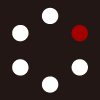
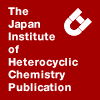
HETEROCYCLES
An International Journal for Reviews and Communications in Heterocyclic ChemistryWeb Edition ISSN: 1881-0942
Published online by The Japan Institute of Heterocyclic Chemistry
e-Journal
Full Text HTML
Received, 26th July, 2013, Accepted, 24th September, 2013, Published online, 27th September, 2013.
DOI: 10.3987/REV-13-SR(S)5
■ Syntheses of Chiral Heterocyclic Compounds via Zirconium-Catalyzed Asymmetric Carboalumination of Alkynes (ZACA Reaction)
Shiqing Xu and Ei-ichi Negishi*
Herbert C. Brown Laboratories of Chemistry, Purdue University, 560 Oval Drive, West Lafayette, Indiana 47907-2084, U.S.A.
Abstract
Shortly after the Zr-catalyzed carboalumination of alkynes was discovered in 1978, we sought expansion of the scope of this reaction so as to develop its alkene version for catalytic asymmetric C–C bond formation, namely the ZACA (Zr-catalyzed asymmetric carboalumination of alkenes). However, the discovery of such an asymmetric reaction proved to be quite challenging. The ZACA reaction was finally discovered in 1995 by suppressing three unwanted side reactions, i.e., (i) cyclic carbometalation, (ii) β-H transfer hydrometalation, and (iii) alkene polymerization, represented by the Ziegler-Natta polymerization. Three mutually complementary procedures for the enantioselective synthesis of methyl-substituted 1-alkanols have been developed, which allow highly flexible designs for the syntheses of chiral organic compounds. This review summarizes the syntheses of chiral heterocyclic compounds of biological and medicinal interest via ZACA reaction, which provides a widely applicable, efficient and selective method for catalytic asymmetric C–C bond formation.CONTENTS
1. Introduction
2. Synthesis of Chiral Heterocyclic Compounds via Zirconium-Catalyzed Asymmetric Carboalumination of Alkenes (ZACA reaction)
2-1. ZACA–Pd-Catalyzed Cross-Coupling Sequential Processes for the Synthesis of Deoxypolypropionates and Related Heterocyclic Compounds
2-2. ZACA–Lipase-Catalyzed Acetylation–Pd- or Cu-Catalyzed Cross-Coupling Synergy to Chiral Heterocyclic Compounds
1. Conclusion
2. Acknowledgements
3. References
1. INTRODUCTION
Palladium-catalyzed cross-coupling, especially those involving Zn, Al, Zr (Negishi coupling),1–3 and B (Suzuki coupling),4,5 has become one of the most widely applicable, selective, and satisfactory methods for C–C bond formation. Two regio- and stereodefined carbon groups generated as R1M (M = Zn, Al, B, Cu, Zr, etc.) and R2X (X = I, Br, Cl, OTs, etc.) may now be cross-coupled to give R1–R2 with essentially full retention of all structural features. It does appear that the synthetic scope of the Pd-catalyzed cross-coupling is limited primarily by the availability of satisfactory routes to the requisite R1M and R2X. From an optimistic viewpoint, one can state and expect that the synthetic scope of the Pd-catalyzed cross-coupling will continue to expand with discoveries and developments of new selective and satisfactory routes to R1M and R2X in the future.
The alkyne elementometalation–Pd-catalyzed cross-coupling tandem process has provided a very attractive, efficient, and selective route to various conceivable types of alkenes.6 In view of a large number of naturally occurring organic compounds of important biological activities containing trisubstituted alkenes, especially those with branching Me groups, it became highly desirable to have the corresponding carbometalation, especially methylmetalation, of alkynes. In 1978, we discovered the Zr-catalyzed carboalumination of alkynes.7 The Zr-catalyzed methylalumination of alkynes (ZMA reaction) was shown to involve one-step syn-addition of a Me–Zr bond to 1-alkynes in an anti-Markovnikov manner followed by Zr-to-Al transmetalation on the resultant carbon group.8,9 This reaction involves acyclic carbometalation of a "super-acidic"10,11 Zr-Al bimetallic system (Scheme 2).
Several years later, Dzhemilev reported a seemingly analogous reaction of Zr-catalyzed carbomagnesiation of alkenes with EtMgBr (Scheme 3).12 There did not appear any forcing reasons to suspect that the mechanisms of these two closely analogous reactions should be radically different. Through our systematic investigations of the "ZrCp2" chemistry,13 however, we accidentally clarified that the Dzhemilev ethylmagnesiation of alkenes actually proceeded through a highly intricate series of transformations involving (i) formation of Et2ZrCp2, (ii) β-agostic interaction-induced intramolecular "acid-base" interaction producing a zirconacyclopropane (1a) which may also be viewed as a zirconocene-ethylene π-complex (1b), (iii) carbozirconation of an alkene with 1 to give, typically a cyclic 3-substituted zirconacyclopentane 2, (iv) subsequent reaction of 2 with another molecule of EtMgBr leading to β-agostic interaction-induced “acid-base” interaction producing a 2-ethyl-1-alkylmagnesium bromide 3 with regeneration of the ethylene-ZrCp2 π-complex 1. All of the steps proposed above have been independently and amply supported (Scheme 3).13,14 We believe that both the discovery of the Dzhemilev ethylmagnesiation and our mechanistic clarification14 have not only clearly established the existence of both acyclic and cyclic carbozirconation processes but also alerted us to sharply and carefully distinguish some seemingly analogous carbometallation reactions of zirconocene derivatives.
We were later further surprised by the existence of bimetallic (involving both Zr and Al) cyclic carbozirconation of alkynes and alkenes that may be viewed as a hybrid of acyclic and cyclic carbozirconation (Scheme 4).15 We also noted that our bimetallic (Zr-Al) cyclic carbozirconation process closely resembled the corresponding carbotitanation of alkenes with titanium-carbene species that can be viewed as a two-membered titanacycle (Tebbe reagent) generated from a Ti-Al bimetallic system.16 Without going into detailed mechanistic discussions, the following brief summary may be presented: (i) Formation of metallacycles including metal-carbene complexes (two-membered metallacycles) is a widely
observable phenomenon with coordinatively unsaturated organotransition-metal complexes, especially in those cases where coordinatively unsaturated dialkylated organotransition-metal species, that are readily prone to β- or even α-agostic interaction-induced cyclization are generated.17 (ii) The propensity for generating the requisite "coordinatively unsaturated dialkyltransition-metal species" rests on a delicate balance between the alkylating power of the respective alkylmetal reagents, e.g., RLi > RMgX > RAlX2, and their ability to avoid formation of coordinatively saturated "ate" complexes. Thus, for example, trialkylalanes, e.g., Et3Al, do not dialkylate ZrCp2Cl2 to give Et2ZrCp2. On the other hand, Grignard
reagents, e.g., EtMgBr, readily dialkylate to give a 16 electron Et2ZrCp2. Triethylation does proceed, but it is readily reversible. All these make alkylmagnesium derivatives some of the optimal reagents for converting ZrCp2Cl2 into zirconacycles. (iii) Even with alkylalanes, however, zirconacycles may still be formed by "bimetallic intramolecular acid-base interaction" discussed above.
At the time we discovered the Zr-catalyzed carboalumination of alkynes in 1978,7 a dreamy thought of expanding the scope of this reaction so as to embrace its alkene version for asymmetric C–C bond formation, which would amount to the single-step version of the Ziegler-Natta alkene polymerization captured senior author’s mind. However, this seemingly easy task proved to be quite challenging, and several intermittent attempts over seventeen years supported heavily by our ongoing systematic investigations of zirconocene chemistry were needed to finally discover in 1995 the ZACA reaction,18,19 as detailed below.
