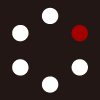
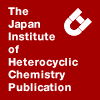
HETEROCYCLES
An International Journal for Reviews and Communications in Heterocyclic ChemistryWeb Edition ISSN: 1881-0942
Published online by The Japan Institute of Heterocyclic Chemistry
e-Journal
Full Text HTML
Received, 23rd August, 2013, Accepted, 11th November, 2013, Published online, 20th November, 2013.
DOI: 10.3987/COM-13-S(S)113
■ Advances in Siloxane-Based Coupling Reactions: Novel 16-Electron Palladium(0) Tri-alkene Catalysts for Allyl-Aryl Coupling in Precursors to Amaryllidaceae Alkaloids
Frederick E. Nytko, III, Krupa H. Shukla, and Philip DeShong*
Department of Chemistry and Biochemistry, University of Maryland, 7950 Baltimore Avenue, College Park, MD 20742, U.S.A.
Abstract
A new class of 16-electron Pd(0) catalysts are surveyed for applications in siloxane based allyl-aryl coupling protocols. The ability to "tune" the catalysts' activity by varying either the cone angle or the electronic characteristics of the alkene ligands attached to palladium was demonstrated. Attempts to prepare chiral adducts in the coupling reaction utilizing chiral bicyclooctadiene derivatives as a ligand for palladium provided no significant enantioenrichment in the coupled product.INTRODUCTION
Aryl siloxane derivatives, unlike their boronic acid counterparts, readily undergo Pd-catalyzed coupling.1-4 We have reported on both the regio- and stereochemical outcomes of this reaction in detail, and have utilized this strategy for the total synthesis of analogs of pancratistatin.5 The Amaryllidaceae alkaloids (+)-pancratistatin6,7 (1) and (+)-7-deoxypancratistatin8,9 (2) have garnered significant attention from the synthetic community due to their exceptional biological activity. Based on our studies with simple systems, we had anticipated that the siloxane coupling strategy could be employed for the regioselective and stereospecific coupling of ring A siloxane and a C-ring allylic carbonate to provide the coupling product 3 as outlined in Scheme 1. Numerous groups have approached the total synthesis of these natural products via the coupling of A-C ring precursors,10-31 but their methods have proven impractical due to low yields, lengthy preparations, and unstable coupling precursors. It was recently reported in the DeShong group, that the coupling of late-stage A-C ring analogues could be achieved (Scheme 1),5 leading to an intermediate in the Hudlicky synthesis of (+)-7-deoxypancratistatin.11
However, this transformation could not be completed using standard Pd(0) coupling catalysts. Based on a mechanistic study performed on the siloxane-based allyl-aryl coupling reaction in our lab, we proposed that palladium(0) complexes with sterically demanding, weakly associated ligands would be ideally suited for the siloxane coupling process. It was determined via mechanistic coupling studies, that the “ligandless”
alkene-based palladium(0) complexes best possessed these characteristics.32 While a variety of tridentate, 16-electron Pd(0) complexes of this general structure have been reported in the literature (Figure 1),33-44 few studies of their catalytic capabilities have been reported.35 In this manuscript, we report a systematic study of the siloxane-based allyl-aryl coupling technology45-47 employing tridentate Pd(0) catalysts.
RESULTS AND DISCUSSION
Based on the experience we have gained from our studies with pancratistatin analogs, the initial studies comparing the effectiveness of the 16-electron Pd(0) catalysts in the allyl-aryl coupling reactions were performed via the coupling of allylic carbonate 4 and aryl siloxane 5. We had previously reported that this coupling could be accomplished in good yield (70% isolated) using the typical 18-electron Pd(0) catalyst Pd(dba)2.32,45-47 The results summarized in Table 1 are a comparison of Pd(dba)2 (7) and a series of 16-electron Pd(0) catalysts based on the complexes reported by Itoh in 1977. Itoh reported the preparation of a series of bis-alkene-maleic anhydride complexes, 8 and 9, respectively, but never reported on the application of these 16-electron Pd(0) complexes as catalysts. Under a variety of reaction conditions, neither complex 8 nor 9 was as effective as Pd(dba)2 (7) in catalyzing the allyl-aryl coupling reaction (see Table 1). However, it is noteworthy that the norbornadiene complex 9 gave a higher yield of the coupled product than its COD analog 8 (38% vs. 54%, respectively). Since the only difference between these two complexes was the cone angle associated with the diene ligands (norbornadiene and cyclooctadiene (COD), respectively), it was concluded that the cone angle must have a significant effect on the stability or reactivity of the intermediate π-allyl complex in the coupling process. Catalyst 9 is more thermally stable than Pd-complex 8, its COD counterpart.
