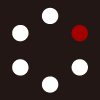
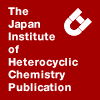
HETEROCYCLES
An International Journal for Reviews and Communications in Heterocyclic ChemistryWeb Edition ISSN: 1881-0942
Published online by The Japan Institute of Heterocyclic Chemistry
e-Journal
Full Text HTML
Received, 20th May, 2013, Accepted, 17th June, 2013, Published online, 24th June, 2013.
DOI: 10.3987/REV-13-773
■ Recent Advances in the Syntheses of Decalin Type Natural Products
Hiroyuki Akita*
Department of Pharmaceutical Sciences, Nihon Pharmaceutical University, 10281 Komuro, Ina-machi, Kitaadachi-gun, Saitama, 362-0806, Japan
Abstract
This review summarizes the practical synthesis of both enantiomers of chiral intermediate for the syntheses of the natural products possessing the bicyclo[4.4.0]ring system with 4,4,10-trimethyl groups and their application to the total syntheses of the related natural products. Chiral induction was carried out based on enzymatic resolution or optical resolution using chiral auxiliary.1. Introduction
Numerous syntheses of the natural products possessing the bicyclo[4.4.0]ring system with 4,4,10-trimethyl groups have reviewed within the last forty years and synthesis has been accomplished by the transformation of other natural products or by total syntheses.1,2 Chiral compounds possessing the bicyclo[4.4.0]ring system with 4,4,10-trimethyl groups are an important requisite found in a variety of natural products like drimane sesquiterpenoids and other related natural compounds. In these chiral compounds, there are many biologically active (10S)-type natural products such as (-)-warburganal (1) and (10R)-type natural products such as (+)-jolkinolide D (2) as shown in Scheme 1. The former type natural products could be synthesized from natural source such as l-abietic acid (3) or (+)-sclareolide (4), while the latter type natural products could be derived from natural source such as (-)-copalic acid (5). In the course of our studies on the chemical conversion of l-abietic acid (3) to optically active natural products,3 ozonolysis of phenolic compounds [11,12-dihydroxy dehydroabietane (7),4 ferruginol (8),4 13-hydroxy-13-deisopropyldehydroabietane (9),4 14-hydroxydehydroabietane (10),4 and 6α,14-dihydroxydehydroabietane (11)5] derived from dehydroabietic acid (6) followed by reduction or/and oxidation with the appropriate reagent afforded (+)-isodrimenin (12),5,6 pentanorlabdane type compound (13),5,6 (+)-pallescensin A (14),5,6 (+)-confertifolin (15),5,6 (+)-valdiviolide (16),5,6 (+)-winterin (17),5,6 (+)-fragrolide (18),5,7 and (+)-bemadienolide (19),5,7 respectively, as shown in Scheme 2.
The mode of cleavage was governed by the substitution pattern of the hydroxyl group on the aromatic ring. On the other hand, a large scale synthesis of (±)-12 and (±)-15 was achieved from the starting β-ionone (20) as shown in Scheme 3.8,9 The cyclization of the furanic ester 21 followed by saponification and decarboxylation afforded (±)-euryfuran (22). Further transformations of the furan ring provided the drimane sesquiterpenoids (±)-12, (±)-15, (±)-1,10,11 (±)-cinnamodial (23),12,13 and (±)-cinnamosmolide (24). 12,13 In this review, we describe the practical synthesis of both enantiomers of chiral intermediate for the syntheses of the natural products possessing the bicyclo[4.4.0]ring system with 4,4,10-trimethyl groups and their application to the total syntheses of the related compounds. Chiral induction was carried out based on enzymatic resolution or optical resolution using chiral auxiliary.