Our initial investigations leading to negative results were conducted with the parent ZrCp2Cl2. It was finally decided to conduct the following two studies: (i) detailed fact-finding investigation of the reaction of 1-decene with 10 mol% of ZrCp2Cl2 in CH2Cl2 and (ii) search for satisfactory procedures based on (i). These studies immediately led to some most useful results shown in Scheme 5. With (Me5C5)2ZrCl2, no reaction was observed under the same conditions.18,19 Clearly, zirconocene derivatives with sufficiently, but not excessively, bulky ligands to suppress unwanted side reactions, most notably β-H transfer hydrometallation, while promoting the desired carbometallation, were needed to realize the desired alkene carbometallation.
Yet another ambush was the initially unexpected Al-Zr bimetallic cyclic carbometallation of alkenes. Before 1995, we believed that dialkylation of zirconocene derivatives would be mandatory for observing the formation of zirconacyclopropanes by β-agostic interaction-induced cyclization. It was, however, found that the reaction of 1-decene with Et3Al in the presence of Erker's (NMI)2ZrCl220 in hexanes would proceed by cyclic carbometallation (Scheme 6),19 even though there were ample indications that trialkylalanes do not lead to dialkylation of zirconocene derivatives. It was indeed this surprising finding that led to the clarification and establishment of the bimetallic cyclic mechanism for carbozirconation of alkynes mentioned earlier (Scheme 4).15 Fortunately, it was soon learned that the use of more polar solvents including CH2Cl2, MeCHCl2, and (CH2Cl)2 almost totally suppressed the undesired cyclic carbometallation process thereby promoting formation of the desired products (Scheme 6).19
Throughout the investigations, the authors’ group was very much concerned about the third potential side reaction, i.e., Zr-catalyzed alkene polymerization of Ziegler and Natta.21 However, this has hardly been of any serious concern. In retrospect, this is not surprising, if one considers (i) essentially 1:1 alkene-to-alane ratios and (ii) absence of highly efficient polymerization promoters, such as methylaluminoxane (MAO), typically required in large quantities relative to trialkylalanes. In the ZACA reaction,18,19 the use of MAO and other promoters is not mandatory and typically not necessary, although addition of one equivalent or less of water or the corresponding amount of preformed MAO can significantly promote an otherwise slow ZACA reaction,22,23 such as that of styrenes.
Having learned about three major pitfalls, namely (i) cyclic carbometallation, (ii) H-transfer hydrometallation, and (iii) Ziegler-Natta-type alkene polymerization as well as how to avoid them, the remaining major task was to find some satisfactory chiral zirconocene catalysts. In this respect, no systematic catalyst optimization involving catalyst design has as yet been made. Instead, a dozen to fifteen known chiral zirconocene complexes were initially screened. Widely used (EBI)ZrCl224 and its partially hydrogenated derivatives25 were less effective. The most effective among those tested thus far is Erker's (NMI)2ZrCl2.20 Although methylalumination is singularly important from the viewpoint of the synthesis of natural products, it is ironically the uniquely unfavorable case where the ee figures are around 75%, as compared with ethylalumination and higher alkylalumination which proceeds in 90–95% ee. An attractive alternative has been developed by taking advantage of high enantioselectivity observed in ethylalumination and higher alkylalumination (Scheme 7).26,27 This new protocol provides methyl substituted alkanols in 90–93% ee. Two critical points are notable in this reaction. First, β-unbranched primary alkyl groups in mixed trialkylalanes, generated in situ by hydroalumination of alkenes with DIBAH (HAli-Bu2) or transmetallation of alkyllithium compounds with ClAli-Bu2, selectively react to produce the desired products containing the β-unbranched primary alkyl groups. Second, IBAO (isobutylaluminoxane), which is prepared by mixing equimolar quantities of i-Bu3Al and H2O in CH2Cl2, can be used as cocatalyst to accelerate the reaction. MAO has similar effects, but the reaction can be complicated by the formation of methylaluminated products.
There are currently three Zr-catalyzed asymmetric carboalumination protocols that can be used for the synthesis of methyl-branched 1-alkanols (Scheme 8). As indicated in Scheme 8, addition of the Me–Al bond via ZACA reaction leads to 70–90% ee, typically 70–80%.27 The same products can be obtained in 85–95% ee by adding Et and higher alkyl groups and Al to propene and by using (NMl)2ZrCl2 of the opposite chirality. Both isomers of (NMI)2ZrCl2 are obtainable from the appropriate isomers of menthol with comparable ease. Moreover, addition of alkyl–Al bonds to free allyl28,29 and homoallyl alcohols as well as longer ω-alken-1-ols by ZACA reaction is generally high-yielding and highly enantioselective, typically around 90% ee.26,27 These three protocols can often be mutually complementary.30
2. SYNTHESIS OF CHIRAL HETEROCYCLIC COMPOUNDS VIA ZACA REACTION
2-1. ZACA–Pd-Catalyzed Cross-Coupling Sequential Processes for the Synthesis of Deoxypolypropionates and Related Heterocyclic Compounds
The ZACA reaction is a novel and as yet rare catalytic asymmetric C–C bond forming reaction of terminal alkenes of one-point-binding without requiring any other functional groups, even though various functional groups may be present. ZACA reaction has a high synthetic potential, since it provides organoaluminium intermediates which allow for a wide variety of further in situ transformations, such as oxidation, iodinolysis, hydrolysis, Pd-catalyzed cross-coupling, etc. Despite some room for improvement, especially (i) improvement of the enatioselectivity of carboalumination and (ii) realization of higher turnover numbers through elevation of the current level of 20–103 to ≥103–104 or higher, the ZACA reaction promises to provide a widely applicable, efficient, and selective asymmetric method for the synthesis of a variety of chiral organic compounds. In view of a large number of naturally occurring and biologically important deoxypolypropionates, intense efforts for the development of efficient and selective methods for their synthesis have been made.31 Most of the currently known and widely used methods for the constructions of deoxypolypropionates are chiral auxiliary-mediated 1,4-conjugate additions32 or enolate alkylations.33 Only a few other transition metal-catalyzed methods are known, of which one that involves catalytic asymmetric conjugate addition by B. L. Feringa34 is noteworthy. However, one homologation by one propylene unit appears to require typically three steps.
Despite the somewhat moderate ee values associated with methylalumination in ZACA reaction, the development of a few synthetic protocols and the exploitation of the well-known principle of statistical enantiomeric amplification have led to some efficient, selective, and practical processes for the synthesis of both α-monoheterofunctional and α,ω-diheterofunctional deoxypolypropionates and related compounds containing two or more asymmetric carbon atoms. According to the principle of statistical enantiomeric amplification, in the absence of internal chiral recognition, the stereochemical outcome of
combining two chiral species and/or asymmetric reactions may be predicted by resorting to the mass action law, as indicated for some representative cases in Table 1. For example, a process II of 80% ee followed by a process I of 90% ee would produce the desired product of 98.8% ee in a maximum 86% yield. The presence of three or more asymmetric carbon centers in the target molecules will make it possible to readily synthesize them in enantiomerically very pure forms (>99.9% ee).
Because most, if not all, of the possible stereoisomers of reduced polypropionate fragments are present in various natural products, any general methods for their synthesis must be capable of synthesizing all possible stereoisomers with comparable ease and without extensive procedural modifications. To this end, two synthetic protocols for the conversion of monomethylsubstituted 1-alkanols into 2,4-dimethyl-1-alkanols have been developed (Scheme 9).27 One is a three-step process involving (i) oxidation of alcohols to aldehydes, (ii) olefination by Wittig or other related reactions, and (iii) Zr-catalyzed methylalumination of alkene into 2,4-dimethyl-1-alkanols (2R, 4R)-6 and (2S, 4R)-6. The other protocol for chain elongation shown in Scheme 9 also involves a three-step process consisting of (i) iodination of alcohols, (ii) zincation followed by Pd-catalyzed vinylation, and (iii) Zr-catalyzed asymmetric methylalumination followed by oxidation with O2. This protocol is iterative, and it should be applicable to the synthesis of reduced polypropionates containing any number of branching methyl groups. An unexpected finding was that the diastereomeric separation of various 2,4-dimethyl- 1-hydroxybutyl derivatives can be readily purified by one round of ordinary chromatographic separation
using silica gel and hexanes–EtOAc to provide the desired major stereoisomers to ≥97–98% pure compounds. The modest preference for the formation of syn-2,4-dimethyl-1-alkanols over that of anti-2,4-dimethyl-1-alkanols was observed in these cases where 1-alkenes containing chiral alkyl groups were subjected to the ZACA reaction.27
Stereoisomers of 2,4-dimethyl-1-hexanols (6) have been used for the syntheses of some natural products, such as zaragozic acid A35 and (+)-sambutoxin36,37 (Scheme 10). (E)-α,β-Unsaturated acid 7 was prepared by oxidation of (2S, 4S)-6 under Swern conditions, condensation with Ph3P=CHCO2Me, and hydrolysis with lithium hydroxide in aqueous THF, which was an key intermediate for the synthesis of zaragozic acid A.35 Compound 8, which has been employed as a key intermediate in a recent synthesis of (+)-sambutoxin,37 was synthesized in 69% yield over three steps from (2S, 4R)-6.
