), 14.62 C(4); IR (neat): 3339br,m, 2924s, 2851s, 1663w, 1449m, 1379w, 1237w, 1184w, 1080w, 999m, 891w, 858w, 808w, 764w; MS (70 eV): 154 (M+, 7), 123 (24), 110 (24), 95 (10), 83 (11), 82 (14), 81 (70), 79 (16), 72 (15), 71 (100), 69 (13), 68 (11), 67 (46), 55 (53), 53 (14), 43 (13), 41 (55); Anal. Calcd for C10H18O: C, 77.87; H, 11.76. Found: C, 77.85; H, 11.79; TLC: Rf 0.20 (hexane/EtOAc, 4:1); GC: tR: 17.87 min (HP-1: 75 ˚C (5 min), 10 ˚C/min, 200 ˚C (15 min)).
Preparation of (Z)-3-Cyclohexyl-2-buten-1-ol ((Z)-7). A dry, 25-mL, three-necked, round-bottomed flask fitted with an addition funnel, stirring bar and thermometer was charged with diisobutylaluminum hydride (1.0 M, 7 mL, 7 mmol, 2.25 equiv) and the solution was cooled to -60 ˚C. A solution of ethyl (Z)-3-cyclohexyl-2-butenoate (Z)-6 (0.610 g, 3.10 mmol) in dry CH2Cl2 (6 mL) was added at -68 ˚C over 20 min to the DIBAL solution. . The mixture was stirred at -68 ˚C for 1.4 h and quenched by the careful addition of 1 N HCl (90 mL) and diluted with CH2Cl2 (50 mL). The clear layers were separated and the aqueous layer was extracted with CH2Cl2 (100 mL). The organic layers were dried (Na2SO4), filtered and concentrated in vacuo. The residue was purified by column chromatography on silica gel with hexane/EtOAc, 4:1 as eluent. Distillation of the chromatographically pure material afforded 0.450 g (94%) of alcohol (Z)-7 as a colorless oil. Analytical data from (Z)-7: bp 80 ˚C (0.3 mm Hg, air bath temperature); 1H NMR (300 MHz, CDCl3): 5.33 (dt, J = 7.0,1.0,1 H, HC(2)), 4.15 (d, J = 7.0, 2 H, H2C(1)), 2.43 (m, 1 H, HC(5)), 1.66 (s, 3 H, H3C( 4)), 1.80-1.12 (m, 10 H, 2H2C(6), 2H2C(7), H2C(8)); 13C NMR (75.5 MHz, CDCl3): 144.97 C(3), 123.09 C(2), 58.38 C(1), 39.87 C(5), 31.24 C(6), 26.40 C(7), 26.04 C(8), 19.63 C(4); IR (neat): 3343m,br, 2960m, 2926s, 2853s, 1657w, 1449s, 1377m, 1320w, 1255w, 1250w, 1240w, 1183w, 1140w, 1090w, 1019s, 992m, 891m, 861w, 764w; MS (70 eV): 154 (M+, 1), 136 (31), 123 (25), 121 (18), 110 (16), 107 (17), 95 (13), 93 (16), 83 (12), 82 (17), 81 (85), 79 (27), 77 (10), 72 (14), 71 (100), 69 (16), 68 (17), 67 (61), 55 (66), 54 (12), 53 (19), 43 (16), 41 (70); Anal. Calcd for C10H18O: C, 77.87; H, 11.76. Found: C, 77.66; H, 11.70; TLC: Rf 0.25 (hexane/EtOAc, 4:1); GC: tR: 17.35 min (HP-5; 75 ˚C (5 min), 10 ˚C/min, 200 ˚C (15 min)).
Preparation of (E)-3-Methyl-2-hepten-1-ol (10). A dry, 250-mL, four-necked, round-bottomed flask fitted with an addition funnel, thermometer, stirring bar and gas control valve was charged with a solution of diisobutylaluminum hydride (1 M, 30 mL, 30 mmol, 2.26 equiv). The flask was cooled to -65 ˚C and a solution of ethyl (E)-3-methyl-2-heptenoate (2.27 g, 13.3 mmol) in dry CH2Cl2 (25 mL) was added to the DIBAL solution at -65 ˚C over 50 min. The reaction was quenched after 1.1 h by the slow addition of 1 N HCl at -40 ˚C to 0 ˚C. The quenched reaction mixture was diluted with CH2Cl2 (40 mL) with a total of 130 mL 1 N HCl was required to completely digest the aluminum salts. The layers were separated and the aqueous layer was extracted with CH2Cl2 (2x50 mL). The combined organic layers were dried (Na2SO4), filtered and concentrated in vacuo. The residue was purified by column chromatography on silica gel with hexane/EtOAc, 4:1 as eluent to afford 99% (1.71 g) of 10. The alcohol obtained was distilled to afford analytically pure 10 as a colorless oil in a 97/3 E/Z ratio. Analytical data from 10: bp 65 ˚C (0.4 mm Hg, air bath temperature); 1H NMR (360 MHz, CDCl3): 5.41 (t, J = 6.8, 1.0, 1 H, HC(2)), 4.,15 (d, J = 6.6, 2 H, H2C(1)), 2.02 (2d, J = 7.6, 7.0, 2 H, H2C(4)), 1.67 (s, 3 H, H3C( 8)), 1.40-1.25 (m, 4 H, H2C(5), H2C(6)), 0.90 (t, J = 7.0, 3 H, H3C( 7)); 13C NMR (75.5 MHz, CDCl3): 140.03 C(3), 123.03 C(2), 59.29 C(1), 39.19 C(4), 29.81 C(5), 22.32 C(6), 16.09 C(8), 13.93 C(7); IR (neat): 3339br,s, 2957s, 2930s, 2875s, 2861s, 1669w, 1456m, 1379m, 1239w, 1205w, 1074w, 999s, 875w; MS (70 eV): 128 (M+, 2), 71 (100), 68 (15), 57 (11), 56 (10), 55 (20), 43 (20), 41 (35); Anal. Calcd for C8H16O: C, 74.94; H, 12.58. Found: C, 74.95; H, 12.57; TLC: Rf 0.20 (hexane/EtOAc, 4:1); GC: tR: 13.43 min, 97/3, E/Z (HP-1; 75 ˚C (5 min), 10 ˚C/min, 200 ˚C (15 min)).