Complex 10, in which maleic anhydride has been replaced with tetracyanoethylene in a COD complex, has also been reported by Itoh. However, Pd-complex 10 was an ineffective coupling catalyst (see Table 1), and we attribute the low yields in this case with the instability of the complex under the typical coupling conditions, with palladium black rapidly precipitating during the reaction. We prepared analogous complexes 11 and 12 using the Itoh method, in which maleimide derivatives were substituted for maleic anhydride. The N-methyl maleimide complex 11 gave the best yield of the coupled product and demonstrated excellent stability under the coupling conditions. From these results, two major conclusions can be drawn: (1) norbornadiene (NBD) complexes give a higher yield of the allyl-aryl coupling product than the complementary COD analogs (comparing the yields when using complex 8 vs. 9, and the improved yield of coupled product with complex 11), and (2) the catalytic activity of the complexes can be "tuned" by modifying the electron-deficient alkene ligand (comparing the coupling yields for norbornadiene complexes 9-12).
As summarized above, we concluded from the results in Table 1 that cone angle and/or the sterics of the bis-alkene ligands, norbornadiene or cyclooctadiene, respectively, have an important role in determining the catalytic activity of the Pd(0) complex. Alkenes, such as norbornene and norbornadiene, possess a high binding affinity to the metal center, because of the relief of ring strain upon carbon rehybridization, as well as a reduction of steric hindrance. Thus, the effective cone angle of the diene ligand can greatly affect the rate of coupling.48 The metal-alkene bond is described as a combination of σ-donation from a filled alkene π-orbital to an empty metal orbital and π-back donation from a filled metal orbital to an empty alkene π* orbital.48,49 For an electron rich d10-configuration such as Pd(0), π-back donation from the palladium center to the alkene is extremely important. Stronger π-back donation results in a stronger palladium-alkene bond, thereby increasing the stability of the palladium complex, but reducing the lability of the alkene ligand and slowing transmetallation.
While the reactions reported in Table 1 were performed at 55 ºC, it is important to note that both Pd(COD)(MAH) (8) and Pd(NBD)(MAH) (9) catalyzed the allyl-aryl coupling reaction even at ambient temperature, which is not the case with Pd(dba)2. This result suggests that these catalysts are far more reactive than Pd(dba)2, even though they provide lower yields of the coupling product. The decrease in yield can be attributed to instability of these 16-electron complexes in solution, compared to Pd(dba)2. As noted above, complex 8 rapidly decomposes to palladium black under the coupling conditions, while complex 9 is more stable. While the preparation of Pd(NBD)(MAH) (9) was trivial, the preparation of Pd(COD)(MAH) (8) and Pd(COD)(TCNE) (10) (TCNE = tetracyanoethylene) was tedious and required careful handling due to their instability (precipitation of palladium black) when exposed to air at ambient temperature. Similarly, the incorporation of maleimides into the Pd(0) catalyst to give complexes 11 and 12, respectively, while providing good yields of the coupled product, were highly unstable at ambient temperature.
A comparison of the results in Table 1 also demonstrated that the catalytic activity, as well as stability, of the metal-alkene complex can be "tuned" by adjusting the electronic properties of the electron-deficient ligand on the metal center. There are two possible explanations for the excellent yields of the NBD complexes 9 and 11, respectively: (1) the NBD complexes have faster turnover rates than other catalysts, or (2) the NBD complexes were more stable than other catalysts. In the first scenario, if the rates of the catalytic cycle are faster for the NBD complexes, then more cycles occurred before the catalysts decomposed, and higher coupling yields are obtained. In the second case, if the catalysts are more stable, then more substrate could be processed before the catalysts decomposed, and higher yields of coupled product were achieved. Without a complete mechanistic study of this coupling process, it is not possible to differentiate between these two explanations.