Since the three-step protocol of (i) iodination, (ii) Pd-catalyzed vinylation, (iii) ZACA reaction followed by oxidation is iterative, and it has been applied to the synthesis of higher reduced polypropionates as shown in Scheme 11.27 2,4-Dimethyl-1-alkanols 6 was readily converted to 2,4,6-trimethyl-1-alkanols 9
by iterative three-step protocol. For this all-syn isomer case, dr of 8.5/1, corresponding to 89.5% stereoselectivity at C2, has been observed. The desired major stereoisomer (2S,4S,6S)-9 was readily purified to >98% purity by one round of ordinary chromatographic separation. As discussed earlier, the overall enantiomeric excess for this trimethyl derivative 9 may safely be estimated to be 99.9%. This trimethyl trimethyl-1-octanol 9 was further converted to compound 10 in two steps, which corresponds to the C11–C20 fragment of antibiotics TMC-151 A–F (11) without the sugar moiety.
One-Pot ZACA–Pd-Catalyzed Vinylation Tandem Process for One-Step Iterative Homologation by a Propylene Unit. Initially, the author’s group used a three-step iterative homologation cycle for incorporation of one propylene unit,27 which consisted of (i) ZACA-oxidation, (ii) iodination, and (iii) metalation–Pd-catalyzed vinylation. Since the initial ZACA reaction product is an alkylalane, its direct use in the Pd-catalyzed vinylation was explored by skipping oxidation and iodination. Thus, a highly efficient one-pot ZACA–Pd-catalyzed vinylation tandem process for one-step iterative homologation by a propylene unit has been developed.38 The isoalkyldimethylalanes, generated by ZACA reaction, was directly used for Pd-catalyzed vinylation under the condition of (i) Zn(OTf)2 as an additive, (ii) Pd(DPEphos)Cl2 and i-Bu2AlH in a 1:2 molar ratio as a catalyst system, and (iii) DMF as a solvent. The ZACA reaction of 1-octene proceeded in 75% ee (Mosher ester analysis of 2-methyl-1-octanol after oxidation). After Pd-catalyzed vinylation at elevated temperature (even at 120 °C), the product 12 was formed in 75% ee, which was determined by HPLC analysis of the amide derivative of its oxidation product 3-methylnonanoic acid. Thus, no racemization took place under the conditions of the Pd-catalyzed vinylation.
This one-pot ZACA–Pd-catalyzed vinylation tandem process has been used to the synthesis of α,ω-diheterofunctional deoxypolypropionates and related compounds containing two or more asymmetric carbon atoms. Compound 13 was prepared by ZACA reaction of inexpensive (<$1/mol) styrene followed by Pd-catalyzed vinylation of the in situ generated isoalkylalane promoted by Zn(OTf)2 (Scheme 13).38 Compound (S)-13 was then converted to (2S,4S)-2-methyl-4-phenyl-1-pentanol 14 by (+)-ZACA reaction followed by in situ oxidation with O2 in 50% yield over two steps from styrene. Since the first ZACA reaction gave 2-phenyl-1-propanol of 89% ee, the diastereomeric ratio (dr) of 7.0/1 observed by 13C NMR spectroscopy for (2S,4S)-14 before purification, indicated that the enantiomeric selectivity in the second (+)-ZACA reaction was 92% and that 14 should be 99% ee according to the principal of statistical enantiomeric amplification (Table 1). The undesired diastereomers of 14 could be separated by ordinary column chromatography. Compound (2S,4S)-14 was converted to tetrahydro-2H-pyran-2-one 15 by acetylation, Ru-catalyzed oxidation with NaIO4, and lactonization. Similarly, a syn isomer of (2S,4R)-14 was prepared in 47% yield over two steps as a 4.6/1 diastereomeric mixture by using (+)-(NMI)2ZrCl2 for both ZACA reaction steps. It is noteworthy that the diastereomeric ratio is higher for the formation of the anti isomer of 14, i.e., (2S,4S)-14 (dr = 7.0/1) than that of the syn isomer of 14, i.e., (2S,4R)-14 (dr = 4.6/1). The observed anti preference may be rationalized in terms of preferred equatorial disposition of the preexisting Me group in a putative pseudochair-like conformation arising from double π-complexation of a 14-electron MeZr(NMI)2 complex.38
α,ω-Diheterofunctional deoxypolypropionates tri- and tetramethyl-branched intermediates 16 and 20 have also been synthesized by this highly efficient all-catalytic asymmetric protocol (Schemes 14 and 15).38 The key point is to repeat the ZACA–Pd-catalyzed vinylation. The undesired diastereomers could be removed by ordinary column chromatography. Compounds 16 and 20 were transformed to compounds 18 and 21, respectively, which are the key intermediates employed in the previous syntheses of reduced polypropionate natural products ionomycin (19)39 and borrelidin (22).40
In 2006, we developed a novel “one-pot” conversion of inexpensive allyl alcohol to TBS-protected (R)- or (S)-3-iodo-2-methyl-1-propanol (24) via (i) ZACA reaction of allyl alcohol with Me3Al, (ii) in situ iodinolysis with I2, and (iii) in situ protection with tBuMe2SiCl (Scheme 16).28 The entire one-pot reaction gave either R or S isomer of 24 in 82% isolated yield by using (–)- or (+)-(NMI)2ZrCl2, respectively. (R)- or (S)-3-Iodo-2-methyl-1-propanol (23) were also readily prepared in 80% isolated yield with 82% ee by the same protocol except hydrolysis with H2O was used instead of in situ protection with tBuMe2SiCl. It should be noted that iodinolysis of the in situ generated isoalkylalane leading to the synthesis of 23 and 24 in high yields was a crucial finding since neither oxidation nor protonolysis of the isoalkylalane intermediate would lead to chiral products.
Compound 24, readily prepared from inexpensive allyl alcohol by one step, is a very useful intermediate to synthesize a wide variety of chiral compounds since it contains two functional groups, e.g. (i) substituting iodine with various carbon groups via Pd- or Cu-catalyzed cross-coupling, (ii) converting OTBS group to desired groups. For the synthesis of deoxypolypropionates and other chiral compounds containing two or more asymmetric carbon centers, compound 24 can be converted to alkene 25 by Pd-catalyzed vinylation in 87% yield. One-pot ZACA–Pd-catalyzed vinylation tandem process for one-step iterative homologation by a propylene unit can be also applied. It should be noted that since the ZACA reaction of 24 has been shown to be more syn-selective (syn/anti = 13/1) than anti-selective (anti/syn = 8/1),41 this protocol nicely complements an anti-selective styrene-based protocol discuss above.38 To demonstrate its synthetic utility, a key intermediate 28 for the synthesis of doliculide (29)42 have been prepared as summarized in Schemes 17.28 For the synthesis of compound 27, 25 (82% ee) was subjected to one round of the (+)-ZACA–Pd-catalyzed vinylation followed by the second (+)-ZACA reaction and oxidation with O2. The crudely isolated 27 (52% from 25) could be purified by ordinary column chromatography. Thus, 27 (dr = 43/1, >99% ee) was prepared in mere four isolation steps and one chromatographic purification from allyl alcohol in 25% overall yield.28 Compound 27 was further converted to 28 in 77% yield over 4 steps.
