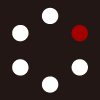
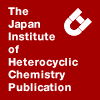
HETEROCYCLES
An International Journal for Reviews and Communications in Heterocyclic ChemistryWeb Edition ISSN: 1881-0942
Published online by The Japan Institute of Heterocyclic Chemistry
e-Journal
Full Text HTML
Received, 17th June, 2013, Accepted, 12th July, 2013, Published online, 19th July, 2013.
DOI: 10.3987/COM-13-S(S)33
■ The Ability of 1-Aryltriazole-Containing Nucleobases to Recognize a TA Base Pair in Triplex DNA
Yoshiyuki Hari,* Motoi Nakahara, Shin Ijitsu, and Satoshi Obika*
Graduate School of Pharmaceutical Science, Osaka University, 1-6 Yamadaoka, Suita, Osaka 560-0871, Japan
Abstract
Phosphoramidites bearing propargyl and (N-propargylcarbamoyl)methyl groups at the C1-position of deoxyribose were synthesized and introduced into oligonucleotides by using an automated DNA synthesizer. Copper-catalyzed alkyne-azide 1,3-dipolar cycloaddition of the oligonucleotides with various aryl azides led to triplex-forming oligonucleotides (TFOs) possessing the corresponding aryltriazole-containing nucleobases. The triplex-forming ability of TFOs with double-stranded DNA (dsDNA) was evaluated through UV-melting experiments, and it was demonstrated that m-hydroxy or m-ureido derivatives in the (1-aryltriazol-4-yl)methyl nucleobases likely interacted with a TA base pair in dsDNA.INTRODUCTION
Triplex formation of double-stranded DNA (dsDNA) by an oligonucleotide (TFO: triplex-forming oligonucleotide) is applicable in various dsDNA-targeting technologies. In triplex formation, TFOs consisting of pyrimidine sequences can sequence-selectively and stably recognize dsDNA via Hoogsteen hydrogen bond formation with AT and GC base pairs in dsDNA by T and C in TFO, respectively. Since no natural nucleic acid specifically recognizes a CG or TA base pair in dsDNA, many studies have been conducted to develop artificial nucleic acids capable of recognizing these base pairs.1-4 However, targeting of a TA base pair is difficult because access to the 4-carbonyl oxygen in T is sterically hindered by the 5-methyl group in T. Therefore, reports on the development of artificial nucleic acids recognizing a TA base pair are scarce.2-4
We considered that facile and efficient syntheses of various derivatives of a nucleobase structure were necessary for implementing detailed and rational designs of nucleobases for CG or TA base pair recognition, and we have used post-elongation modification (PEM) methods, namely, modification methods after oligonucleotide synthesis, to synthesize derivatives.2,5,6 We used a copper-catalyzed alkyne-azide cycloaddition (CuAAC) reaction7 as a PEM method and evaluated the various derivatives synthesized; 2-(1-m-carbonylaminophenyl-1,2,3-triazol-4-yl)ethyl nucleobases were recently predicted to interact with the A base of a TA base pair (Figure 1a).2 In addition, replacement of the ethylene unit by a methylenoxy unit led to a significant decrease in the binding affinity to a TA base pair. This may be due to the high flexibility of the methyleneoxy unit. Thus, based on unit length and suppression of the unit flexibility, nucleobases possessing a methylene unit would be of interest (Figure 1a). However, Guianvarc’h et al. reported the nucleobase S, which is the N-H group in the amide moiety thought to interact with the 4-carbonyl group in T of a TA base pair (Figure 1b).3 Therefore, nucleobases possessing an amide unit may also be effective as a scaffold for screening nucleobases to recognize a TA base pair (Figure 1b).
Based on previous studies, we synthesized TFOs containing various derivatives of two types of nucleobases, as shown in Figure 1. The TA base pair-recognition ability of the nucleobases was examined by UV melting experiments of triplexes formed with dsDNA.