Preparation of (E)-3,4,4-Trimethyl-2-penten-1-ol (11). A 500-mL, three-necked, round-bottomed flask equipped with an addition funnel, thermometer, stir bar and gas control valve was charged with a solution of diisobutylaluminum hydride (1 M, 100 mL, 100 mmol, 2.15 equiv). A solution of ethyl (E)-3,4,4-trimethyl-2-pentenoate (7.92 g, 46.5 mmol) in dry CH2Cl2 (8 mL) was added to the cooled DIBAL solution at -70 ˚C over 1.5 h. The reaction was quenched 1.5 h after complete addition by dilution with CH2Cl2 (100 mL) and slow addition of 1 N HCl (100 mL). Then, the mixture was transferred to a 1 L beaker and 1 N HCl was added until the aqueous layer was clear (400 mL acid total). After dilution with CH2Cl2 (50 mL) the layers were separated and the aqueous layer was extracted with CH2Cl2 (2x300 mL). The combined organic layers were dried (Na2SO4), concentrated in vacuo and the residue was distilled to give 5.95 g (99%) of alcohol 11 as a clear oil. Analytical data from 11: bp 130 ˚C (20 mm Hg, air bath temperature); 1H NMR (300 MHz, CDCl3): 5.45 (t, J = 6.5, 1 H, HC(2)), 4.18 (d, J = 6.5, 2 H, H2C(1)), 1.66 (s, 3 H, H3C( 6)), 1.05 (s, 9 H, H3C( 5,7,8)); 13C NMR (75.5 MHz, CDCl3): 147.08 C(3), 120.34 C(2), 59.98 C(1), 36.11 C(4), 28.81 C(5,7,8), 12.81 C(6); IR (neat): 3339s,br, 3056w, 2965s, 2915s, 2870s, 1653w, 1466m, 1380m, 1362m, 1302w, 1262w, 1217w, 1140w, 1082w, 1028s, 1001s, 853w, 803w; MS (70 eV): 97 (46), 95 (36), 72 (25), 71 (75), 70 (13), 69 (83), 67 (20), 57 (73), 56 (11), 55(62), 53 (17), 43 (51), 41 (100), 39 (37); Anal. Calcd for C8H16O: C, 74.94; H, 12.58. Found: C, 74.95; H, 12.56; TLC: Rf 0.20 (hexane/EtOAc, 4:1); GC: tR: 11.81 min (HP-5; 75 ˚C (5 min), 10 ˚C/min, 200 ˚C (10 min)).
Preparation of (E)-(S)-3-Cyclohexyl-2-butenyl N-[2-Methoxy-1-(1-naphthyl)ethyl] Carbamate ((E)-(S)-1). A solution of 3-cyclohexyl-2-buten-1-ol (E)-7 (E/Z 97/3; 0.300 g, 1.9 mmol), (S)-(-)-2-methoxy-1-(1-naphthyl)ethyl isocyanate (S)-8 (0.460 g, 2.0 mmol, 94% ee), N,N-diethylethanolamine (5 mg, catalyst) and dry toluene (10 mL) was placed in a dry, 25-mL, three-necked, round-bottomed flask fitted with a reflux condenser and thermometer. The solution was heated at reflux for 47 h. The solution was cooled, concentrated in vacuo and the residue was purified by column chromatographed on silica gel with 1/2% MeOH in CHCl3 to afford 0.640 g (88%) of carbamate (E)-(S)-1 as a waxy solid. It was recrystallized from Et2O/pentane, 8:1 to afford analytically pure material. Analytical data from (E)-(S)-1: mp 80 - 82 ˚C (Et2O/pentane, 8:1); Optical Rotation: [α]D24 -13.65 (c 1.0, CHCl3), 96.0:2.0 er; 1H NMR (300 MHz, CDCl3): 8.11 (d, J = 9, 1 H, HC(10)), 7.87 (d, J = 9, 1 H, HC(4)), 7.78 (d, J = 9, 1 H, HC(7)), 7.56-7.42 (m, 4 H, HC(5,6,8,9)), 5.73 (brs, 1 H, HC(1)), 5.49 (brs, 1 H, HC(14)), 5.31 (brs, 1 H, HR2), 4.59 (brs, 2 H, H2C(13)), 3.82 (dd, J = 9, 3, 1 H, HC(2)), 3.70 (brs, 1 H, HC(2)), 3.35 (s, 3 H, H3C(11)), 1.85-1.50 (m, 6H, HeqC6H11, HC(17)), 1.65 (brs, 3 H, H3C(16)), 1.30-1.10 (m, 5 H, HaxC6H11); 13C NMR (75.5 MHz, CDCl3): 156.18 C(12), 146.96 C(15), 135.51 C(3), 133.89 C(10a), 130.85 C(6a), 129.95 C(4), 128.18 C(7), 126.35 C(6), 125.60 C(8), 125.28 C(9), 123.59 C(5), 122.74 C(10), 116.84 C(14), 74.58 C(2), 62.11 C(13), 59.04 C(11), 50.56 C(1), 47.13 C(17), 31.61 C(18), 26.61 C(19), 26.31 C(20), 14.84 C(16); IR (KBr): 3295m, 3038w, 2980w, 2960w, 2930m, 2920m, 2851m, 1707s, 1661m, 1600w, 1545s, 1510w, 1478m, 1443m, 1383m, 1358w, 1337m, 1285m, 1262s, 1233s, 1190m, 1149w, 1109s, 1084s, 1076s, 1038s, 994m, 957m, 945m, 909w, 890w, 860w, 855w, 830w, 801s, 777s; MS (70 eV): 381 (M+, 3), 336 (13), 292 (28), 200 (16), 157 (12), 156 (97), 154 (18), 153 (20), 137 (45), 127 (10), 95 (51), 81 (100), 69 (23), 67 (26), 55 (70), 45 (13), 41 (28); MS (10 eV): 381 (M+, 20), 337 (33), 336 (100), 292 (49), 200 (35), 156 (78), 137 (29); Anal. Calcd for C24H31NO3: C, 75.56; H, 8.19; N, 3.67. Found: C, 75.50; H, 8.17; N, 3.66; TLC: Rf 0.50 (1/2% MeOH in CHCl3).
Preparation of (Z)-(R)-3-Cyclohexyl-2-butenyl N-(2-Methoxy-1-(1-naphthyl)ethyl) Carbamate ((Z)-(R)-1). To a dry, 15-mL, three-necked, round-bottomed flask fitted with a thermometer and reflux condenser was added (R)-2-methoxy-1-(1-naphthyl)ethyl isocyanate (R)-8 (0.669 g, 2.94 mmol, 98.2% ee), (Z)-3-cyclohexyl-2-buten-1-ol (Z)-7 (0.442 g, 2.87 mmol), N,N-diethylethanolamine (10 mg, catalyst) and dry toluene (3 mL). The solution was heated at 110 ˚C for 18.5 h. The solution was cooled and concentrated in vacuo and the residue purified by column chromatography on silica gel with hexane/EtOAc, 5:1 to yield 1.00 g (92%) of carbamate (Z)-(R)-1 as a viscous yellow oil. The oil solidified after having been dried under vacuum (0.05 mm Hg) overnight. Analytical data from (Z)-(R)-1: mp 67 - 72 ˚C; Optical Rotation: [α]D24 +8.68 (c 1.45, CHCl3), 99.1:0.9 er; 1H NMR (300 MHz, CDCl3): 8.11 (d, J = 8, 1 H, HC(10)), 7.85 (d, J = 8, 1 H, HC(4)), 7.76 (d, J = 8, 1 H, HC(7)), 7.55-7.39 (m, 4 H, HC(5,6,8,9)), 5.74 (m, 1 H, HC(1)), 5.55 (m, 1 H, HC(2')), 5.26 (m, 1 H, HR2), 4.60 (d, J = 7.4, 2 H, H2C(1')), 3.80 (dd, J = 9.6, 4.4, 1 H, HC(2)), 3.69 (m, 1 H, HC(2)), 3.32 (s, 3 H, H3C(11)), 2.42 (m, 1 H, HC(5')), 1.87-1.13 (m, 13 H, 10H of C6H11 and H3C( 4')); 13C NMR (75.5 MHz, CDCl3): 156.08 (C=O), 146.99 C(3'), 135.40 C(3), 133.81 C(10a), 130.76 C(6a), 128.86 C(4), 128.09 C(7), 126.26 C(6), 125.51 C(8), 125.19 C(9), 123.50 C(5), 122.65 C(10), 118.32 C(2'), 74.51 C(2), 61.10 C(1'), 58.92 C(11), 50.48 C(1), 39.90 C(5'), 31.02 C(6'), 26.27 C(7'), 26.00 C(8'), 19.62 C(4'); MS (70 eV): 381 (M+), 292 (21), 200 (15), 182 (15), 157 (12), 156 (94), 154 (18), 153 (18), 137 (43), 127 (11), 95 (53), 81 (100), 69 (23), 67 (23), 55 (64), 45 (12), 41 (26); Anal. Calcd for C24H31NO3: C, 75.56; H, 8.19; N, 3.67. Found: C, 75.60; H, 8.19; N, 3.67; TLC: Rf 0.30 (hexane/EtOAc, 5:1).