(–)-Spongidepsin (30), isolated from the Vanuatu marine sponge Spongia sp., displays cytotoxic and antiproliferative activities against J774.A1, HEK-293, and WEHI-164 cancer cell lines.43 We reported an efficient total synthesis of (–)-spongidepsin (30) by using 31, 32, and 33 through application of the
esterification–amidation–ring closing metathesis44 strategy employed in the previous syntheses45 (Scheme 18).46 The C1–C5 fragment (31) and the C6–C13 fragment (32) were synthesized via ZACA reaction as key steps. The synthesis of 31 was achieved via the two-step conversion of allyl alcohol into 25 via 24 in 71% yield and 82% ee, followed by one-pot ZACA–oxidation tandem process and chromatographic purification to give 34 in 32% overall yield (dr ≥ 40/1) from allyl alcohol (Scheme 18). Compound 34 was converted to 35 via Swern oxidation and Wittig olefination, and 35 was then converted to 31 via desilylation with TBAF, followed by PDC oxidation (18% over 7 steps from allyl alcohol).46 Compound 32 was synthesized from inexpensive 1,5-pentanediol in 20% overall yield via TBDPS-protection, Swern oxidation and Brown allylboration47 to give 36 in 74% over 3 steps. Conversion of 36 via ZACA reaction followed by in situ oxidation with O2 gave 37 (dr = 3.5/1) in 73% yield (43% after chromatographic purification to dr = 40/1). Oxidation of 37, followed by reduction with DIBAL-H and olefination by the Wittig reaction provided 32 in 64% yield in 3 steps (20% yield from 1,5-pentanediol over 7 steps). The final assembly of (–)-spongidepsin (30) was summarized in Scheme 18.
(+)-Scyphostatin (55), a naturally occurring ceramide analogue, is a promising pharmacological target for the treatment of inflammation, immunological and neurological disorders.48 We have developed one efficient and convergent total synthesis of (+)-scyphostatin (55)49 and two different routes to the side-chain (49).41,50 (+)-Scyphostatin (55) has been synthesized in the high level of convergency by amidation of compounds 53 and 54, followed by one step of deprotection (Scheme 19).49 Synthesis of compound 53 was performed by (i) oxidation of 49 with TPAP and NMO, (ii) olefination of the resultant aldehyde with 51, and (iii) hydrolysis with LiOH. For the synthesis of the side-chain (49), two different routes have been developed via ZACA reaction by treatment of 47 with two different intermediates 45 and 46, respectively (Scheme 19). Compound 47 was synthesized by iodination of 34 which was prepared by two rounds of ZACA reaction as discussed in Scheme 18 (30% over 4 steps from allyl alcohol). Compound 44 was prepared by asymmetric ethylalumination of TBDPS-protected allyl alcohol, desilylation, Swern oxidation, and Corey-Fuchs reaction. Hydrozirconation/iodinolysis of 44 provided (2E,4R)-2-iodo-4-methyl-2-hexene (45) of ≥98% isomeric purity in 71% yield. The C7–C16 side-chain (49) was then prepared by PdCl2(DPEphos)-catalyzed Negishi coupling of 45 with zinc derivative of 47 generated via treatment of 47 with t-BuLi (2.1 equiv) and dry ZnBr2 (0.65 equiv), followed by desilylation with TBAF.41 Our recently developed 1-halo-1-alkyne hydroboration–Negishi coupling–organoborate migratory insertion protocol has been used in another route to the side-chain 49 (Scheme 19).50 (Z)-1-Bromo-1-alkenylboranes 46 was prepared by treatment of 43 with LiHMDS and then with HBBr2 followed by addition of pinacol. Conversion of 46 with zinc derivative of 47 by Negishi coupling provided (Z)-alkenylborane 48 in ≥98% retention of configuration. Side-chain (49) was then synthesized by further transformation of 48 via (i) “ate” complexation with MeLi, (ii) I2-induced methyl migration, and (iii) hydroxy-induced anti-deiodoboration, followed by removal of the TBS group with TBAF (76% yield in two steps).50
2-2. ZACA–Lipase-Catalyzed Acetylation–Pd- or Cu-Catalyzed Cross-Coupling Synergy to Chiral Heterocyclic Compounds
Having developed unprecedentedly efficient methods for the synthesis of deoxypolypropionates with two or more stereogenic carbon centers as discussed above, it was acutely realized that, only if ZACA products containing just one stereogenic carbon center can be readily and predictably purified, the ZACA-based asymmetric synthetic method would become much more widely applicable. The senior author recently became fully aware of the following strengths and weaknesses of the previously known lipase-catalyzed (S)-selective acetylation: (i) Enantiomerically pure (R)-2-methyl-1-alkanols can be reliably obtained from their racemic mixtures, although the maximally attainable yield (or recovery) of (R)-alcohols of ≥98% ee is limited to 50% or, more specifically ≤25% if E = 10, ≤35% if E = 20, and ≤45% if E = 100, where E (enantiomeric ratio or selectivity factor) = ln[(1-C)(1-ee)]/ln[(1-C)(1+ee)] and C and ee are the extent of conversion and the enantiomeric excess of the unreacted alcohol, respectively.51 As such, it is not an attractive method, especially if the starting 2-methyl-1-alkanols are very expensive; (ii) Much more striking and important is that the lipase-catalyzed acetylation method is practically incapable of providing the ≥99% pure acetates of (S)-2-methyl-1-alkanols from their racemic mixtures in one cycle, since it can be predicted that the maximally attainable yields of ≥99% pure acetates would be ≤1–2% (E≤100).51 Consequently, iterative purification processes, in which the purity of desired compound must be gradually elevated, will be required. This theoretical prediction also points to a significant advantage in being able to start with enantiomerically enriched (S)-2-methyl-1-alkanols as shown in Table 2. Some maximally attainable yields of ≥99% pure acetates of (S)-2-methyl-1-alkanols can be predicted as follows: ≤80% if the initial eeo is 70% and E is 50; ≤85% if eeo is 80% and E is 30; ≤95% if eeo is 90% and E is 20.51 It is clear that neither the ZACA reaction alone nor the lipase-catalyzed acetylation alone is capable of providing a satisfactory method for the synthesis of either R or S isomer of 2-methyl-1-alkanols of ≥99% isomeric purity but that a combination of the two would be, provided that (i) the ZACA reaction is sufficiently enantioselective, preferably 80–90% ee but minimally ≥70% ee and
(ii) the E values are sufficiently high, preferably ≥20–30. The ZACA–lipase-catalyzed acetylation sequential process has indeed been successfully applied to the purification of either R or S isomers of 2-methyl-1-alkanols, as represented by the results shown in Table 3.52 Thus, 2-alkyl-1-alkanols, even in some cases of lacking any proximal π-bonds or heterofunctional groups, have been efficiently synthesized in ≥98% ee by ZACA–lipase-catalyzed acetylation sequential protocol.
As discussed above, the efficiency of the lipase-catalyzed acetylation heavily depends on the E factor. In more demanding (feebly chiral) cases, especially when two alkyl groups are very similar, it is difficult to purify to >98% ee even from enantiomerically enriched mixtures by lipase-catalyzed acetylation. To overcome this difficulty, the ZACA–lipase-catalyzed acetylation–Pd- or Cu-catalyzed cross-coupling sequential process was considered and developed for the synthesis of various feebly chiral 2-alkyl-1-alkanols of >99% ee as outlined in Scheme 20.29 The first step involves a “one-pot” conversion of inexpensive allyl alcohol to various 2-substituted (R)- or (S)-3-iodo-1-propanols (56) by ZACA reaction followed by iodinolysis. The introduction of iodine here is based on two considerations: (i) iodides serve as excellent cross-coupling partners and (ii) iodine displays a high E factor which makes both (S)- and (R)-56 readily purifiable to the level of ≥99% ee by lipase-catalyzed acetylation. Moreover, enantiomerically pure (>99% ee) 56 and 57 can be readily and efficiently transformed further into a wide variety of feebly chiral 2-substituted 1-alkanols by Pd- or Cu-catalyzed cross-coupling to introduce various carbon groups with >99% retention of all carbon skeletal features.