At this point, there are several mechanistic factors that require additional consideration: as shown in Scheme 2, NBD complex 9 presumably undergoes π-allyl formation with concomitant loss of maleic anhydride to give complex A. Transmetallation of the aryl group from the silicate to palladium provides either complex B or C. Reductive elimination from B / C releases the coupled product and regenerates the catalyst. A full mechanistic study of this process is underway and will be reported in due course.
The general instability of the Itoh complexes 8-12 limited their application in the allyl-aryl coupling reaction. Accordingly, we chose to examine 16-electron Pd(0) complexes containing quinones, as prepared from Pd2dba3·CHCl3 by both the Sakai and Itoh groups.34,35 These complexes are significantly more stable at ambient temperature, and therefore more readily prepared without resorting to glove box protocols. When employed in the allyl-aryl coupling reaction, Pd(COD)(NQ) (13) gave superior yields in comparison to Pd(COD)(DQ) (14) and Pd(COD)(BQ) (15), indicating a strong electronic influence in the catalyst's performance. It is interesting to note that subtle changes, such as the inclusion of an aromatic ring from naphthoquinone (NQ) in the catalyst, dramatically improved the coupling yield compared to benzoquinone (BQ) and duroquinone (DQ). With duroquinone and benzoquinone acting as bidentate ligands, the palladium center has a full compliment of 18 electrons and no open coordination sites, theoretically leading to slower transmetallation and lower yields. A dinuclear Pd(0) complex possessing BQ as a bidentate ligand and NBE (norbornene) as a monodentate ligand was also prepared,35 however this catalyst (16) provided a poor coupling yield (Table 2).
Given that the intermediate π-allyl complex derived from cyclohexenyl carbonate is a meso-compound (Scheme 3; when L* is achiral), the coupling reaction provided a racemic product. On the other hand, enantioenrichment of the coupled product should be possible if chirality can be imparted upon the intermediate (Scheme 3) by the incorporation of a chiral bicyclooctadiene ligand into the catalyst. While the use of chiral dienes as ligands in transition-metal mediated reactions is well established using rhodium,50 their implementation as ligands on palladium is less widely reported.51
By using (1R,4R)2,5-diphenylbicyclo[2.2.2]octa-2,5-diene (R,R-DPBCO), possessing C2 rotational symmetry, as a ligand in the preparation of the Itoh catalysts, the intermediate prior to reductive elimination to give the coupled product is chiral, and should favor formation of one enantiomer based on the sterics of the ligand. While the yield of coupled product using Pd(R,R-DPBCO)(MAH) as a catalyst was notable at 78%, only a trace degree (>2%) of enantioenrichment was observed. This lack of enantioselectivity in the coupling reaction is likely due to the length of the coordinative bonds present in the intermediate, limiting the ability for steric directing to occur. Attempts at coupling more thoroughly functionalized allyl carbonates and phenyl siloxanes may provide a greater degree of enantioselectivity, given the higher incidence of steric interaction.
In conclusion, we have surveyed a novel set of 16-electron palladium(0) catalysts for the coupling of allyl carbonates and aryl siloxanes. Our studies have demonstrated that the reactivity of these catalysts can be "tuned" by two means: (1) the choice of diene ligand, which controls the cone angle of the alkene coordination, and (2) by adjusting the electronic characteristics of the electron-deficient alkene attached to the metal center. Attempts to employ these catalysts for enantioselective couplings were unsuccessful. Further studies into the utilization of these complexes in Pd-catalyzed coupling will be reported in due course.