Preparation of (E)-(R)-3-Methyl-2-heptenyl N-[2-Methoxy-1-(1-naphthyl)ethyl] Carbamate ((E)-(R)-2). A dry, 15-mL, three-necked, round-bottomed flask fitted with a thermometer and reflux condenser was charged with (E)-3-methyl-2-hepten-1-ol 10 (0.930 g, 4.09 mmol), isocyanate (R)-8 (0.930 g, 4.09 mmol, 98.2% ee), and N,N-diethylethanolamine (10 mg) and dry toluene (4 mL). The solution was heated at 110 ˚C for 30.5 h and then cooled. The solution was concentrated in vacuo and the slightly yellow residue was purified by column chromatography on silica gel with hexane/EtOAc, 5:1 to afford 1.38 g (97%) of carbamate (E)-(R)-133 which was analytically pure. Analytical data from (E)-(R)-2: mp softens 46 - 50 ˚C; melts 50 – 53 ˚C; Optical Rotation: [α]D24 +12.3 (c 1.0, CHCl3), 99.1:0.8 er; 1H NMR (300 MHz, CDCl3): 8.11 (d, J = 8.1, 1 H, HC(10)), 7.86 (d, J = 7.6, 1 H, HC(4)), 7.76 (d, J = 8.0, 1 H, HC(7)), 7.55-7.40 (m, 4 H, HC(5,6,8,9)), 5.74 (m, 1 H, HR2), 5.53 (m, 1 H, HC(2’)), 5.32 (m, 1 H, HC(1)), 4.57 (m, 2 H, H2C(1’)), 3.80 (dd, J = 9.5, 4.4, 1 H, HC(2)), 3.70 (m, 1 H, HC(2)), 3.33 (s, 3 H, H3C(11)), 2.00 (m, 2 H, H2C(4’)), 1.66 (m, 3 H, H3C( 8’)), 1.36-1.26 (m, 4 H, H2C(5’), H2C(6’)), 0.88 (t, J = 7.0, 3 H, H3C( 7’)); 13C NMR (75.5 MHz, CDCl3): 156.10 (C=O), 142.33 C(3’), 135.39 C(3), 133.82 C(10a), 130.77 C(6a), 128.89 C(4), 128.11 C(7), 126.28 C(5), 125.54 C(8), 125.21 C(9), 123.52 C(6), 122.66 C(10), 118.38 C(2’), 74.51 C(2), 61.90 C(1’), 58.96 C(11), 50.48 C(1), 39.16 C(4’), 29.69 C(5’), 22.28 C(6’), 16.28 C(8’), 13.94 C(7’); IR (CCl4): 3449w, 3052w, 2959m, 2930m, 2874w, 2835w, 1725s, 1598w, 1499s, 1381w, 1289w, 1250w, 1217s, 1121m, 1088w, 1042w, 968w; MS (70 eV): 355 (M+, 1), 266 (10), 200 (17), 182 (11), 156 (71), 154 (14), 153 (15), 111 (33), 69 (100), 55 (60), 41 (22); Anal. Calcd for C22H29NO3: C, 74.33; H, 8.22; N, 3.94. Found: C, 74.38; H, 8.24; N, 3.87; TLC: Rf 0.225 (hexane/EtOAc, 5:1).
Preparation of (E)-(R)-3,4,4-Trimethyl-2-pentenyl N-[2-Methoxy-1-(1-naphthyl)ethyl] Carbamate ((E)-(R)-3). A dry, 15-mL, three-necked, round-bottomed flask fitted with a thermometer and reflux condenser was charged with (E)-3,4,4-trimethyl-2-penten-1-ol 11 (0.500 g, 3.90 mmol), isocyanate (R)-8 (0.90 g, 3.96 mmol, 99.1:0.9 er), N,N-diethylethanolamine (10 mg), and dry toluene (4.5 mL). The solution was heated at 100 ˚C for 29 h. The reaction solution was cooled, concentrated in vacuo and the residue was purified by column chromatography on silica gel with hexane/EtOAc, 5:1 to afford 1.25 g (90%) of (E)-(R)-134 as a white powder. Analytical data from (E)-(R)-3: M. W.: 355.48; mp 70 - 85 ˚C; Optical Rotation: [α]D24 +11.42 (c 1.08, CHCl3), 99.1:0.9 er; 1H NMR (300 MHz, CDCl3): 8.11 (d, J = 8.1, 1 H, HC(10)), 7.86 (d, J = 7.8, HC(4)), 7.77 (d, J = 8.0, HC(7)), 7.56-7.40 (m, 4 H, HC(5,6,8,9)), 5.74 (m, 1 H, HC(1)), 5.55 (m, 1 H, HC(2’)), 5.37 (m, 1 H, HR2), 4.61 (m, 2 H, H2C(1’)), 3.81 (dd, J = 9.2, 4.1, 1 H, HC(2)), 3.69 (m, 1 H, HC(2)), 3.34 (s, 3 H, H3C(11)), 1.66 (br s, 3 H, H3C( 8’)), 1.21 (m, 9 H, H3C( 5’,6’,7’)); 13C NMR (75.5 MHz, CDCl3): 156.12 (C=O), 148.98 C(3’), 135.39 C(3), 133.82 C(10a), 130.78 C(6a), 128.89 C(4), 128.12 C(7), 126.29 C(6), 125.55 C(8), 125.21 C(9), 123.51 C(5), 122.67 C(10), 115.77 C(2’), 74.49 C(2), 62.58 C(1’), 58.97 C(11), 50.48 C(1), 36.22 C(4’), 28.71 C(5’,6’,7’), 12.95 C(8’); IR (CCl4): 3451w, 3170w, 2967m, 2920w, 2880w, 2820w, 1725s, 1600w, 1499s, 1379w, 1289w, 1256w, 1217m, 1121m, 1046w; MS (70 eV): 355 (M+, 1), 310 (10), 266 (14), 200 (16), 157 (10), 156 (77), 154 (16), 153 (20), 111 (85), 69 (100), 59 (10), 57 (21), 56 (17), 55 (51), 45 (19), 43 (20), 41 (36); Anal. Calcd for C22H29NO3: C, 74.33; H, 8.22; N, 3.94. Found: C, 74.41; H, 8.23; N, 3.90; TLC: Rf 0.25 (hexane/EtOAc, 5:1).