This ZACA–lipase-catalyzed acetylation–Pd- or Cu-catalyzed cross-coupling synergy has been applied to highly efficient and enantioselective synthesis of various chiral compounds.28,29 Thus, (S)-23 of >99% ee, obtained by ZACA/iodolysis–lipase-catalyzed acetylation, was converted to 1,1-dibromo-alkene 58 of >99% ee in 74% yield over four steps. Compound 58 was further transformed to 59, a potential intermediate for the synthesis of callystatin A, by PdCl2(DPEphos)-catalyzed Negishi coupling reactions
where the second Negishi coupling proceeding with a clean stereoinversion.28 (R)-Arundic acid is a drug candidate for the treatment of acute ischemic stroke, as well as clinical development in other neurodegenerative diseases, such as Alzheimer’s disease and Parkinson’s disease.53 For the synthesis of (R)-arundic acid, (R)-60 of ≥99% ee, prepared by ZACA–lipase-catalyzed purification–Cu-catalyzed cross-coupling synergy (Scheme 21), was transformed into (R)-arundic acids in 98% yield by oxidation with NaClO2 in the presence of catalytic amounts of NaClO and 2,2,6,6-tetramethylpiperidin-1-yloxyl (TEMPO).29
The ZACA–lipase-catalyzed acetylation synergy has been widely applied to the syntheses of a variety of monochiral natural products as well as those multichiral compounds in which the chiral centers do not effectively interact among them for allowing efficient isomeric purification by ordinary column chromatography. This synergy was applied to an efficient synthesis of (2R,6R)-2,6,10-trimethylundecan-1-ol (63), which is a key intermediate54 for the synthesis of vitamins E and K as shown in Scheme 22.52 In our previous synthesis,54 the preparation of 63 as a stereoisomerically pure substance was achieved via three-step purification process involving formation–repeated recrystallization–hydrolysis of a bisurethane derived from 63 and p-phenylene diisocyanate, which was rather laborious and of unpredictable applicability. In the recent synthesis,52 1-alkanol 61 of 78% ee, prepared by ZACA reaction of the corresponding alkene followed by oxidation, was readily purified to 98% ee by Amano PS lipase-catalyzed acetylation in 58% recovery yield. After two-step conversion of 61 to 62 in 81% yield, a second ZACA–oxidation provided crude 63 in 78% yield (74% ee at C-2), which can also be purified to 98% ee at C-2 by Amano PS lipase-catalyzed acetylation. Thus, 63 (dr > 50/1 and >99.9% ee) was synthesized in 14% yield (4 steps and two purification operations from 6-methylhept-1-ene), which was readily converted to vitamins E and K as shown in Scheme 22.54
The ZACA–lipase-catalyzed acetylation process was also applied to an efficient and enantioselective synthesis of (–)-stellettamide B as shown in Scheme 23. Crude alcohol 68 prepared by the ZACA reaction of the corresponding terminal alkenes 67 in 76% and 75% ee, was purified by Amano PS lipase-catalyzed acetylation to >98% ee in 66% recovery.52 Oxidation of 68 of >98% ee with N-methylmorpholine N-oxide (NMO) and catalytic amount of tetrapropylammonium perruthenate (TPAP) produced aldehyde 69 which has been converted to the antifungal and cytotoxic (–)-stellettamide B.55
A large number of natural products and related compounds of biological and medicinal interest contain 2,4-dimethyl-1-penten-1,5-ylidene moieties and derivatives thereof. Nafuredin (70),56 a selective NADH-fumarate reductase inhibitor, milbemycin β3 (71),57 a macrolide antibiotics, (–)-bafilomycin A1 (72),58 a potent vacuolar H+-APTase inhibitor, are some of the representatives examples containing an (E)-trisubstituted alkene moiety, while discodermolide (73),59 an anticancer marine natural product, for example, contains a (Z)-trisubstituted alkene. Others including (–)-callystatin A (74),60 a potential antitumor polyketide, contain a disubstituted (E)-alkene.
With the goal of developing widely applicable, efficient, and stereo- and regioselective synthetic protocols, two protocols with 1,4-pentenyne (75) and its silyl derivatives (76) as five-carbon synthons have been developed for constructing 2,4-dimethyl-1-penten-1,5-ylidene derivatives via ZMA reaction and ZACA reaction (Scheme 24).61
(2R,4E,6E,8S)-2,4,8-Trimethyldeca-4,6-dienal (80), a key intermediate for synthesis of nafuredin (70),56 was prepared according to Proctocol I from 75 as shown in Scheme 25.61 The conjugated diene 78 (≥98% E,E) was synthesized via Pd-catalyzed cross-coupling of 77 with alkenylaluminum intermediate obtained from ZMA reaction of 75. Its ZACA reaction with (–)-(NMI)2ZrCl2 as a catalyst followed by oxidation with O2 provided 79 in 79% yield and 72% ee (C-2). Although this compound contains two asymmetric carbon centers with a relatively rigid (E,E)-diene moiety between them, it was difficult to purify 79 by column chromatography. On the other hand, 79 of ≥98% ee (C-2) was prepared by lipase-catalyzed acetylation using Amano PS lipase in 52% overall yield from 78. Oxidation of 79 with Dess-Martin periodinane provided 80 of ≥98% isomeric purity in 91% yield.
For an efficient and selective synthesis of 86, a potential key intermediate for the synthesis of milbemycin β3 (71),57 a 10-step synthesis converting 75 into 86 in 18% overall yield has been developed (Scheme 26).61 Zr-catalyzed methylalumination of 75, evaporation of the volatiles, ate complexation of the alane with n-BuLi, and addition of ethylene oxide in one pot produced 81 in 74% yield, after protection with TBDPSCl. The ZACA reaction of 81 with Me3Al (2.5 equiv) and 1% of (–)-(NMI)2ZrCl2 gave crude 82 of 75% ee in 78% yield. Its purification by lipase-catalyzed acetylation using Amano PS lipase provided 82 of 98% ee in 53% overall yield from 81. After conversion of 82 into 83 in 69% yield over 3 steps, hydrozirconation–transmetallation–Pd-catalyzed alkenyl-alkenyl coupling with alkenyl iodide 84 produced 85 in 81% yield, which was further converted to 86 of ≥98% isomerical purity in 81% yield over three steps.61 Alkenyl iodide 84 was prepared from commercially available 3-methyl-4-methoxybenzaldehyde in 36% overall yield over 5 steps with Pd-catalyzed Negishi alkynylation and Zr-catalyzed methylalumination as key steps.61
Synthesis of intermediate 9458 for the synthesis of (–)-bafilomycin A1 has been achieved as shown in Scheme 27.61 TIPS-protected 1,4-pentenyne 76, prepared by CuBr-catalyzed allylation of lithio derivatives of TIPS-protected ethynes with allyl bromide, was subjected to the ZACA reaction to produce crude 87 in 85% yield and 73% ee, which was readily purified to 97% ee by lipase-catalyzed acetylation. After conversion of 87 into 90 in 50% yield over five steps by oxidation, Brown crotylboration, desilylation, ZMA/iodolysis, and protection with TBSOTf, oxidation of 90 with NMO/OsO4 and NaIO4 produced 91 in 71% yield, which was converted to 94 of >98% purity in 72% yield over 3 steps.61