RESULTS AND DISCUSSION
As shown in Scheme 1, phosphoramidites 1 and 2 possessing ethynyl units to be converted into desired nucleobases after oligonucleotide synthesis were synthesized. Reduction of 38 with DIBAL followed by treatment with Ohira-Bestmann reagent, (MeO)2POC(N2)COMe,9 afforded 4 in 82% yield over 2 steps, which was phosphitylated to give the desired 1. For synthesis of 2, hydrolysis of 3 under alkaline conditions and condensation with propargylamine in the presence of EDC•HCl and DMAP furnished 5 in 89% yield over 2 steps. The desired phosphoramidite 2 was prepared in 90% yield in the same procedure as 1.
Introduction of the synthesized phosphoramidites 1 and 2 into oligonucleotides was performed using an automated DNA synthesizer under conditions of general phosphoramidite chemistry; singly-modified oligonucleotides 6 and 7 as substrates of CuAAC reactions for PEM were successfully synthesized. Through the CuAAC reactions of 6 and 7 with aryl azides10 under optimized conditions [CuSO4, sodium ascorbate, tris[(1-benzyl-1H-1,2,3-triazol-4-yl)methyl]amine (TBTA)11 and azides in 30% DMSO-phosphate buffer (pH 7.0)],12 TFOs 8a–f and 9a–f bearing the corresponding aryltriazole moieties were obtained, respectively. The purity and molecular weight of all oligonucleotides synthesized were confirmed by reversed-phase HPLC and MALDI-TOF-MS, respectively.
The ability of non-natural nucleobases to recognize a TA base pair was evaluated by UV melting experiments of triplexes formed from TFOs 8 and 9 with a dsDNA target, and the results were compared with those of TFO 10 reported in our previous study.2 The obtained Tm values and the difference (ΔTm) in the Tm values of TFOs possessing any substitution (b–f) on the benzene ring from those of unsubstituted congeners (a) are summarized in Figure 2. TFO 8a possessing an unsubstituted phenyl group showed a Tm value of 17 °C, which was drastically lower than that (22 °C) of TFO 10a. The (1-phenyltriazol-4-yl)methyl unit in TFO 8a may be spatially incompatible with this dsDNA target in triplex formation because these nucleobases in TFOs 8a and 10a likely have no positive interaction with a TA base pair. Based on the results of TFOs 8a–c, the decrease in Tm values was not observed for the o-substituent, unlike TFOs 10a–c. In contrast, m-substituents (8d–f) generally increased in the binding affinity to a TA base pair, and hydroxy (8d) and ureido (8f) groups at the m-position led to significantly increased Tm values of +6 °C and +5 °C compared with unsubstituted 8a, respectively. These results suggest that these analogs positively interact with the TA base pair, for example, via formation of a hydrogen bond with A of a TA base pair. In contrast to the significant stabilization by 8d, 10d containing the same m-hydroxy group did not stabilize the triplex at all, which is of interest, and further investigation involving a computational study will be required to clarify the reason for this observation. For TFO 8 bearing (1-aryltriazol-4-yl)methyl nucleobases, remarkable changes in Tm values were observed, which were thought to be caused by the lower flexibility of the methylene unit, as expected (Figure 1a). However, the Tm value of TFO 9a possessing an unsubstituted phenyl group was higher than that of 8a, but nearly the same as that of 10a. This result implies that the amide N-H in 9a does not recognize the T of a TA base pair, unlike the S found by Sun’s group3 (Figure 1b). TFOs 9b–c with o-substituents showed slightly decreased affinity to the TA base pair, while 9d–f with m-substituents showed increased Tm values of +2 °C compared with that of 9a. However, changes of Tm values were globally low, which may have been caused by the high flexibility of the four-atom length-chain, -CH2CONHCH2-.