2. SYNTHESIS OF BOTH ENANTIOMERS OF CHIRAL SYNTHON AND THEIR APPLICATION TO THE SYNTHESES OF THE NATURAL PRODUCTS POSSESSING THE BICYCLO[4.4.0]RING SYSTEM WITH 4,4,10-TRIMETHYL GROUPS
2.1. Lipase assisted hydrolysis or esterification of the racemic methyl (1β,2α,4aα,8aβ)-2-hydroxydecahydro-5,5,8a-trimethylnaphthalene-1-carboxylate and its congener
Optically active title compound (29) was considered to be an important chiral intermediate for the synthesis of drimane type natural products and the racemic substrate for enzymatic resolution was synthesized from (±)-linalool (25) as shown in Scheme 4.14 Heating of 25 and ethyl vinyl ether in the presence of H3PO4 followed by acid treatment gave a mixture of aldehyde (26), which was reacted with Wittig reagent to afford the methyl Δ2 trans-dodecatrienoate (27). Biominetic cyclization of 27 in HCOOH-H2SO4 yielded the (±)-formate (28) and β-hydroxy ester (±)-29 in 30% and 8% overall yield from (±)-25, respectively. Saponification of (±)-28 gave quantitatively (±)-29, which was treated with Ac2O in pyridine to provide the acetate (±)-30. When the β-acyloxy esters (±)-28 and (±)-30 were exposed to the immobilized lipase OF-360 from Candida rugosa in a water-saturated organic solvent, the hydrolyzed product (8aS)-29 was obtained in moderate chemical (40~27%) and high optical (>99% ee) yields. On the other hand, enzymatic acetylation of (±)-29 and vinyl acetate in the presence of the same lipase gave 89% ee of (8aS)-30 in 9% yield as shown in Scheme 4. From this experiment, conversion rate using lipase was found to be late. Then enantioselective and regioselective acetylation of the (±)-1,3-diol (31) obtained from LiAlH4 reduction of (±)-29 was carried out as shown in Scheme 5. Exposure of (±)-31
with isopropenyl acetate in the presence of the lipase Godo E-4 from Pseudomonas sp. provided four kinds of optically active compounds (8aS)-32 (94% ee), (8aS)-33 (75% ee), (8aS)-34 (78% ee) and (8aR)-31 (89% ee) because (±)-31 has two reaction sites for acetylation.14 This problem could be overcome by converting two reaction sites to one reaction site in the substrate. This synthetic strategy could be realized by the formation of a phenolic acetal (±)-35 which corresponds to the masked 1,3-diol
structure. The newly introduced bulky naphthyl group in the substrate (±)-35 situated with the thermodynamically stable equatorial conformation. The reaction of (±)-31 and 2-hydroxy-1-naphthaldehyde gave the phenolic acetal (±)-35 as a single diastereomer in quantitative yield whose acetylation afforded the corresponding acetate (±)-36 in 99% yield as shown in Scheme 6.15 From a screening experiment using various kinds of lipase and acylase, a commercially available acylase I (No. A-2156) from Aspergillus melleus was found to be effective. When the phenolic acetate (±)-36 was
exposed to the acylase I in water-saturated diisopropyl ether, hydrolyzed product (8aR)-35 (47%, 80% ee) and unchanged (8aS)-36 (40%, 92% ee) were obtained. An enantiomeric excess of both materials was improved to give enantiomerically pure (8aR)-35 and (8aS)-36 by recrystallization, respectively. On the other hand, (±)-cis-1,3-diol (37) obtaind from oxidation of (±)-29 followed by LiAlH4 reduction was converted to the phenolic acetate (±)-39 by the same way as the preparation of (±)-36 as shown in Scheme 6.16 When the phenolic acetate (±)-39 was exposed to the acylase I in water-saturated diisopropyl ether, hydrolyzed product (8aS)-38 (50%, 56% ee) and unchanged (8aR)-36 (49%, 58% ee) were obtained. It was found that steric recognition by lipase was opposite between (±)-36 and (±)-39.