Trisubstituted alkenes including monoalkenes, conjugated dienes and oligoenes represent a wide variety of natural products. We have developed several simple and highly satisfactory procedures for synthesis of trisubstituted alkenes including both tail-to-head (T-to-H)50,62,63 and head-to-tail (H-to-T)64 construction directions. Four T-to-H routes have been developed for highly stereo- and regioselective synthesis of trisubstituted alkenes via (Z)-1-halo-1-alkenylboranes as key intermediates, prepared via hydroboration of 1-halo-1-alkynes (Scheme 28).50,63 Route I consists of (i) 1-halo-1-alkyne hydroboration, (ii) migratory insertion of 1-halo-1-alkenylboron derivatives, (iii) Zn-promoted transmetalative iodinolysis, and (iv) Pd-catalyzed Negishi cross-coupling with alkenylzincs.63 This protocol has been applied to the syntheses of the key intermediates of several natural products including archazolid A and B (103),65 discodermolide (73),59 and (–)-callystatin A (74).60
As shown in Scheme 29, the (–)-ZACA/oxidation of TBDPS-protected allyl alcohol produced crude 95 of 75% ee in 83% yield, which was readily purified to >98% ee by Amano PS lipase-catalyzed acetylation due to the notable selectivity-enhancing effect of proximal heterofunctional TBDPS group. After conversion of 95 to 1-halo-1-alkyne 96 in 77% yield over 3 steps, hydroboration of 96 followed by migratory insertion with dialkylzincs (3 equiv) gave alkenylboranes. Although their Pd-catalyzed Suzuki coupling had been problematic, their in situ transmetallation to the corresponding Zn derivatives with additional dialkylzincs (1 equiv) and sequential iodinolysis gave alkenyl iodide 97, which have been shown to readily undergo highly demanding Pd-catalyzed Negishi coupling, as exemplified by the synthesis of potential intermediates 99, 100, and 102 for archazolid A and B (103),65 discodermolide (73),59 and (–)-callystatin A (74)60 (Schemes 29), respectively. More recently, three other simple and highly efficient routes II, III, IV have also been developed in the authors’ group, which promise to provide ultimately satisfactory protocols to trisubstituted alkenes.50 Route IV has been applied to highly selective synthesis of side chain (49) of scyphostatin (55) from allyl alcohol as discussed in Scheme 19.50
Wipf and co-worker reported the first total synthesis of a marine natural product (+)-bistramide C (104) via a triple convergency and a late-stage linkage of acid 105 to ester 106 and spiroketal 107 by azide couplings (Scheme 30).66 The preparation of segment 105 began with the ZACA reaction of terminal alkene 108 in the presence of 2.8 mol% of (+)-(NMI)2ZrCl2, AlMe3 (4.3 equiv) and MAO (1.5 equiv) providing 109 in 78% yield and 83% ee. Oxidation of 109 with NaOCl and a catalytic amount of TEMPO, followed by a HWE reaction provided enoate 110 in 84% yield. After conversion of enoate 110 into 1,3-diol 111 via a DIBAH reduction followed by a Sharpless asymmetric epoxidation and reductive opening of the resulting epoxy alcohol with Red-Al, the 1,3-diol 111 was converted to 1,5-diol 112 by selective protection and desilylation with TBAF. The 1,5-diol 112 was further transformed into the aldehyde 113 in 64% over three steps. The construction of trans-2,6-substituted tetrahydropyrans 114 was achieved via a tandem bismuth(III)-initiated etherification–allylation process67 in 72% yield and >5:1 diastereomeric ratio. The key intermediate 105 was then prepared from 114 in six additional steps, which was used in the final assembly of (+)-bistramide C (104).66
Fluvirucinine A1 (115) is an aglycone of fluvirucin A1 exhibiting considerable inhibitory activity against influenza A virus.68 A convergent and highly efficient synthesis of fluvirucinine A1 (115) via 116, which was obtained from 117 and 118, has been achieved as shown in Scheme 31.69 Two enantioselective routes to 117 were achieved in a similar manner with ZACA–lipase-catalyzed acetylation as key steps. In Route I, crude 119 was obtained by the ZACA reaction of TBS-protected 4-penten-1-ol with Et3Al (2 equiv), isobutylaluminoxane (IBAO, 1 equiv), and just 0.1 mol% of (–)-(NMI)2ZrCl2 in 82% yield and 90% ee. Its lipase-catalyzed acetylation with Amano PS lipase gave 119 of ≥98% ee in 75% recovery. After six additional steps, including formation and reduction of an azide, (R)-117 of ≥98% ee was obtained in 38% yield over 8 steps from 4-penten-1-ol (Route I in Scheme 31). Similarly, 3-buten-1-ol was protected with TBSCl and was subjected to the ZACA reaction with Et3Al, IBAO, and 0.1 mol% of (–)-(NMI)2ZrCl2. The crude 121 was obtained in 84% yield and 90% ee, which was readily purified to the level of ≥98% ee in 80% recovery by Amano PS lipase-catalyzed acetylation. These results clearly indicate that the ZACA–lipase-catalyzed acetylation protocol promises to provide a very favorable route to 2-alkyl-1-alkanols, where the 2-alkyl group is ethyl or a higher primary alkyl group in high enantiomeric purity. After six additional well-known steps including cyanation and reduction with LiAlH4 of the formed nitrile, 117 of ≥98% ee was produced in 46% yield over 8 steps from 3-buten-1-ol (Route II in Scheme 31). The higher overall yield and the use of less expensive 3-buten-1-ol make Route II somewhat more attractive than Route I. Preparation of the other key intermediate 118 was achieved by conversion of (–)-(S)-β-citronellol (123) in 44% yield over nine steps, including the Brown crotylboration, OsO4-catalyzed oxidative alkene cleavage with NaIO4 used twice, Swern oxidation, and Wittig olefination. The final assembly of fluvirucinine A1 (115) was performed by amidation of 117 with 118, Ru-catalyzed ring closing metathesis (RCM), hydrogenation, and desilylation with TBAF. Thus, fluvirucinine A1 (115) was prepared in 34% yield over 13 steps from commercially available (–)-(S)-β-citronellol.69
3. CONCLUSION
The ZACA reaction is a novel and as yet rare catalytic asymmetric C–C bond forming reaction of terminal alkenes of one-point-binding without requiring any other functional groups, even though various functional groups may be present. Since it provides organoaluminium intermediates which allow for a wide range of in situ transformations, ZACA reaction has a high synthetic potential. Despite some room for improvement, especially (i) improvement of the enatioselectivity of carboalumination and (ii) realization of higher turnover numbers through elevation of the current level of 20–103 to ≥103–104 or higher via systematic rational design and screening of chiral ligands, even at the current level of development, ZACA reaction provides a widely applicable, efficient and selective method for catalytic asymmetric C–C bond formation, which has already been used to significantly modernize and improve syntheses of natural products including deoxypolypropionates, isoprenoids, and other heterocyclic compounds of biological and medicinal interest. We believe that ZACA reaction will be widely embraced by the organic synthetic community in the near future.
4. ACKNOWLEDGEMENTS
The senior author sincerely thanks all of his coworkers whose names appear in the publications cited herein. Our research works discussed above have been mainly supported by NSF, NIH, and Purdue University. We also thank Sigma-Aldrich, Albemarle, Boulder Scientific, and Teijin for their support.