Preparation of (E)-(S)-3-Phenyl-2-propenyl N-[2-Methoxy-1-(1-naphthyl)-ethyl] Carbamate ((E)-(S)-4). A dry, 15-mL, three-necked, round-bottomed flask fitted with a thermometer and reflux condenser was charged with (E)-cinnamyl 4-nitrophenyl carbonate (0.610 g, 2.04 mmol), amino ether (S)-1210 (0.436 g, 2.17 mmol, 99.6:0.4 er), N,N-diethylethanolamine (10 mg), and dry toluene (7 mL). The solution was heated at 110 ˚C for 30 h and then cooled. The dark yellow solution was diluted with benzene (10 mL) and washed with 1 M NaOH (10 mL). The benzene layer was washed with NaCl (2x10 mL) and the aqueous layers were extracted with benzene (2x20 mL). The combined organic layers were dried (Na2SO4), concentrated in vacuo and the residue was purified by column chromatography on silica gel with hexane/EtOAc, 4:1. The slightly yellow solid was recrystallized from Et2O/THF 6:1 to afford analytically pure (E)-(S)-4 in 70% yield (0.516 g). Analytical data from (E)-(S)-4: mp 115 - 117 ˚C (Et2O/THF, 6:1); Optical Rotation: [α]D24 -31.88° (c 1.05, CHCl3), 99.6:0.4 er; 1H NMR (300 MHz, CDCl3): 8.12 (d, J = 8, 1 H, HC(10)), 7.86 (d, J = 8, 1 H, HC(4)), 7.78 (d, J = 8, 1 H, HC(7)), 7.55-7.23 (m, 9 H, H5C6, HC(5,6,8,9)), 6.62 (m, 1 H, HC(3')), 6.27 (m, 1 H, HC(2')), 5.76 (m, 1 H, HC(1)), 5.61 (m, 1 H, HR2), 4.71 (m, 2 H, H2C(1')), 3.80 (dd, J = 9, 4, 1 H, HC(2)), 3.71 (m, 1 H, HC(2)), 3.35 (s, 3 H, H3C(11)); 13C NMR (75.5 MHz, CDCl3): 155.77 (C=O), 136.27 C(4'), 135.30 C(3), 133.86 C(3'), 133.59 C(6a), 130.78 C(10a), 128.95 C(4), 128.49 C(6'), 128.22 C(7), 127.86 C(7'), 126.53 C(5'), 126.40 C(9), 125.61 C(5), 125.26 C(8), 123.75 (C2'), 123.56 C(6), 122.63 C(10), 74.50 C(2), 65.55 C(1'), 59.01 C(11), 50.59 C(1); IR (KBr): 3303m, 3078w, 3050w, 3025w, 2985w, 2930w, 2886w, 2865w, 2805w, 1686s, 1549s, 1505w, 1500w, 1465s, 1455s, 1450s, 1390w, 1383w, 1355w, 1335m, 1266s, 1195w, 1180w, 1150w, 1121s, 1098m, 1080m, 1048s, 967s, 915w, 797m, 774s, 750m, 735w, 693m; MS (70 eV): 361 (M+, 1), 272 (13), 118 (11), 117 (100), 115 (13); Anal. Calcd for C23H23NO3: C, 76.43; H, 6.41; N, 3.88. Found: C, 76.40; H:, 6.37; N, 3.85; TLC: Rf 0.25 (hexane/EtOAc, 4:1).
Preparation of (E)-(R)-3-Phenyl-2-butenyl N-[2-Methoxy-1-(1-naphthyl)ethyl] Carbamate ((E)-(R)-5). A dry, 15-mL, three-necked, round-bottomed flask fitted with a thermometer and reflux condenser was charged with (E)-3-phenyl-2-buten-1-ol 13 (0.424 g, 2.86 mmol), isosyanate (R)-8 (0.650 g, 2.86 mmol, 99.1:0.9 er), N,N-diethylethanolamine (10 mg), and dry toluene (3 mL). The solution was heated at 110 ˚C for 29 h and then cooled. The cooled mixture was concentrated in vacuo and the residue was purified by column chromatography on silica gel twice with hexane/EtOAc, 5:1 to afford 1.0 g (93%) of carbamate (E)-(R)-5 as a waxy white solid. Analytical data from (E)-(R)-5: mp 77-78 ˚C; Optical rotation: [α]D24 +23.0 (c 1.07, CHCl3) 99.1:0.9 er; 1H NMR (300 MHz, CDCl3): 8.12 (d, J = 7.8, 1 H, HC(10)), 7.86 (d, J = 7.7, 1 H, HC(4)), 7.77 (d, J = 8.0, 1 H, HC(7)), 7.53-7.20 (m, 9 H, 5PhH, HC(5,6,8,9)), 5.89 (m, 1 H, HC(1)), 5.76 (m, 1 H, HC(2’)), 5.58 (brd, J = 6.1, HR2), 4.78 (m, 2 H, H2C(1’)), 3.80 (m, 1 H, HC(2)), 3.70 (m, 1 H, HC(2)), 3.34 (s, 3 H, H3C(11)), 2.08 (s, 3 H, H3C( 8’)); 13C NMR (75.5 MHz, CDCl3): 155.98 (C=O), 142.55 C(3’), 139.71 C(4’), 135.50 C(3), 133.84 C(6a), 130.77 C(10a), 128.92 C(4), 128.17 C(6’,7), 127.35 C(7’), 126.34 C(9), 125.78 C(5’), 125.58 C(5), 124.24 C(8), 123.54 C(6), 122.64 C(10), 121.84 C(2’), 74.49 C(2), 62.19 C(1’), 58.99 C(11), 50.59 C(1), 16.12 C(8’); IR (CCl4): 3447w, 3056w, 2990w, 2926w, 2895w, 2820w, 1727s, 1595w, 1497s, 1383w, 1340w, 1300w, 1289w, 1250w, 1217m, 1121m, 1095w, 1060w, 1044m; MS (70 eV): 375 (M+,10), 330 (26), 287 (15), 286 (51), 182 (46), 157 (11), 156 (61), 155 (13), 154 (29), 153 (25), 141 (12) 133 (12), 132 (42), 131 (100), 130 (13), 129 (27), 128 (16), 127 (25), 116 (21), 115 (26), 105 (11), 91 (52), 77 (13); Anal. Calcd for C24H25NO3: C, 76.77; H, 6.71; N, 3.73. Found: C, 76.77; H, 6.76; N, 3.71; TLC: Rf 0.18 (hexane/EtOAc, 5:1).