EXPERIMENTAL
All reactions were run under an atmosphere of argon unless otherwise noted. Glassware used in the reactions was dried for a minimum of 12 h in an oven at 120 ºC. Tetrahydrofuran and diethyl ether were distilled from sodium / benzophenone ketyl, while methylene chloride, pyridine, and dimethylformamide were distilled from calcium hydride. Infrared spectra were recorded on a Nicolet 560 FT-IR spectrophotometer. Samples used for obtaining infrared spectra were either dissolved in carbon tetrachloride or taken neat. IR band positions are reported in reciprocal centimeters (cm-1) and relative intensities are listed as: br (broad), s (strong), m (medium), or w (weak). Nuclear magnetic resonance (1H, 13C, DEPT-135 NMR) spectra were recorded on a 400 or 500 MHz spectrometer. Chemical shifts are reported in parts per million (δ) and coupling (J values) are reported in hertz (Hz). Spin multiplicities are indicated by the following symbols: s (singlet), d (doublet), t (triplet), q (quartet), m (multiplet), br s (broad singlet) and br d (broad doublet). Low-resolution mass spectrometry (LRMS) and high-resolution mass spectrometry (HRMS) were obtained on a JEOL SX-02A instrument.
Cyclohexenyl Carbonate (4): To 2.09 g (21.3 mmol, 1.00 equiv.) of commercially available 2-cyclohexen-1-ol, in 20.0 mL anhydrous CH2Cl2 and 2.57 mL (31.9 mmol, 1.50 equiv.) anhydrous pyridine, was added 3.17 mL (31.9 mmol, 1.50 equiv.) of ethyl chloroformate dropwise via syringe under argon. The reaction was allowed to stir at room temperature for 7 days. The reaction mixture was extracted with CH2Cl2 (3 × 50 mL), washed with H2O (50 mL), dried over MgSO4 and concentrated in vacuo. Flash chromatography on silica gel (5% EtOAc/95% hexane, Rf = 0.51) afforded 2.32 g (64%) of the cyclohexenyl carbonate 4 as a colorless oil; IR (CCl4) 3042 (w), 2981 (w), 2947 (m), 2875 (w), 2838 (w), 1737 (s), 1373 (s), 1265 (s), 1017 (s) cm-1; 1H NMR (400 MHz, CDCl3) δ 5.97-5.93 (m, 1H), 5.76- 5.73 (m, 1H), 5.10-5.08 (m, 1H), 4.16 (q, J = 7 Hz, 2H), 2.09-2.04 (m, 1H), 1.99-1.84 (m, 1H), 1.83-1.72 (m, 3H), 1.63-1.60 (m, 1H), 1.28 (t, J = 7 Hz, 3H); 13C NMR (100 MHz, CDCl3) δ 154.9, 133.2, 125.1, 71.6, 63.7, 28.3, 24.9, 18.6, 14.3.
General Pd(0) Catalyst Preparation (5): To 50.0 mg (0.0483 mmol, 1 equiv.) of Pd2dba3•CHCl3 in 1.5 mL of anhydrous acetone was added 134.0 mg (1.449 mmol, 30 equiv.) of norbornadiene and 27.0 mg (0.242 mmol, 5 equiv.) of N-methylmaleimide under an atmosphere of argon. The reaction mixture was stirred for 30 min at room temperature, upon which noticeable palladium black had accumulated in the reaction vessel. The reaction mixture was transferred via cannula and filtered under argon to provide a translucent yellow-green solution. The solution was briefly concentrated in vacuo to provide a more viscous, yellow-green oil, to which 5.0 mL of anhydrous Et2O was added. This provided an opaque, yellow-green suspension of Pd(NBD)(NMM) catalyst as a fine yellow powder, which was used immediately in the coupling reaction, to avoid degradation.
3-Phenylcyclohex-1-ene (6): To 30.0 mg (0.0969 mmol, 0.25 equiv.) of Pd(NBD)(NMM) catalyst from the formation reaction above, under an atmosphere of argon, was added 65.6 mg (0.388 mmol, 1.00 equiv.) of cyclohexenyl carbonate 4 and 186 mg (0.775 mmol, 2.00 equiv.) of aryl siloxane 5 in 5.00 mL of anhydrous THF. This was followed by addition of 0.775 mL (0.775 mmol, 2.00 equiv.) of 1 M TBAF solution in THF, and the reaction mixture was stirred at 55 ºC for 24 h. The product was extracted with Et2O (5 × 25 mL) and washed with 25 mL H2O. The combined organic layers were dried over MgSO4 and concentrated in vacuo to give crude 3-phenylcyclohex-1-ene 6. Purification by column chromatography (100% pentane; Rf = 0.65) rendered pure 3-phenylcyclohex-1-ene 6 as a colorless oil; IR (CCl4) 3025 (s), 2934 (s), 2858 (m), 2838 (m), 1601 (w), 1492 (m), 1452 (m) cm-1; 1H NMR (400 MHz, d6-Benzene) δ 7.20-7.17 (m, 4H), 7.11-7.07 (m, 1H), 5.82-5.73 (m, 2H), 3.32-3.27 (m, 1H), 1.92-1.89 (m, 3H), 1.56-1.47 (m, 3H); 13C NMR (125 MHz, benzene-d6) δ 147.2, 131.0, 129.0, 128.8, 128.7, 128.5, 128.3, 126.7, 42.6, 33.4, 25.7, 21.8.