In conclusion, two phosphoramidites with ethynyl units were synthesized and introduced into the oligonucleotides. Moreover, through CuAAC reactions of the oligonucleotides, the facile synthesis of TFOs bearing 1-aryltriazole-containing nucleobases was fulfilled. UV melting experiments of synthesized TFOs demonstrated that [1-(m-hydroxy- or 1-m-ureido-phenyl)triazol-4-yl]methyl nucleobases likely interacted with a TA base pair. Based on this finding and our previous results, the appropriate spacer between aryltriazole and deoxyribose moieties is likely of one- or two-atom length and less flexible. In future studies, we will structurally optimize phenyltriazole and spacer moieties to set the functional group at a suitable position to recognize the TA base pair.
EXPERIMENTAL
All moisture-sensitive reactions were carried out in thoroughly dried glassware under a nitrogen atmosphere. 1H, 13C and 31P spectra were recorded on a JEOL JNM-AL300 or JEOL JNM-EX400 spectrometer. Chemical shifts are reported in parts per million referenced to tetramethylsilane (δ = 0.00 ppm) for 1H NMR spectra, CDCl3 (δ = 77.0 ppm) and CD3OD (δ = 49.0 ppm) for 13C NMR spectra, and phosphoric acid (δ = 0.00 ppm) for 31P NMR spectra. IR spectra were recorded on a JASCO FT/IR-4200 spectrometer. Specific rotations were recorded on a JASCO P-2200 polarimeter. EI and FAB mass spectra were measured on a JEOL JMS-600 or JEOL JMS-700 mass spectrometer. MALDI-TOF mass spectra were recorded on a Bruker Daltonics Autoflex II TOF/TOF or JEOL JMS-S3000 mass spectrometer. Fuji Silysia silica gel PSQ-60B (0.060 mm) and FL-60D (0.060 mm) were used for flash column chromatography. For HPLC, SHIMADZU LC-10ATVP, SHIMADZU SPD-10AVP and SHIMADZU CTO-10VP instruments were used. EYELA Cute Mixer CM-1000 was used as a shaker.
1,2-Dideoxy-5-O-(4,4’-dimethoxytrityl)-1-(prop-2-ynyl)-β-D-ribofuranose (4)
Under a nitrogen atmosphere, DIBAL (5.1 mL, 4.74 mmol) was added to a solution of 38 (1.2 g, 2.37 mmol) in anhydrous CH2Cl2 (10 mL) at -78 °C, and the mixture was stirred for 0.5 h. After addition of saturated NaHCO3 aq., the mixture was extracted with CH2Cl2. The organic extracts were washed with brine, dried over Na2SO4, and concentrated under reduced pressure. The residue was purified by flash silica gel column chromatography (n-hexane/AcOEt = 1:2) to give the aldehyde (1.0 g, 92%). This compound was not subjected to further purification and a portion of this was used in the next step. Under a nitrogen atmosphere, (MeO)2POC(N2)COMe (250 mg, 1.30 mmol) and K2CO3 (450 mg, 3.24 mmol) were added to a solution of aldehyde (500 mg, 1.08 mmol) in anhydrous MeOH (10 mL) at rt. After being stirred for 5 h, the mixture was concentrated under reduced pressure. The residue was extracted with AcOEt. The organic extracts were washed with water and brine, dried over Na2SO4, and concentrated under reduced pressure. The residue was purified by flash silica gel column chromatography (n-hexane/AcOEt = 2:1) to give compound 4 (420 mg, 82% for 2 steps) as a colorless oil. [α]D24 +8.4 (c 1.06, CHCl3); IR νmax (KBr) 2932, 1607, 1509, 1462, 1444, 1301, 1251, 1177, 1085, 1035 cm-1; 1H-NMR (CDCl3) δ 1.92–2.02 (3H, m), 2.43–2.49 (2H, m), 3.09 (1H, dd, J = 6.0, 10.0 Hz), 3.24 (1H, dd, J = 4.5, 10.0 Hz), 3.77 (6H, s), 3.93–3.94 (1H, m), 4.29–4.34 (2H, m), 6.81–6.83 (4H, m), 7.22–7.44 (9H, m); 13C-NMR (CDCl3) δ 25.0, 39.7, 55.1, 64.5, 70.0, 74.4, 76.3, 80.2, 85.9, 86.1, 113.0, 126.7, 127.7, 128.1, 130.0, 136.0, 144.8, 158.4; MS (EI) m/z 458 (M+, 100); HRMS (EI) m/z Calcd for C29H30O5: 458.2093. Found 458.2089.