2.2. Synthesis of (-)-ambrox and (+)-galanolactone
(-)-Ambrox (48) is a perfume and synthesized from the present enzymatic product (8aS)-36 as shown in Scheme 7.15 A catalytic hydrogenation of (8aS)-36 followed by treatment with benzaldehyde in the presence of a catalytic amount of acid afforded an acetal (8aS)-40 exclusively in 98% yield. Reduction of
(8aS)-40 with a mixed reducing reagent [LiAlH4 (1 equiv.)-AlCl3 (4 equiv.)] provided selectively primary alcohol (8aS)-41 (93%), which was treated with CBr4 and Ph3P to give the bromide (8aS)-42 in 98% yield. A catalytic hydrogenation of (8aS)-42 followed by treatment with NaCN provided nitrile (8aS)-44 in 96% overall yield. Oxidation of (8aS)-44 with Jones reagent followed by acidic hydrolysis gave the β-keto acid (8aS)-13 in 85% overall yield. Reaction of (8aS)-13 with MeLi followed by treatment with p-TsOH provided the γ-lactone (8aS)-46 (65%). Reduction of (8aS)-46 with LiAlH4 yielded the diol (8aS)-47 (76%), which was treated with p-TsOH in MeNO2 provided the (-)-ambrox (48) in 45% yield. (+)-Galanolactone (53) is reported to exhibit anti-5HT (serotonin) effect and synthesized from the keto nitrile (8aS)-45 as shown in Scheme 7.17 Wittig olefination of (8aS)-45 with Ph3P=CH2 provided the exo-olefin (8aS)-49 (96%), which was reduced with Dibal-H to provide an aldehyde (8aS)-50 (92%). Coupling of (8aS)-50 with the anion of diethylphosphono-2-butyrolactone afforded the isomeric lactones (10S)-51 (Z-form, 26% yield) and (10S)-52 (E-form, 59% yield). Isomerization of (10S)-51 to (10S)-52 was effected by irradiation in the presence of diphenyl disulfide and 91% conversion yield of (10S)-52 was achieved. By applying the reported procedure,18 epoxidation of exo-olefin group of (10S)-52 with m-CPBA gave (+)-galanolactone (53) in 19% yield.
2.3. Formal synthesis of (+)-zonarol
A formal total synthesis of (+)-zonarol (59) from enzymatic product (8aR)-35 was then carried out as shown in Scheme 8.15 The (8aR)-35 was converted into the 1,3-bromohydrin (8aR)-43 in 88% overall yield in the same way as in the conversion of (8aS)-36 to (8aS)-43. Oxidation of (8aR)-43 gave the
bromo-ketone (8aR)-54 in 97% yield, which was treated with DBU to afford the α,β-unsaturated ketone (8aR)-55 in 86% yield. Michael addition of (8aR)-55 with the 2,5-dimethoxyphenyl Grignard reagent in the presence of CuI followed by treatment with Ac2O provided an enol acetate (10R)-56 in 82% yield, which was hydrolyzed to the ketone (10R)-57 in 85% yield. Wittig reaction of (10R)-57 with phosphonium salt in the presence of n-BuLi afforded the exo-olefin (10R)-58 (73% yield) whose spectral data were identical with those of the reported olefin (10R)-58.19 Total synthesis of (+)-zonarol (59) from (10R)-58 has been already achieved.19
3. SYNTHESIS OF BOTH ENANTIOMERS OF ALBICANOL AND THEIR APPLICATION TO THE SYNTHESES OF THEIR RELATED NATURAL PRODUCTS
3.1. Lipase assisted esterification of the racemic albicanol, drimenol and bicyclofarnesol
The substrates [(±)-albicanol (64), (±)-drimenol (65) and (±)-bicyclofarnesol (67)] for enzymatic reaction were synthesized from geranyl acetone (60) as shown in Scheme 9.20,21 The reaction of 60 and dimethyl carbonate in the presence of NaH gave the β-keto ester (61), which was subjected to biomimetic cyclization to afford the (±)-cyclic β-keto ester (62) in 42% overall yield from 60. Wittig olefination of (±)-62 followed by reduction gave (±)-64 in 90% overall yield from (±)-62. BF3·Et2O assisted isomerization of (±)-64 selectively gave (±)-65 in 93% yield. On the other hand, the reaction of (±)-62 with diethyl chlorophosphate followed by treatment with Me2CuLi yielded the α,β-unsaturated ester (±)-66, which was reduced with Dibal-H to afford (±)-67 in 74% overall yield from (±)-62. Then, enzymatic esterification of thus obtained (±)-64, (±)-65 and (±)-67 was carried out as shown in Scheme 10. Enzymatic acetylation of (±)-64 in the presence of isopropenyl acetate and lipase “PL-266” gave (8aR)-64 (>99% ee, 38% yield) and an acetate (8aS)-68 (67% ee, 57% yield). The 67% ee of (8aS)-68 was hydrolyzed followed by repeated acetylation to afford the optically pure (8aS)-68.20 This enzymatic esterification was improved by exchange of acylating reagent.21 Enzymatic esterification of (±)-64 in the presence of vinyl myristate and lipase “QL” gave (8aR)-64 (>99% ee, 43% yield) and the myristate (8aS)-69 (82% ee, 53% yield). The 82% ee of (8aS)-69 was hydrolyzed followed by repeated acylation to afford the optically pure (8aS)-69 in 74% overall yield. Hydrolysis of (8aS)-69 (>99% ee) with K2CO3 in MeOH gave the optically pure (8aS)-64 (98%). Enzymatic acetylation of (±)-65 in the presence of isopropenyl acetate and lipase “PL-266” gave (8aR)-65 (80% ee, 40% yield) and an acetate (8aS)-70 (61% ee, 53% yield). Enzymatic acetylation of (±)-67 in the presence of isopropenyl acetate and lipase “PL-266” gave (8aR)-67 (12% ee, 46% yield) and an acetate (8aS)-71 (12% ee, 43% yield). The results of enzymatic esterification of (±)-64, (±)-65 and (±)-67 were found to be subtly effected by the position of double bond in the substrate.