ACKNOWLEDGEMENTS
The generous financial support of the National Institutes of Health, National Cancer Institute (CA 82169), and the University of Maryland is acknowledged. We thank Dr. Yiu-fai Lam and Dr. Yue Li (University of Maryland) for their assistance obtaining NMR and mass spectral data, respectively. The support of the National Science Foundation for the purchase of NMR instrumentation (CHE0615049) is acknowledged.
References
1. T. Hiyama, J. Org. Chem., 1988, 53, 918. CrossRef
2. T. Hiyama and E. Shirakawa, Top. Curr. Chem., 2002, 219, 61. CrossRef
3. T. Hiyama and Y. Hatanaka, Pure Appl. Chem., 1994, 66, 1471. CrossRef
4. T. Hiyama, J. Organomet. Chem., 2002, 653, 58. CrossRef
5. K. H. Shukla and P. DeShong, Heterocycles, 2012, 86, 1055. CrossRef
6. G. Pettit, V. Gaddamidi, and G. Cragg, J. Chem. Soc., Chem. Commun., 1984, 1693. CrossRef
7. G. Pettit, V. Gaddamidi, D. Herald, S. Singh, G. Cragg, J. Schmidt, F. Boettner, M. Williams, and Y. Sagawa, J. Nat. Prod., 1986, 49, 995. CrossRef
8. B. Gabrielsen, T. P. Monath, J. W. Huggins, D. F. Kefauver, G. R. Pettit, G. Groszek, M. Hollingshead, J. J. Kirsi, W. M. Shannon, and E. M. Schubert, J. Nat. Prod., 1992, 55, 1569. CrossRef
9. S. Ghosal, S. Singh, Y. Kumar, and R. Srivastava, Phytochemistry, 1989, 28, 611. CrossRef
10. B. Trost and S. Pulley, J. Am. Chem. Soc., 1995, 117, 10143. CrossRef
11. T. Hudlicky, X. Tian, K. Konigsberger, R. Maurya, J. Rouden, and B. Fan, J. Am. Chem. Soc., 1996, 118, 10752. CrossRef