References
1. E. Negishi, Acc. Chem. Res., 1982, 15, 340. CrossRef
2. E. Negishi, Bull. Chem. Soc. Jpn., 2007, 80, 233. CrossRef
3. E. Negishi, Angew. Chem. Int. Ed., 2011, 50, 6738. CrossRef
4. N. Miyaura and A. Suzuki, Chem. Rev., 1995, 95, 2457. CrossRef
5. N. Miyaura, K. Yamada, and A. Suzuki, Tetrahedron Lett., 1979, 20, 3437. CrossRef
6. E. Negishi, G. Wang, H. Rao, and Z. Xu, J. Org. Chem., 2010, 75, 3151. CrossRef
7. D. E. Van Horn and E. Negishi, J. Am. Chem. Soc., 1978, 100, 2252. CrossRef
8. E. Negishi, N. Okukado, A. O. King, D. E. Van Horn, and B. I. Spiegel, J. Am. Chem. Soc., 1978, 100, 2254. CrossRef
9. E. Negishi, D. E. Van Horn, and T. Yoshida, J. Am. Chem. Soc., 1985, 107, 6639. CrossRef
10. G. A. Olah, Angew. Chem., Int. Ed. Engl., 1993, 32, 767. CrossRef
11. E. Negishi, Chem. Eur. J., 1999, 5, 411. CrossRef
12. V. M. Dzhemilev, O. S. Vostrikova, and R. M. Sultanov, Izv. Akad. Nauk SSSR, Ser. Kim., 1983, 218.
13. E. Negishi and D. Y. Kondakov, Chem. Soc. Rev., 1996, 26, 417. CrossRef
14. T. Takahashi, T. Seki, Y. Nitto, M. Saburi, C. J. Rousset, and E. Negishi, J. Am. Chem. Soc., 1991, 113, 6266. CrossRef
15. E. Negishi, D. Y. Kondakov, D. Choueiry, K. Kasai, and T. Takahashi, J. Am. Chem. Soc., 1996, 118, 9577. CrossRef
16. K. C. Ott, E. J. M. deBoer, and R. H. Grubbs, Organometallics, 1984, 3, 223. CrossRef
17. E. Negishi and T. Novak, Chap. 10. 06, in: Comprehensive Organometallic Chemistry III, ed. by I. Ojima, Elsevier, Oxford, 2007, pp. 251–297. CrossRef
18. D. Y. Kondakov and E. Negishi, J. Am. Chem. Soc., 1995, 117, 10771. CrossRef
19. D. Y. Kondakov and E. Negishi, J. Am. Chem. Soc., 1996, 118, 1577. CrossRef
20. G. Erker, M. Aulbach, M. Knickmeier, D. Wingbermuhle, C. Krüger, M. Nolte, and S. Werner, J. Am. Chem. Soc., 1993, 115, 4590. CrossRef
21. H. G. Alt and A. Köppl, Chem. Rev., 2000, 100, 1205. CrossRef
22. P. Wipf and S. Ribe, Org. Lett., 2000, 2, 1713. CrossRef
23. S. Ribe, R. K. Kondru, D. N. Beratan, and P. Wipf, J. Am. Chem. Soc., 2000, 122, 4608. CrossRef
24. F. R. W. P. Wild, L. Zsolnai, G. Huttner, and H. H. Brintzinger, J. Organomet. Chem., 1982, 232, 233.
25. F. R. W. P. Wild, M. Wasiucionek, G. Huttner, and H. H. Brintzinger, J. Organomet. Chem., 1985, 288, 63. CrossRef
26. S. Huo, J. Shi, and E. Negishi, Angew. Chem. Int. Ed., 2002, 41, 2141. CrossRef
27. E. Negishi, Z. Tan, B. Liang, and T. Novak, Proc. Natl. Acad. Sci. USA, 2004, 101, 5782. CrossRef
28. B. Liang, T. Novak, Z. Tan, and E. Negishi, J. Am. Chem. Soc., 2006, 128, 2770. CrossRef
29. S. Xu, C.-T. Lee, G. Wang, and E. Negishi, Chem. Asian J., 2013, 8, 1829. CrossRef
30. For a recent review, see: E. Negishi, ARKIVOC, 2011, viii, 34.
31. For reviews, see: S. Hanessian, S. Giroux, and V. Mascitti, Synthesis, 2006, 7, 1057; CrossRef B. Horst, B. L. Feringa, and A. J. Minnaard, Chem. Commun., 2010, 46, 2535. CrossRef
32. W. Oppolzer, Tetrahedron, 1987, 43, 1969. CrossRef
33. D. A. Evans, R. L. Dow, T. L. Shih, J. M. Takacs, and R. Zahler, J. Am. Chem. Soc., 1990, 112, 5290. CrossRef
34. F. Lόpez, S. R. Harutyunyan, A. Minnaard, and B. L. Feringa, J. Am. Chem. Soc., 2004, 126, 12784; R. Des Mazery, M. Pullez, F. Lόpez, S. R. Harutyunyan, A. Minnaard, and B. L. Feringa, J. Am. Chem. Soc., 2005, 127, 9966; CrossRef F. Lόpez, S. R. Harutyunyan, A. Meetsma, A. Minnaard, and B. L. Feringa, Angew. Chem. Int. Ed., 2005, 44, 2752.
35. D. Stoermer, S. Caron, and C. H. Heathcock, J. Org. Chem., 1996, 61, 9115. CrossRef
36. M. Magnin-Lachaux, Z. Tan, B. Liang, and E. Negishi, Org. Lett., 2004, 6, 1425. CrossRef
37. D. R. Williams and R. A. Turske, Org. Lett., 2000, 2, 3217. CrossRef
38. T. Novak, Z. Tan, B. Liang, and E. Negishi, J. Am. Chem. Soc., 2005, 127, 2838. CrossRef
39. S. Hanessian, N. G. Cooke, B. DeHoff, and Y. Sakito, J. Am. Chem. Soc., 1990, 112, 5276; CrossRef D. A. Evans, R. L. Dow, T. L. Shih, J. M. Takacs, and R. Zahler, J. Am. Chem. Soc., 1990, 112, 5290; CrossRef M. Lautens, J. T. Colucci, S. Hiebert, N. D. Smith, and G. Bouchain, Org. Lett., 2002, 4, 1879. CrossRef
40. M. O. Duffey, A. LeTiran, and J. P. Morken, J. Am. Chem. Soc., 2003, 125, 1458; CrossRef S. Hanessian, Y. Yang, S. Giroux, V. Mascitti, J. Ma, and F. Raeppel, J. Am. Chem. Soc., 2003, 125, 13784; CrossRef B. G. Vong, S. H. Kim, S. Abraham, and E. A. Theodorakis, Angew. Chem. Int. Ed., 2004, 43, 3947; CrossRef T. Nagamitsu, D. Takano, T. Fukuda, K. Otoguro, I. Kuwajima, Y. Harigaya, and S. Omura, Org. Lett., 2004, 6, 1865. CrossRef
41. Z. Tan and E. Negishi, Angew. Chem. Int. Ed., 2004, 43, 2911. CrossRef
42. A. K. Ghosh and C. Liu, Org. Lett., 2001, 3, 63.
43. A. Grassia, I. Bruno, C. Debitus, S. Marzocco, A. Pinto, L. Gomez-Paloma, and R. Riccio, Tetrahedron, 2001, 57, 6257. CrossRef
44. R. H. Grubbs, S. J. Miller, and G. C. Fu, Acc. Chem. Res., 1995, 28, 446; CrossRef T. M. Trnka and R. H. Grubbs, Acc. Chem. Res., 2001, 34, 18; CrossRef A. Fürstner, Angew. Chem. Int. Ed., 2000, 39, 3012. CrossRef
45. J. Chen and C. J. Forsyth, Angew. Chem. Int. Ed., 2004, 43, 2148; CrossRef A. K. Ghosh and X. Xu, Org. Lett., 2004, 6, 2055; CrossRef L. Ferrié, S. Reymond, P. Capdevielle, and J. Cossy, Org. Lett., 2006, 8, 3441. CrossRef