3-O-[2-Cyanoethoxy(diisopropylamino)phosphino]-1,2-dideoxy-5-O-(4,4’-dimethoxytrityl)-1-(prop-2-ynyl)-β-D-ribofuranose (1)
Under a nitrogen atmosphere, 2-cyanoethyl-N,N-diisopropylchlorophosphoramidite (120 μL, 0.539 mmol) was added to a solution of compound 4 (190 mg, 0.414 mmol) and N,N-diisopropylethylamine (210 μL, 0.539 mmol) in anhydrous CH2Cl2 (2 mL) at 0 °C, and the mixture was stirred at rt for 1.5 h. After addition of water, the solvent was removed under reduced pressure and the residue was purified by flash silica gel column chromatography (n-hexane/AcOEt = 5:1) to give compound 1 (237 mg, 87%) as a colorless syrup. 1H NMR (CDCl3) δ 1.08 (3H, d, J = 7.0 Hz), 1.13–1.18 (9H, m), 1.91–1.96 (2H, m), 2.06–2.21 (1H, m), 2,43–2.46 (1H, m), 2.50–2.55 (2H, m), 2.60 (1H, t, J = 6.5 Hz) 3.11–3.16 (2H, m), 3.52–3.81 (10H, m), 4.10–4.15 (1H, m), 4.30–4.33 (1H, m), 4.44–4.48 (1H, m), 6.79–6.83 (4H, m), 7.19–7.36 (7H, m), 7.44–7.47 (2H, m); 31P-NMR (CDCl3) δ 147.1, 147.7; MS (FAB) m/z 681 [M+Na]+; HRMS (FAB) m/z Calcd for C38H47N2O6P [M+Na]+: 681.3069. Found 681.3093.
2-[1,2-Dideoxy-5-O-(4,4’-dimethoxytrityl)-β-D-ribofuranos-1-yl]-N-(prop-2-ynyl)ethanamide (5)
KOH (220 mg, 3.95 mmol) was added to a solution of 38 (1.0 g, 1.97 mmol) in THF/H2O (1:1, 10 mL) at 0 °C and the mixture was stirred at rt for 12 h. After addition of 2N HCl aq., the mixture was extracted with AcOEt. The organic extracts were washed with brine, dried over Na2SO4, and concentrated under reduced pressure. The residue was purified by flash silica gel column chromatography (n-hexane/AcOEt = 1:6) to give carboxylic acid (970 mg, 96%). This compound was not subjected to further purification and a portion of this was used in the next step. Under a nitrogen atmosphere, propargylamine (27 μL, 0.426 mmol), EDC·HCl (82 mg, 0.426 mmol) and DMAP (9 mg, 0.0711 mmol) were added to a solution of carboxylic acid (170 mg, 0.355 mmol) in anhydrous CH2Cl2 (3 mL) at rt and the mixture was stirred for 11 h. After addition of water, the mixture was extracted with CH2Cl2. The organic extracts were washed with water and brine, dried over Na2SO4, and concentrated under reduced pressure. The residue was purified by flash silica gel column chromatography (n-hexane/AcOEt = 1:3) to give compound 5 (170 mg, 89% for 2 steps) as a colorless syrup. [α]D22 -13.8 (c 1.12, CHCl3); IR nmax (KBr) 3288, 2931, 1656, 1607, 1509, 1444, 1300, 1251, 1082, 1034 cm-1; 1H-NMR (CDCl3) δ 1.72 (1H, d, J = 3.5 Hz), 1.83 (1H, ddd, J = 5.5, 9.5, 13.0 Hz), 2.00 (1H, ddd, J = 2.0, 4.0, 13.0 Hz), 2.13 (1H, t, J = 2.5 Hz), 2.44 (1H, dd, J = 8.5, 15.5 Hz), 2.55 (1H, dd, J = 3.0, 15.5 Hz), 3.16 (1H, dd, J = 5.5, 10.0 Hz), 3.24 (1H, dd, J = 5.0, 10.0 Hz), 3.80 (6H, s), 3.98 (1H, ddd, J = 2.5, 5.0, 5.0 Hz), 4.31–4.36 (1H, m), 4.41–4.51 (1H, m), 6.70 (1H, brs), 6.81–6.86 (4H, m), 7.22–7.44 (9H, m); 13C-NMR (CDCl3) δ 28.9, 40.7, 42.0, 55.2, 64.3, 71.3, 73.8, 74.7, 79.6, 86.2, 86.4, 113.1, 126.8, 127.8, 128.1, 130.0, 135.8, 144.7, 158.5, 170.5; MS (EI) m/z 515 (M+, 100); HRMS (EI) m/z Calcd for C31H33NO6: 515.2308. Found 515.2307.
2-{3-O-[2-Cyanoethoxy(diisopropylamino)phosphino]-1,2-dideoxy-5-O-(4,4’-dimethoxytrityl)-β-D-ribofuranos-1-yl}-N-(prop-2-ynyl)ethanamide (2)
Under a nitrogen atmosphere, 2-cyanoethyl-N,N-diisopropylchlorophosphoramidite (52 μL, 0.233 mmol) was added to a solution of compound 5 (100 mg, 0.194 mmol) and N,N-diisopropylethylamine (100 μL, 0.582 mmol) in anhydrous CH2Cl2 (2 mL) at 0 °C, and the mixture was stirred at rt for 3 h. After addition of saturated NaHCO3 aq., the solvent was removed under reduced pressure and the residue was purified by flash silica gel column chromatography (n-hexane/AcOEt = 1:1) to give compound 2 (125 mg, 90%) as a colorless syrup. 1H NMR (CDCl3) δ 1.08 (4H, d, J = 7.0 Hz), 1.12–1.18 (8H, m), 1.75–1.84 (1H, m), 2.05–2.21 (2H, m), 2.40–2.48 (2H, m), 2.56–2.60 (2H, m), 3.14–3.20 (2H, m), 3.51–3.88 (10H, m), 3.95–4.00 (2H, m), 4.10–4.13 (1H, m), 4.40–4.45 (2H, m), 6.68 (1H, brs), 6.81–6.85 (4H, m), 7.20–7.34 (7H, m), 7.42–7.45 (2H, m); 31P-NMR (CDCl3) δ 147.7, 148.2; HRMS (MALDI-TOF) m/z Calcd for C40H50N3NaO7P [M+Na]+: 738.3279. Found 738.3297.
Synthesis of oligonucleotides
The syntheses of 6 and 7 were performed on a 0.2-µmol scale or 1.0-µmol scale on an automated DNA synthesizer (Gene Design nS-8) using the common phosphoramidite protocol. TFOs synthesized on DMTr-ON mode were cleaved from the CPG resin by treatment with 28% NH3 aq. at rt for 1.5 h and all the protecting groups on TFOs were removed by treatment with 28% NH3 aq. at 55 °C for 12 h. The obtained crude TFOs were purified on Sep-Pak® Plus C18 cartridges (Waters) followed by reversed-phase HPLC (Waters XBridge® OST C18 column 2.5 µm, 10 mm × 50 mm). The composition of the TFOs was confirmed by MALDI-TOF-MS analysis. MALDI-TOF-MS data ([M-H]–) for 6 and 7: 6, found 4409.26 (calcd. 4409.93). 7, found 4467.01 (calcd. 4466.99).