Cuprate Reaction of (E)-(S)-3-Cyclohexyl-2-butenyl N-[2-Methoxy-1-(1-naphthyl)ethyl] carbamate (E)-(S)-1. Phenyl as Tranferrable Ligand, Use of Two Equivalents Phenylcopper. Preparation of (R)-3-Cyclohexyl-3-phenyl-1-butene ((R)-14). Two three-necked, round-bottomed flasks (15 and 25 mL) containing stirring bars were oven-dried, then evacuated and filled with dry nitrogen twice while hot. When the flasks had cooled, copper(I) iodide (0.919 g, 4.83 mmol, 2.05 equiv) was placed in the 25-mL flask and then a septum and a thermometer (small bulb) were fitted in the outer necks. The flask was evacuated and filled twice with dry nitrogen while stirring the powder. To the 15-mL flask was added (E)-(S)-1 (0.864 g, 2.40 mmol) and 10 mg of 2,2'-dipyridyl (end point indicator). The flask was then equipped with a septum and thermometer in the same way as the 25-mL flask and was evacuated and filled twice with dry nitrogen. Dry Et2O (5 mL) was added to both flasks and the suspension of CuI was cooled to 0 °C. The carbamate slurry was stirred at room temperature until cooled for anion formation. This treatment provided time for the large chunks of carbamate to be evenly suspended in the ether for ease of deprotonation. Any large pieces of carbamate left in the anion solution typically did not dissolve and therefore changed the exact amount of carbamate used for the reaction as the undissolved carbamate could not be transferred. Phenyllithium (2.74 M, 1.75 mL, 4.80 mmol, 2.04 equiv) was added dropwise at -5 °C over 3 min to give a brown-black slurry. The mixture was stirred for 20 min at -5 °C to 0 °C) with one brief removal of the cooling bath and movement of the stirring bar to the sides of the flask to mix all the copper(I) iodide into the Et2O. Then, n-butyllithium (1.60 M, 1.50 mL, 2.40 mmol, 1.02 equiv) was added dropwise slowly over 10 min to the cooled (0 °C) carbamate mixture, until a persistent purple color was observed. The temperature was allowed to rise to 10 °C briefly halfway through the addition to dissolve the unreacted carbamate. The anion solution was transferred to the phenylcopper slurry via Teflon cannula (normal addition) and the 15-mL flask was rinsed with dry Et2O (1 mL) and this was also transferred via Teflon cannula to the reaction vessel giving a brown slurry with a final cuprate concentration of 0.154 M. The mixture was stirred for 13 min at 0 °C and the cooling bath was removed. The root beer brown slurry slowly became black over 22 h. The reaction was quenched by addition of saturated neutral ammonium chloride and dilution with Et2O. The black slurry was transferred to a 250-mL Erlenmeyer flask after scraping the sides of the reaction vessel to loosen all the solid. A total of 50 mL of aqueous ammonium chloride and 100 mL of Et2O were used to quench the reaction and thoroughly rinse the contents of the reaction vessel into the Erlenmeyer flask. The biphase was stirred 0.5 h and the layers were separated. A fresh aliquot of ammonium chloride (50 mL) was added to the ether layer which still contained much undissolved solid and the biphase was stirred 0.5 h again. The layers were separated and the ammonium chloride wash was repeated a third time. This treatment was sufficient to dissolve most of the solid that formed during quench of the reaction. The aqueous layers were extracted separately with Et2O (2x100 mL) and the ether layers were washed with the individual aqueous ammonium chloride aliquots. The organic layers were dried (Na2SO4), concentrated and the residue was chromatographed as follows: the column was loaded and one column volume eluted with pentane (this elutes the alkene and separates the biphenyl; use of any ether caused coelution of the alkene and biphenyl). The eluent was changed to hexane/EtOAc, 4/1 for approximately one column volume to elute the unreacted carbamate. Finally, the eluent was changed to 12% MeOH in CHCl3 to elute the amino ether chiral auxiliary. The yields of (R)-14 were 67% and 87% with 99:1 and >99.9:0.1 er, respectively for two reactions. An analytical sample of (R)-14 was obtained after column chromatography on silica gel with pentane and distillation of the isolated alkene. Analytical data from (R)-14: bp 105 ˚C (1 mm Hg, air bath temperature); Optical rotation: [α]D24 +35.72 (c 1.45, CHCl3), >99.9:0.1 er; 1H NMR (300 MHz, CDCl3): 7.33 (m, 4 H, 2HC(6), 2HC(7)), 7.18 (m, 1 H, HC(8)), 6.20 (dd, J = 15, 3, 1 H, HC(2)), 5.16 (d, J = 12, 1 H, HZC(1)), 5.04 (d, J = 18, 1 H, HEC(1)), 1.79-1.63 (m, 4 H, HaxC(9), 2HeqC(11), HeqC(12)), 1.48 (m, 2 H, 2HeqC(10)), 1.35 (s, 3 H, H3C(13)), 1.24-0.90 (m, 5 H, 2HaxC(10b), 2HaxC(11), HaxC(12)); 13C NMR (75.5 MHz, CDCl3): 148.12 C(5), 145.27 C(2), 127.93 C(6), 126.59 C(7), 125.42 C(8), 112.74 C(1), 47.26 C(9), 47.23 C(3), 28.14 C(10), 27.78 C(10), 27.16 C(12), 27.07 C(11), 26.70 C(11), 20.75 C(13); IR (neat): 3083w, 3058w, 3025w, 2979m, 2926s, 2851s, 1634w, 1599w, 1493m, 1445m, 1412w, 1372w, 1060w, 1030w, 1015w, 1010w, 912s, 760m, 729w, 698s; MS (70 eV): 214 (M+, 13), 132 (43), 131 (100), 130 (12), 129 (10), 118 (29), 117 (19), 116 (11), 115 (15), 91 (25), 55 (19), 41 (14); Anal. Calcd for C16H22: C, 89.65; H, 10.35. Found: C, 89.62; H, 10.38; TLC: Rf 0.60 (pentane); GC: tR: 18.37 min (HP-1; 75 ˚C (5 min), 10 ˚C/min, 200 ˚C (15 min)).
Cuprate Reaction of (E)-(R)-3-Methyl-2-heptenyl N-[2-Methoxy-1-(1-naphthyl)ethyl] carbamate (E)-(R)-2; Phenyl as Transferable Ligand; Use of Two Equivalents Phenylcopper. Preparation of (R)-3-Methyl-3-phenyl-1-heptene ((R)-16). Carbamate (E)-(R)-2 was allowed to react with 2 equivalents phenylcopper under standard normal addition conditions as described for (E)-(S)-1 to give 66-74% yields of alkene (R)-16 with 88.5:11.5 to 85.0:15.0 er, respectively for two reactions (see Table 3 for summary of results). An analytical sample of (R)-16 was obtained after column chromatography on silica gel with pentane and distillation of the isolated alkene. Analytical data from (R)-16: bp 65 ˚C (0.1 mm Hg, air bath temperature); Optical rotation: [α]D24 -10.9 (c 1.05, CHCl3), 88.5:11.5 er; 1H NMR (300 MHz, CDCl3): 7.37 (m, 4 H, HC(9), 2HC(10), HC(11)), 7.24 (m, 1 H, HC(9)), 6.08 (dd, J = 17.5, 10.8, 1 H, HC(2)), 5.14 (dd, J = 11.3, 1.0, 1 H, HZC(1)), 5.09 (dd, J = 18.3, 1.0, 1 H, HEC(1)), 1.78 (m, 2 H, H2C(4)), 1.39 (s, 3 H, HC(12)), 1.29 (2 quintets, J = 7.1, H2C(6)), 1.40-1.00 (m, 2 H, H2C(5), 0.91 (t, J = 7.1 , 3 H, H3C( 7)); 13C NMR (75.5 MHz, CDCl3): 147.70 C(8), 147.16, C(2), 127.99 C(10), 126.57 C(9), 125.62 C(11), 111.50 C(1), 44.21 C(3), 40.87 C(4), 26.68 (5), 24.94 C(12), 23.39 C(6), 14.06 C(7); IR (neat): 3085m, 3058m, 3027m, 3000m, 2959s, 2932s, 2863s, 1634w, 1601w, 1495m, 1458m, 1447m, 1412w, 1374w, 1109w, 1073w, 1030w, 1000w, 912s, 764s; MS (70 eV): 188 (M+, 7), 132 (13), 131 (100), 91 (25); Anal. Calcd for C14H20: C, 89.29; H, 10.71. Found: C, 89.22; H, 10.74; TLC: Rf 0.75 (pentane).
Preparation of Analytes for Enantiomeric Excess Determination.