46. G. Zhu and E. Negishi, Org. Lett., 2007, 9, 2771. CrossRef
47. H. C. Brown and P. K. Jadhav, J. Am. Chem. Soc., 1983, 105, 2092. CrossRef
48. V. Wascholowski and A. Giannis, Drug News Perspect., 2001, 14, 581.
49. E. Pitsinos, N. Athinaios, Z. Xu, G. Wang, and E. Negishi, Chem. Commun., 2010, 46, 2200. CrossRef
50. S. Xu, C.-T. Lee, H. Rao, and E. Negishi, Adv. Synth. Catal., 2011, 353, 2981. CrossRef
51. C.-S. Chen, Y. Fujimoto, G. Girdaukas, and C. J. Sih, J. Am. Chem. Soc., 1982, 104, 7294; CrossRef C.-S. Chen, and C. J. Shi, Angew. Chem., Int. Ed. Engl., 1989, 28, 695. CrossRef
52. Z. Huang, Z. Tan, T. Novak, G. Zhu, and E. Negishi, Adv. Synth. Catal., 2007, 349, 539. CrossRef
53. N. Tateishi, T. Mori, Y. Kagamiishi, S. Satoh, N. Katsube, E. Morikawa, T. Morimoto, T. Matsui, and T. Asano, J. Cereb. Blood. Flow. Metab., 2002, 22, 723; CrossRef L. A. Sorbera and J. Castaner, Drugs Future, 2004, 29, 441. CrossRef
54. S. Huo and E. Negishi, Org. Lett., 2001, 3, 3253. CrossRef
55. N. Yamazaki, W. Dokoshi, and C. Kibayashi, Org. Lett., 2001, 3, 193. CrossRef
56. S. Ōmura, H. Miyadera, H. Ui, K. Shiomi, Y. Yamaguchi, R. Masuma, T. Nagamitsu, D. Takano, T. Sunazuka, A. Harder, H. Kölbl, M. Namikoshi, H. Miyoshi, K. Sakamoto, and K. Kita, Proc. Nat. Acad. Sci. USA, 2001, 98, 60; CrossRef D. Takano, T. Nagamitsu, H. Ui, K. Shiomi, Y. Yamaguchi, R. Masuma, I. Kuwajima, and S. Ōmura, Tetrahedron Lett., 2001, 42, 3017; CrossRef D. Takano, T. Nagamitsu, H. Ui, K. Shiomi, Y. Yamaguchi, R. Masuma, I. Kuwajima, and S. Ōmura, Org. Lett., 2001, 3, 2289. CrossRef
57. H. Mishima, M. Kurabayashi, C. Tamura, S. Sato, H. Kuwano, A. Saito, and A. Aoki, Tetrahedron Lett., 1975, 10, 711; CrossRef A. B. Simith, III, S. R. Schow, J. D. Bloom, A. S. Thompson, and K. N. Winzenberg, J. Am Chem. Soc., 1982, 104, 4015; CrossRef S. R. Schow, J. D. Bloom, A. S. Thompson, K. N. Winzenberg, and A. B. Simith, III, J. Am Chem. Soc., 1986, 108, 2662; CrossRef D. R. Williams, B. A. Barner, K. Nishitani, and J. G. Phillips, J. Am Chem. Soc., 1982, 104, 4708; CrossRef R. Baker, M. J. O’Mahony, and C. J. Swain, J. Chem. Soc., Chem. Commun., 1985, 19, 1326; CrossRef S. D. A. Street, C. Yeates, P. Kocienski, and S. F. Campbell, J. Chem. Soc., Chem. Commun., 1985, 20, 1386; CrossRef C. Yeates, S. D. A. Stephen, P. Kocienski, and S. F. Campbell, J. Chem. Soc., Chem. Commun., 1985, 20, 1388; CrossRef P. J. Kocienski, S. D. A. Street, C. Yeates, and S. F. Campbell, J. Chem. Soc., Perkin Trans. 1, 1987, 10, 2171; CrossRef S. V. Attwood, A. G. M. Barrett, R. A. E. Carr, and G. Richardson, J. Chem. Soc., Chem. Commun., 1986, 6, 479; CrossRef M. Li and G. A. O’Doherty, Org. Lett., 2006, 8, 3987. CrossRef
58. D. A. Evans and M. A. Calter, Tetrahedron Lett., 1993, 34, 6871; CrossRef K. Toshima, T. Jyojima, H. Yamaguchi, H. Murase, T. Yoshida, S. Matsumura, and M. Nakata, Tetrahedron Lett., 1996, 37, 1069; CrossRef K. Toshima, H. Yamaguchi, T. Jyojima, Y. Noguchi, M. Nakata, and S. Matsumura, Tetrahedron Lett., 1996, 37, 1073; CrossRef K. Toshima, T. Jyojima, H. Yamaguchi, Y. Noguchi, T. Yoshida, H. Murase, M. Nakata, and S. Matsumura, J. Org. Chem., 1997, 62, 3271; CrossRef K. A. Scheidt, A. Tasaka, T. D. Bannister, M. D. Wendt, and W. R. Roush, Angew. Chem. Int. Ed., 1999, 38, 1652; CrossRef K. A. Scheidt, T. D. Bannister, A. Tasaka, M. D. Wendt, B. M. Savall, G. J. Fegley, and W. R. Roush, J. Am. Chem. Soc., 2002, 124, 6981; CrossRef J. A. Marshall and N. D. Adams, Org. Lett., 2000, 2, 2897; CrossRef J. A. Marshall and N. D. Adams, J. Org. Chem., 2002, 67, 733; CrossRef S. Hanessian, J. Ma, and W. Wang, J. Am. Chem. Soc., 2001, 123, 10200; CrossRef J.-C. Poupon, E. Demont, J. Prunet, and J.-P. Férézou, J. Org. Chem., 2003, 68, 4700. CrossRef
59. A. B. Smith, III, Y. Qiu, D. R. Jones, and K. Kobayashi, J. Am. Chem. Soc., 1995, 117, 12011; CrossRef S. S. Harried, G. Yang, M. A. Strawn, and D. C. Myles, J. Org. Chem., 1997, 62, 6098; CrossRef J. A. Marshall and B. A. Johns, J. Org. Chem., 1998, 63, 7885; CrossRef I. Paterson, G. J. Florence, K. Gerlach, and J. Scott, Angew. Chem. Int. Ed., 2000, 39, 377. CrossRef
60. N. W. Wang, M. Aoki, Y. Tsutsui, M. Sugimoto, and M. Kobayashi, Tetrahedron Lett., 1998, 39, 2349; CrossRef A. B. Smith, III and B. M. Brandt, Org. Lett., 2001, 3, 1685; CrossRef M. Kalesse, M. Quitschalle, C. P. Khandavalli, and A. Saeed, Org. Lett., 2001, 3, 3107; CrossRef J. A. Marshall and M. P. Bourbeau, J. Org. Chem., 2002, 67, 2751; CrossRef M. Lautens and T. A. Stammers, Synthesis, 2002, 14, 1993; CrossRef N. F. Langille and J. S. Panek, Org. Lett., 2004, 6, 3203. CrossRef
61. G. Zhu and E. Negishi, Chem. Eur. J., 2008, 14, 311. CrossRef
62. X. Zeng, Q. Hu, M. Qian, and E. Negishi, J. Am. Chem. Soc., 2003, 125, 13636; CrossRef X. Zeng, M. Qian, Q. Hu, and E. Negishi, Angew. Chem. Int. Ed., 2004, 43, 2259. CrossRef
63. Z. Huang and E. Negishi, J. Am. Chem. Soc., 2007, 129, 14788. CrossRef
64. E. Negishi, T. Tobrman, H. Rao, S. Xu, and C.-T. Lee, Isr. J. Chem., 2010, 50, 696. CrossRef
65. J. Hassfeld, C. Farès, H. Steinmetz, T. Carlomagno, and D. Menche, Org. Lett., 2006, 8, 4751; CrossRef D. Menche, J. Hassfeld, J. Li, and S. Rudolph, J. Am. Chem. Soc., 2007, 129, 6100. CrossRef
66. P. Wipf and T. D. Hopkins, Chem. Commun., 2005, 27, 3421. CrossRef
67. P. A. Evans, J. Cui, S. J. Gharpure, and R. J. Hinkle, J. Am. Chem. Soc., 2003, 125, 11456. CrossRef
68. N. Naruse, O. Tenmyo, K. Kawauo, K. Tomita, N. Ohgusa, T. Miyaki, M. Konishi, and T. Oki, J. Antibiot., 1991, 44, 733; CrossRef N. Naruse, T. Tsuno, Y. Sawada, M. Konishi, and T. Oki, J. Antibiot., 1991, 44, 741; CrossRef N. Naruse, M. Konishi, T. Oki, Y. Inouye, and H. Kakisawa, J. Antibiot., 1991, 44, 756. CrossRef
69. B. Liang and E. Negishi, Org. Lett., 2008, 10, 193. CrossRef