Click chemistry: General procedure
A solution of azide compound (5 mM in DMSO, 8 µL) was added to a mixture of CuSO4 (2 mM in H2O, 4 µL), TBTA (2 mM in DMSO, 4 µL), sodium ascorbate (10 mM in H2O, 4 µL), 6 or 7 [0.2 mM in phosphate buffer (pH 7.0), 20 µL] in a 1.5-mL Eppendorf tube. The mixture was shaken at rt using a shaker (1000 rpm) until the reaction was complete. The entire product was purified by reversed-phase HPLC [column: Waters XBridge® OST C18 column 2.5 µm, 4.6 mm × 50 mm; eluent: gradient system of MeCN/0.1 M triethylammonium acetate buffer (pH 7.0); flow rate: 1.0 mL/min] to give the desired TFO 8 or 9. Yields and MALDI-TOF-MS data ([M-H]–) for TFOs 8a-f and 9a-f: 8a, 77% yield, found 4529.21 (calcd. 4529.06); 8b, 71% yield, found 4545.93 (calcd. 4545.01); 8c, 65% yield, found 4571.01 (calcd. 4572.03); 8d, 78% yield, found 4545.11 (calcd. 4545.01); 8e, 84% yield, found 4572.12 (calcd. 4572.03); 8f, 71% yield, found 4588.02 (calcd. 4587.05); 9a, 75% yield, found 4586.41 (calcd. 4586.11); 9b, 73% yield, found 4630.72 (calcd. 4630.12); 9c, 74% yield, found 4628.68 (calcd. 4629.14); 9d, 81% yield, found 4602.54 (calcd. 4602.11); 9e, 80% yield, found 4629.49 (calcd. 4629.14); 9f, 89% yield, found 4644.31 (calcd. 4644.15).
UV-Melting experiments
UV-Melting experiments were carried out on SHIMADZU UV-1650 and SHIMADZU UV-1800 spectrometers equipped with Tm analysis accessory. Equimolecular amounts of the target hairpin-loop dsDNA and TFOs were dissolved in 10 mM sodium cacodylate buffer (pH 6.8) containing 100 mM KCl and 50 mM MgCl2 to give a final strand concentration of 1.9 µM. The samples were annealed by heating at 100 °C followed by slow cooling to 5 °C. The melting profiles were recorded at 260 nm from 5 °C to 90 °C at a scan rate of 0.5 °C/min.
ACKNOWLEDGEMENTS
This work was supported in part by a Grand-in-Aid for Scientific Research on Innovative Areas (No. 22136006) from the Ministry of Education, Culture, Sports, Science and Technology, Japan (MEXT), a Grant-in-Aid for JSPS Fellows of Japan Society for the Promotion of Science (JSPS) and the Mochida Memorial Foundation for Medical and Pharmaceutical Research.