Preparation of (±)-(2-Cyclohexyl-2-phenyl)-1-propyl N-(3,5-Dinitrophenyl) Carbamate ((±)-23). A solution of 3,5-dinitrobenzoylazide (0.322 g, 1.36 mmol) in dry toluene (8 mL) in a one-necked, 10-mL, round-bottomed flask fitted with a reflux condenser was heated for 10 min at 110 ˚C and then (±)-3-cyclohexyl-3-phenyl-1-propanol (±)-22 (0.220 g, 1.08 mmol) in dry toluene (1 mL) was added. The solution was heated 30 min at 110 ˚C and cooled. The mixture was concentrated in vacuo and the yellow residue was purified by column chromatography on silica gel with hexane/EtOAc, 5:1 to yield a yellow white solid. The solid was recrystallized from hexane/EtOAc to yield 0.430 g (96%) of (±)-23 as a slightly yellow solid. Analytical data from (±)-23: mp 144 - 146 ˚C (Et2O/pentane, 1:1); 1H NMR (300 MHz, CDCl3): 8.6 (t, J = 2.0, 1 H, HC(4)), 8.57 (brs, 2 H, 2HC(2)), 7.32 (m, 4 H, HC(5’,6’,7’,8’)), 7.22 (m, 1 H, HC(9’)), 6.97 (s, 1 H, HR2), 4.62 (d, J = 10.7, 1 H, HC(1’)), 4.43 (d, J = 10.7, 1 H, HC(1’)), 1.75-1.60 (m, 5 H, HeqC6H11), 1.43-0.85 (m, 6 H, HaxC6H11), 1.39 (s, 3 H, H3C( 3’)); 13C NMR (75.5 MHz, CDCl3): 152.88 (C=O), 148.77 C(3), 144.55 C(8), 140.42 C(1), 128.10 C(10), 126.59 C(9), 126.03 C(11), 117.83 C(2), 112.67 C(4), 72.84 C(5), 46.27 C(12), 44.38 C(6), 27.88 C(13), 27.48 C(17), 26.93 C(14), 26.88 C(16), 26.44 C(15), 18.64 C(7); IR (CDCl3): 3422w, 3110w, 3070w, 2988w, 2934w, 2855w, 1744m, 1603w, 1549s, 1528m, 1475w, 1445w, 1424w, 1347m, 1335m, 1270w, 1242m, 1208s, 1075w; MS (70 eV): 209 (19), 187 (28), 135 (18), 119 (10), 118 (66), 117 (40), 106 (11), 105 (100), 91 (31), 83 (18), 77 (10), 57 (11), 55 (33), 43 (19), 41 (21); Anal. Calcd for C22H25N3O6: C, 61.82; H, 5.90; N, 9.83. Found: C, 61.76; H, 5.92; N, 9.82; TLC: Rf 0.375 (hexane/EtOAc, 7:1); HPLC: tR: (R)-23 = 14.03 min; (S)-23 = 18.22 min [column A, (hexane(845):1,2-dichloroethane(50)/t-butanol(5))/i-PrOH : 98/2; 1 mL/min].
Preparation of (R)-2-Methyl-2-phenyl-1-hexanol ((S)-24). A 15-mL, three-necked, round-bottomed flask fitted with a gas dispersion tube, gas exit valve, stirring bar and thermometer was charged with (S)-3-methyl-3-phenyl-1-heptene (R)-16 (0.131 g, 0.722 mmol), CHCl3 (0.36 mL), and MeOH (2.2 mL). The solution was cooled to -42 ˚C while being purged with oxygen. Ozone was then passed through the solution until the faint blue color of excess ozone appeared (10 min). The solution was purged with nitrogen and a solution of NaBH4 (0.385 g, 10.2 mmol) in 50% aqueous EtOH (5 mL) was added dropwise at -30 ˚C to room temperature over 1 h. The resulting mixture was stirred overnight and the reaction was diluted with Et2O (25 mL). A solution of 1N HCl (8 mL) was added dropwise until all the borate salts has dissolved. The layers were separated and the aqueous layer was extracted with Et2O (2x25 mL). The organic layers were combined, dried (Na2SO4), filtered and concentrated in vacuo. The residue was purified by column chromatography on silica gel with hexane/EtOAc, 5:1 to yield a colorless oil. Distillation afforded 0.100 g (72%) of (S)-24 in 72% yield (0.100 g) as a colorless oil in analytically pure form. Analytical data from (S)-24: bp 70 - 80 ˚C (0.05 mm Hg, air bath temperature); Optical Rotation: [α] 0° at all common wavelengths. HPLC analysis of the 3,5-DNP carbamate showed this alcohol to be 85.0:15.0 er; 1H NMR (300 MHz, CDCl3): 7.32 (m, 4 H, HC(8,9,10,11)), 7.21 (m, 1 H, HC(12)), 3.70 (d, J = 10.8, 1 H, HC(1)), 3.52 (d, J = 10.8, 1 H, HC(1)), 1.76 (ddd, J = 13.3, 12.2, 4.3, 1 H, HC(3)), 1.50 (ddd, J = 13.0, 12.6, 4.3, 1 H, HC(3)), 1.34 (s, 3 H, H3C(13)), 1.23 (m, 2 H, H2C(5), 1.15 (m, 2 H, HC(4)), 0.83 (t, J = 7.1, 3 H, H3C(13)); 13C NMR (75.5 MHz, CDCl3): 144.83 C(7), 128.36 C(9,11), 126.68 C(8,12), 126.02 C(10), 72.67 C(1), 43.35 C(2), 38.26 C(3), 25.94 C(4), 23.38 C(13), 21.43 C(5), 13.99 C(6); IR (neat): 3550w,br, 3355m,br, 3088w, 3058w, 3025w, 2957s, 2932s, 2870s, 1940w, 1870w, 1800w, 1740w, 1601w, 1590w, 1497m, 1468m, 1445m, 1379m, 1310w, 1270w, 1195w, 1161w, 1096w, 1026s, 998w, 957w, 909w, 764m, 698s; MS (70 eV): 192 (M+, 3), 161 (40), 119 (16), 105 (100), 91 (64); Anal. Calcd for C12H20O: C, 81.07; H, 10.49. Found: C, 81.20; H, 10.48; TLC: Rf 0.36 (hexane/EtOAc, 5:1); GC: tR: 21.72 min (HP-5; 70 ˚C (5 min), 10 ˚C/min, 200 ˚C (15 min)).
Preparation of (R)-2-Phenyl-2,3,3-trimethylbutanol ((R)-25). A 15-mL, three-necked, round-bottomed flask fitted with a gas dispersion tube, gas exit valve, stirring bar and thermometer was charged with (S)-3-phenyl-3,4,4-trimethyl-1-pentene (R)-18 (0.100 g, 0.546 mmol), CHCl3 (0.20 mL), and MeOH (1.5 mL). The solution was cooled to -42 ˚C while being purged with oxygen. Ozone was then passed through the solution until the faint blue color of excess ozone appeared (15 min). The solution was purged with nitrogen and a solution of NaBH4 (0.278 g, 7.35 mmol) in 50% aqueous EtOH (3 mL) was added dropwise at -30 ˚C to room temperature over 1 h. The resulting mixture was stirred overnight and the reaction was diluted with Et2O (30 mL). A solution of 1 N HCl (7 mL) was added dropwise until all the borate salts had dissolved. The layers were separated and the aqueous layer was extracted with Et2O (2x30 mL). The organic layers were combined, dried (Na2SO4), filtered and concentrated in vacuo. The residue was purified by column chromatography on silica gel with hexane/EtOAc, 5:1 to yield 30 mg (30%) of (R)-25 as a white solid which was analytically pure. Analytical data from (R)-25: mp 60 - 63 ˚C; Optical Rotation: [α]D24 +5.43° (c 1.2, CHCl3), 85:15 er; 1H NMR (300 MHz, CDCl3): 7.35 (m, 4 H, 4 ArH), 7.24 (m, 1 H, HC(8)), 4.45 (d, J = 11.1, 1 H, HC(1)), 3.70 (d, J = 10.9, 1 H, HC(1)), 1.46 (s, 3 H, H3C( 7)), 1.41 (s, 1 H, OH), 0.84 (s, 9 H, H3C( 4,5,6)); 13C NMR (75.5 MHz, CDCl3): 142.50 C(8), 128.78 C(10), 127.77 C(9), 126.00 C(11), 66.64 C(1), 48.81 C(2), 35.49 C(3), 26.45 C(4,5,6), 19.30 C(7); IR (neat): 3596w, 3100w, 3061w, 2979s, 2907m, 2876w, 1595w, 1501w, 1482w, 1460w, 1443w, 1402w, 1375m, 1364m, 1269w, 1210w, 1205w, 1170w, 1135w, 1036s, 1021m, 915w; MS (70 eV): 192 (M+, 4), 161 (36), 147 (12), 136 (16), 135 (65), 134 (21), 131 (12), 119 (57), 118 (93), 117 (64), 116 (11), 115 (20), 106 (29), 105 (88), 103 (24), 93 (17), 92 (12), 91 (63), 79 (18), 78 (20), 77 (29), 65 (11), 57 (100), 55 (11), 51 (15); Anal. Calcd for C12H20O: C, 81.07; H, 10.49. Found: C, 81.20; H, 10.49; TLC: Rf 0.36 (hexane/EtOAc, 5:1); GC: tR: 21.72 min (HP-5; 70 ˚C (5 min), 10 ˚C/min, 200 ˚C (15 min)).