12. P. Magnus and I. Sebhat, Tetrahedron, 1998, 54, 15509. CrossRef
13. J. Rigby, U. Maharoof, and M. Mateo, J. Am. Chem. Soc., 2000, 122, 6624. CrossRef
14. H. Ko, E. Kim, J. E. Park, D. Kim, and S. Kim, J. Org. Chem., 2004, 69, 112. CrossRef
15. J. L. Aceña, O. Arjona, M. L. León, and J. Plumet, Org. Lett., 2000, 2, 3683. CrossRef
16. T. Doyle, M. Hendrix, D. VanDerveer, S. Javanmard, and J. Haseltine, Tetrahedron, 1997, 53, 11153. CrossRef
17. N. Chida, M. Jitsuoka, Y. Yamamoto, M. Ohtsuka, and S. Ogawa, Heterocycles, 1996, 43, 1385. CrossRef
18. S. Danishefsky and J. Y. Lee, J. Am. Chem. Soc., 1989, 111, 4829. CrossRef
19. G. R. Pettit, N. Melody, and D. L. Herald, J. Org. Chem., 2001, 66, 2583. CrossRef
20. A. Wu and P. Zhou, Tetrahedron Lett., 2006. 47, 3707. CrossRef
21. A. Padwa and H. Zhang, J. Org. Chem., 2007, 72, 2570. CrossRef
22. A. E. Håkansson, A. Palmelund, H. Holm, and R. Madsen, Chem. Eur. J., 2006, 12, 3243. CrossRef
23. J. H. Dam and R. Madsen, Eur. J. Org. Chem., 2009, 4666. CrossRef
24. G. Keck, S. McHardy, and J. Murry, J. Org. Chem., 1999, 64, 4465. CrossRef
25. U. Rinner and T. Hudlicky, Synlett, 2005, 365. CrossRef
26. Y. Chapleur, F. Chretien, S. Ibn Ahmed, and M. Khaldi, Curr. Org. Synth., 2006, 3, 341. CrossRef
27. T. Hudlicky, ARKIVOC, 2006, vii, 276.
28. M. Manpadi and A. Kornienko, Org. Prep. Proced. Int, 2008, 40, 107. CrossRef
29. A. Kornienko and A. Evidente, Chem. Rev., 2008, 108, 1982. CrossRef
30. J. Collins, U. Rinner, M. Moser, T. Hudlicky, I. Ghiviriga, A. E. Romero, A. Kornienko, D. Ma, C. Griffin, and S. Pandey, J. Org. Chem., 2010, 75, 3069. CrossRef
31. S. E. Denmark and N. S. Werner, J. Am. Chem. Soc., 2008, 130, 16382. CrossRef
32. K. H. Shukla and P. DeShong, J. Org. Chem., 2008, 73, 6283. CrossRef
33. K. Itoh, F. Ueda, K. Hirai, and Y. Ishii, Chem. Lett., 1977, 877. CrossRef
34. M. Hiramatsu, K. Shiozaki, T. Fujinami, and S. Sakai, J. Organomet. Chem., 1983, 246, 203. CrossRef
35. Y. Yamamoto, T. Ohno, and K. Itoh, Organometallics, 2003, 22, 2267; CrossRef K. Itoh, K. Hirai, M. Sasaki, Y. Nakamura, and H. Nishiyama, Chem. Lett., 1981, 865. CrossRef
36. M. Green, J. Howard, J. Spencer, and F. G. A. Stone, J. Chem. Soc., Chem. Commun., 1975, 449. CrossRef
37. M. Green, J. Howard, J. Spencer, and F. G. A. Stone, J. Chem. Soc., Dalton Trans., 1977, 271. CrossRef
38. J. Krause, G. Cestaric, K.-J. Haack, K. Seevogel, W. Storm, and K.-R. Pörschke, J. Am. Chem. Soc., 1999, 121, 9807. CrossRef
39. M. Schwalbe, D. Walther, H. Schreer, J. Langer, and H. Goerls, J. Organomet. Chem., 2006, 691, 4868. CrossRef
40. A. M. Kluwer, C. J. Elsevier, M. Bühl, M. Lutz, and A. L. Spek, Angew. Chem. Int. Ed., 2003, 42, 3501. CrossRef
41. M. Grundl, J. Kennedy-Smith, and D. Trauner, Organometallics, 2005, 24, 2831. CrossRef
42. J. Masllorens, A. Pla-Quintana, T. Parella, and A. Roglans, ARKIVOC, 2010, ii, 203.
43. L. Canovese and F. Visentin, Inorg. Chim. Acta, 2010, 363, 2375. CrossRef
44. K. Blum, E. S. Chernyshova, R. Goddard, K. Jonas, and K.-R. Pörschke, Organometallics, 2007, 26, 5174. CrossRef
45. M. Brescia and P. DeShong, J. Org. Chem., 1998, 63, 3156. CrossRef
46. M. Mowery and P. DeShong, J. Org. Chem., 1999, 64, 1684. CrossRef
47. R. Correia and P. DeShong, J. Org. Chem., 2001, 66, 7159. CrossRef
48. J. B. Johnson and T. Rovis, Angew. Chem. Int. Ed., 2008, 47, 840. CrossRef
49. I. J. S. Fairlamb, Org. Biomol. Chem., 2008, 6, 3645. CrossRef
50. H. Grützmacher and E. M. Carreira, Angew. Chem. Int. Ed., 2008, 47, 4482. CrossRef
51. S.-S. Zhang, Z.-Q. Wang, M.-H. Xu, and G.-Q. Lin, Org. Lett., 2010, 12, 5546. CrossRef