References
1. Reviews: S. O. Doronina and J.-P. Behr, Chem. Soc. Rev., 1997, 63; I. Luyten and P. Herdewijn, Eur. J. Med. Chem., 1998, 33, 515; CrossRef D. M. Gowers and K. R. Fox, Nucleic Acids Res., 1999, 27, 1569; CrossRef K. R. Fox, Curr. Med. Chem., 2000, 7, 17; CrossRef J. Robles, A. Grandas, E. Pedroso, F. J. Luque, R. Eritja, and M. Orozco, Curr. Org. Chem., 2002, 6, 1333; CrossRef M. G. M. Purwanto and K. Weisz, Curr. Org. Chem., 2003, 7, 427; CrossRef S. Buchini and C. J. Leumann, Curr. Opin. Chem. Biol., 2003, 7, 717; CrossRef K. R. Fox and T. Brown, Quart. Rev. Biophys., 2005, 38, 311; CrossRef D. A. Rusling, V. J. Broughton-Head, T. Brown, and K. R. Fox, Curr. Chem. Biol., 2008, 2, 1; V. Malnuit, M. Duca, and R. Benhida, Org. Biomol. Chem., 2011, 9, 326; CrossRef K. R. Fox and T. Brown, Biochem. Soc. Trans., 2011, 39, 629; CrossRef Y. Hari, S. Obika, and T. Imanishi, Eur. J. Org. Chem., 2012, 2875. CrossRef
2. Y. Hari, M. Nakahara, and S. Obika, Bioorg. Med. Chem., 2013, 21, in press (doi: 10.1016/j.bmc.2013.05.034). CrossRef
3. D. Guianvarc’h, R. Benhida, J.-L. Fourrey, R. Maurisse, and J.-S. Sun, Chem. Commun., 2001, 1814; CrossRef D. Guianvarc’h, J.-L. Fourrey, R. Maurisse, J.-S. Sun, and R. Benhida, Bioorg. Med. Chem., 2003, 11, 2751. CrossRef
4. S. Verma and P. S. Miller, Bioconjugate Chem., 1996, 7, 600; CrossRef O. A. Amosova and J. R. Fresco, Nucleic Acids Res., 1999, 27, 4632; CrossRef D. Guianvarc’h, J.-L. Fourrey, R. Maurisse, J.-S. Sun, and R. Benhida, Org. Lett., 2002, 4, 4209; CrossRef Y. Wang, D. A. Rusling, V. E. C. Powers, O. Lack, S. D. Osborne, K. R. Fox, and T. Brown, Biochemistry, 2005, 44, 5884; CrossRef S. Buchini and C. J. Leumann, Eur. J. Org. Chem., 2006, 3152; CrossRef S. Obika, H. Inohara, Y. Hari, and T. Imanishi, Bioorg. Med. Chem., 2008, 16, 2945; CrossRef Y. Hari, S. Kashima, H. Inohara, S. Ijitsu, T. Imanishi, and S. Obika, Tetrahedron, 2013, 69, 6381. CrossRef
5. Y. Hari, M. Nakahara, J. Pang, M. Akabane, T. Kuboyama, and S. Obika, Bioorg. Med. Chem., 2011, 19, 1162. CrossRef
6. Y. Hari, M. Akabane, Y. Hatanaka, M. Nakahara, and S. Obika, Chem. Commun., 2011, 47, 4424; CrossRef Y. Hari, M. Akabane, and S. Obika, Chem. Commun., 2013, 49, in press (doi: 10.1039/c3cc44030c). CrossRef
7. Reviews: H. C. Kolb, M. G. Finn, and K. B. Sharpless, Angew. Chem. Int. Ed., 2001, 40, 2004; CrossRef H. C. Kolb and K. B. Sharpless, Drug Discov. Today, 2003, 8, 1128. CrossRef
8. J. Hovinen and H. Salo, J. Chem. Soc., Perkin Trans. 1, 1997, 3013.
9. S. Ohira, Synth. Commun., 1989, 19, 561; CrossRef S. Müller, B. Liepold, G. J. Roth, and H. J. Bestmann, Synlett, 1996, 521. CrossRef
10. All azides used in this study were prepared according to the previous reports.2,5,13.
11. . CrossRef
12. M. Nakahara, T. Kuboyama, A. Izawa, Y. Hari, T. Imanishi, and S. Obika, Bioorg. Med. Chem. Lett., 2009, 19, 3316. CrossRef
13. H. Tomioka, T. Matsushita, S. Murata, and S. Koseki, Liebigs Ann., 1996, 1971; J. Andersen, U. Madsen, F. Björkling, and X. Liang, Synlett, 2005, 2209. CrossRef