ACKNOWLEDGEMENTS
We are grateful to the National Science Foundation for generous financial support (NSF CHE8515371 and 8818147.
References
1. For recent reviews, see: (a) I. Denissov and L. Barriault, Tetrahedron, 2003, 59, 10105; CrossRef (b) C. J. Douglas and L. E. Overman, Proc. Natl. Acad. Sci., USA, 2004, 101, 5363; CrossRef (c) B. M. Trost and J. Chunhui, Synthesis, 2006, 369; CrossRef (d) For a recent monograph, see: Quaternary Stereocenters: Challenges and Solutions for Organic Synthesis, (ed. by J. Christoffers and A. Baro), Wiley-VCH, Weinheim, 2005.
2. (a) I. Marek and G. Sklute, Chem. Commun., 2007, 1683; CrossRef (b) S. E. Denmark and J. Fu, Org. Lett., 2002, 4, 1951. CrossRef
3. C. Spino in The Chemistry of Organocopper Compounds, Part 2, (ed. by Z. Rapoport and I. Marek), Wiley-Interscience, Chichester, 2009, Chapter 13.
4. B. Breit and P. Demel, in Modern Organocopper Chemistry, (ed. by N. Krause), Wiley-VCH, Weinheim, 2002, Chapter 6.
5. (a) A. Alexakis, P. Mangeney, A. Ghribi, I. Marek, R. Sedrani, C. Guir, and J. Normant, Pure Appl. Chem., 1988, 60, 49; CrossRef b) H. Rakotoarisoa, R. Guttierez Perez, P. Mangeney, and A. Alexakis, Organometallics, 1996, 15, 1957. CrossRef
6. For recent reviews see: (a) H. Yorimitsu and K. Oshima, Angew. Chem. Int. Ed., 2005, 44, 4435; CrossRef (b) C. A. Falciola and A. Alexakis, Eur. J. Org. Chem., 2008, 3765; CrossRef (c) A. Alexakis, J. E. Bäckvall, N. Krause, O. Pámies, and M. Diéguez, Chem. Rev., 2008, 108, 2796; CrossRef (d) S. R. Harutyunyan, T. den Hartog, K. Geurts, A. J. Minnaard, and B. L. Feringa, Chem. Rev., 2008, 108, 2824. CrossRef
7. (a) J. J. Van Veldhuizen, J. E. Campbell, R. E. Guidici, and A. H. Hoveyda, J. Am. Chem. Soc., 2005, 127, 6877; CrossRef (b) M. A. Kacprzynski, T. L. May, S. A. Kazane, and A. H. Hoveyda, Angew. Chem. Int. Ed., 2007, 46, 4554; CrossRef (c) H. Seo, D. Hirsch-Weil, K. A. Abboud, and S. Hong, J. Org. Chem., 2008, 73, 1983. CrossRef
8. (a) Y. Lee and A. H. Hoveyda, J. Am. Chem. Soc., 2006, 128, 15604; CrossRef (b) Y. Lee, B. Li, and A. H. Hoveyda, J. Am. Chem. Soc., 2009, 131, 11625; CrossRef (c) K. Kobayashi, M. Ueno, H. Naka, and Y. Kondo, Chem. Eur. J., 2009, 15, 9805; CrossRef (d) O. Jackowski and A. Alexakis, Angew. Chem. Int. Ed., 2010, 49, 3346; CrossRef (e) J. A. Dabrowski, F. Gao, and A. H. Hoveyda, J. Am. Chem. Soc., 2011, 133, 4778. CrossRef
9. (a) K. Ishihara, H. Nakamura, and H. Yamamoto, Synlett, 2001, 1113; CrossRef (b) H. Nakamura, K. Ishihara, and H. Yamamoto, J. Org. Chem., 2002, 67, 5124. CrossRef
10. S. E. Denmark and L. K. Marble, J. Org. Chem., 1990, 55, 1984. CrossRef
11. (a) C. Gallina and P. G. Ciattini, J. Am. Chem. Soc., 1979, 101, 1035; CrossRef (b) H. L. Goering, S. S. Kantner, and C. C. Tseng, J. Org. Chem., 1983, 48, 715; CrossRef (c) T. Underiner and H. L. Goering, J. Org. Chem., 1989, 54, 3239. CrossRef
12. (a) B. Breit and D. Breuninger, Synthesis, 2005, 147; CrossRef (b) B. Breit and Y. Schmidt, Chem. Rev., 2008, 108, 2928. CrossRef
13. V. Theus and H. Schinz, Helv. Chim. Acta, 1956, 39, 1290. CrossRef
14. (a) S. T. Young, J. R. Turner, and D. S. Tarbell, J. Org. Chem., 1963, 28, 928; CrossRef (b) K. Tanaka, N. Yamagishi, R. Tanikaga, and A. Kaji, Bull. Chem. Soc. Jpn., 1979, 52, 3619; CrossRef (c) K. Schmidt, N. H. Chishti, and T. Breining, Synthesis, 1982, 391. CrossRef
15. The chemical shifts for the cyclohexyl methine protons in (E)-6 and (E)-6 were unique, with δ = 1.95 (multiplet) for (E)-6 and δ = 3.60 (tt, J = 11.5, 3.2) for (Z)-6. The 13C resonances for the allylic methyl carbons were also diagnostic, with carbon 4 of (E)-6 at δ = 17.2 ppm and that of (Z)-6 at δ = 20.9 ppm, consistent with the characteristic chemical shifts of allylic methyl groups in E- and Z-trisubstituted olefinic systems.
16. (a) N. Okukado and E. Negishi, Tetrahedron Lett., 1978, 2357; CrossRef (b) E. Negishi, Pure Appl. Chem., 1981, 53, 2333; CrossRef (c) E. Negishi and D. E. van Horn, J. Am. Chem. Soc., 1978, 100, 2252. CrossRef
17. (a) A. DeBoer and J. A. Hunter, J. Org. Chem., 1973, 38, 144; CrossRef (b) A. C. Weedon, Can. J. Chem., 1984, 62, 1933; CrossRef (c) M. S. Newman, and R. Rosher, J. Org. Chem., 1944, 9, 221; CrossRef (d) H. Taguchi, K. Shimoji, H. Yamamoto, and H. Nozaki, Bull. Chem. Soc. Jpn., 1974, 47, 2529. CrossRef
18. (a) R. Heilmann and R. Glenat, Bull. Chim. Soc. Fr., 1955, 1586; (b) C. Roux and H. Daoust, Can. J. Chem., 1973, 51, 288. CrossRef
19. (a) J. Dobney, A. Johnson, G. Armand, and R. Kon, J. Chem. Soc., 1926, 2748; (b) C. R. Hauser and J. K. Lindsay, J. Am. Chem. Soc., 1955, 77, 1050; CrossRef (c) E. E. Royals and E. R. Covington, J. Am. Chem. Soc., 1955, 77, 1697; CrossRef (d) E. G. E. Hawkins and R. D. Thompson, J. Chem. Soc., 1961, 70.
20. For all different substrates, reactions of the racemic carbamates were carried out to prepare the racemic alkenes which were degraded to racemic analytes for calibration of the HPLC analytical method for enantiomeric composition determination (see Scheme 10).
21. (a) J. Tanaka, M. Nojima, and S. Kusabayashi, J. Am. Chem. Soc., 1987, 109, 3391; CrossRef (b) I. Fleming, S. K. Patel, and C. J. Urch, J. Chem. Soc., Perkin Trans. 1, 1989, 115. CrossRef
22. (a) E. Nakamura and S. Mori, Angew. Chem. Int. Ed., 2000, 39, 3750; CrossRef (b) S. Mori and E. Nakamura, in Modern Organocopper Chemistry, (ed. by N. Krause), Wiley-VCH, Weinheim, 2002, Chapter 10; (c) M. Yamanaka, S. Kato, and E. Nakamura, J. Am. Chem. Soc., 2004, 126, 6287; CrossRef (d) E. Nakamura and N. Yoshikai, in The Chemistry of Organocopper Compounds, Part 1, (ed. by Z. Rapoport and I. Marek), Wiley-Interscience, Chichester, 2009, Chapter 1.
23. (a) J. T. B. H. Jastrzebski and G. van Koten, in Modern Organocopper Chemistry, (ed. by N. Krause), Wiley-VCH, Weinheim, 2002, Chapter 1; (b) R. M. Gschwind, Chem. Rev., 2008, 108, 3029; CrossRef (c) G. van Koten and J. T. B. H. Jastrzebski, in The Chemistry of Organocopper Compounds, Part 1, (ed. by Z. Rapoport and I. Marek), Wiley-Interscience, Chichester, 2009, Chapter 2.
24. (a) J. H. van der Hende and W. C. Baird, Jr., J. Am. Chem. Soc., 1963, 85, 1009; CrossRef (b) N. C. Baenziger, H. L. Haight, and J. R. Doyle, Inorg. Chem., 1964, 3, 1535; CrossRef (c) R. G. Salomon and J. K. Kochi, J. Am. Chem. Soc., 1973, 95, 1889; CrossRef (d) R. G. Salomon and J. K. Kochi, J. Chem. Soc., Chem. Comm., 1972, 559; (e) R. G. Salomon and J. K. Kochi, J. Am. Chem. Soc., 1973, 95, 3300; CrossRef (f) R. G. Salomon and J. K. Kochi, J. Organomet. Chem., 1974, 64, 135; CrossRef (g) D. L. Reger and M. D. Dukes, J. Organomet. Chem., 1976, 113, 173. CrossRef
25. (a) G. Hallnemo, T. Olsson, and C. Ullenius, J. Organomet. Chem., 1984, 265, C22; CrossRef (b) G. Hallnemo and C. Ullenius, J. Organomet. Chem., 1985, 282, 133; CrossRef See also (c) E. J. Corey and N. W. Boaz, Tetrahedron Lett., 1985, 26, 6015; CrossRef (d) E. J. Corey and N. W. Boaz, Tetrahedron Lett., 1984, 25, 3059; CrossRef (e) E. J. Corey and N. W. Boaz, Tetrahedron Lett., 1984, 25, 3063. CrossRef
26. S. R. Krauss and S. G. Smith, J. Am. Chem. Soc., 1981, 103, 141. CrossRef
27. S. H. Bertz, S. Cope, M. Murphy, C. A. Ogle, and B. J. Taylor, J. Am. Chem. Soc., 2007, 129, 7208. CrossRef
28. (a) K. R. Stewart, J. R. Lever, and M.-H. Whangbo, J. Org. Chem., 1982, 47, 1472; CrossRef (b) E. C. Ashby and J. J. Watkins, J. Am. Chem. Soc., 1977, 99, 5312; CrossRef (c) H. O. House and J. M. Wilkins, J. Org. Chem., 1978, 43, 2443. CrossRef
29. (a) G. van Koten and J. T. B. H. Jastrzebski, J. Am. Chem. Soc., 1985, 107, 697, and references therein; CrossRef (b) G. van Koten and J. G. Noltes, J. Am. Chem. Soc., 1979, 101, 6593. CrossRef
30. (a) R. K. Dieter and M. Tolkes, J. Am. Chem. Soc., 1987, 109, 2040; CrossRef (b) G. Hallnemo and C. Ullenius, Tetrahedron Lett., 1986, 27, 395; CrossRef (c) K. Tanaka, H. Ushio, and H. Suzuki, J. Chem. Soc., Chem. Commun., 1990, 795; CrossRef (d) K. Yamamoto, M. Kanoh, N. Yamamoto, and J. Tsuji, Tetrahedron Lett., 1987, 28, 6347. CrossRef
31. B. E. Rossiter and M. Eguchi, Tetrahedron Lett., 1990, 31, 965. CrossRef
32. W. C. Still, M. Kahn, and A. Mitra, J. Org. Chem., 1978, 43, 2923. CrossRef
33. L. M. Harwood, Aldrichimica Acta, 1985, 18, 25.
34. H. Gilman and F. K. Cartledge, J. Organomet. Chem., 1964, 2, 447. CrossRef
35. W. G. Kofron and L. M. Baclawski, J. Org. Chem., 1976, 41, 1879. CrossRef
36. F. W. Sum and L. Weiler, Org. Synth., 1984, 62, 14.
37. (a) P. G. M. Wuts, Synth. Commum., 1981, 11, 139; CrossRef b) H. O. House, C. Y. Chu, J. M. Wilkens, and M. J. Umen, J. Org. Chem., 1975, 40, 1460. CrossRef
38. (a) H. G. Kolloff, J. H. Hunter, E. H. Woodruff, and R. B. Moffett, J. Am. Chem. Soc., 1948, 70, 3862; CrossRef (b) J. C. Craig and W. E. Pereira, Jr., Tetrahedron, 1971, 27, 1173. CrossRef
39. (a) W. C. Still and C. Gennari, Tetrahedron Lett., 1983, 24, 4405; CrossRef (b) H. J. Bestmann, H. Dornauer, and K. Rostock, Chem. Ber., 1970, 103, 685; CrossRef (c) E. Negishi, G. Lew, and T. Yoshida, J. Org. Chem., 1974, 39, 2321. CrossRef
40. (a) Y. Kuwahara and L. Sakuma, Agric. Biol. Chem., 1982, 46, 1855. CrossRef
42. (b) K. Ogura, T. Nishino, T. Koyama, and S. Seto, J. Am. Chem. Soc., 1970, 92, 6036. CrossRef