3.2. Syntheses of (-)-drimenin, (-)-drimenol, (-)-albicanyl 3,4-dihydroxycinnamate and formal synthesis of (-)-warburganal
(-)-Drimenin (73) was isolated from the stem bark of the South American Drimys species. Epoxidation of (+)-albicanol (64) gave the diastereomerically pure epoxide (8aS)-72 in 99% yield. Jones oxidation of 72 followed by treatment with p-TsOH provided (-)-73 in 46% overall yield from 72 as shown in Scheme 11.20 (-)-Drimenol (65) was obtained by treatment of (+)-64 with BF3·Et2O in 90% yield.20 Acetylation of (-)-65 gave a (8aS)-drimenyl acetate (70), which was converted to potent antifeedant such as (-)-warburganal (1).22 (-)-Albicanyl 3,4-dihydroxycinnamate (75) was isolated from Bazzania japonica and Bazzania pompenaa as the major component.23 The reaction of (+)-64 and 3,4-diacetoxycinnamic acid in the presence of DCC / DMAP / CSA gave (8aS)-albicanyl 3,4-diacetoxycinnamate (74) in 50% yield, which was subjected to enzymatic hydrolysis using lipase “Amano P” to provide (-)-75 in 99% yield.20
3.3. Syntheses of (+)-α-polypodatetraene, (-)-copalic acid and (-)-ambrox
Polypodanes constitute a new class of bicyclic terpenoids and their occurrence in nature is important for the mechanistic study of the biosynthesis of polycyclic terpenoids. (+)-α-Polypodatetraene (80) is the first compound of this class and was isolated from the fresh leaves of Polypodium fauriei and Lemmaphyllum microphyllum.24 Straightforward synthesis of (+)-80 from (+)-albicanol (64) was shown in Scheme 11.25 Mesylation of (+)-64 followed by treatment with benzenethiolate ion gave sulfide (8aS)-77 in 74% overall yield from (+)-64. Oxidation of (8aS)-77 with 30% H2O2 in the presence of Mo7O24(NH4)6·4H2O afforded sulfone (8aS)-78 in 90% yield, which was lithiated with LDA followed by treatment with (E,E)-farnesyl bromide to give a diastereomeric mixture of (8aS)-79 in 83% yield. Treatment of (8aS)-79 with 5% Na-Hg gave (+)-80 in 44% yield. On the other hand, (-)-copalic acid (5) isolated from Copaifera sp. was reported to possess antimicrobial activity and oil resin from Copaifera sp. was used as traditional medicine in Brazil.26 (-)-Copalic acid (5) could be only a natural source for the synthesis of (8aR)-labdane type diterpenes. Straightforward synthesis of (-)-5 from (-)-albicanol (64) was shown in Scheme 11.21 (8aR)-Abicanyl phenyl sulfone (8aR)-78 was obtained from (-)-64 in the same way as for the preparation of (8aS)-78 from (+)-64. Treatment of (8aR)-78 with n-BuLi followed by treatment of propylene oxide afforded the reaction product, which was subjected to reductive desulfonylation to give a diastereomeric mixture of secondary alcohol (8aR)-81 in 67% overall yield. Dess-Martin oxidation of (8aR)-81 provided a ketone (8aR)-82 in 97% yield, which was subjected to Horner-Emmones condensation with methyl dimethoxyphosphonoacetate to afford methyl (10R)-(E)-copalate 8 in 63% yield. Alkaline hydrolysis of (10R)-83 gave (-)-copalic acid (5) in 95% yield. Then the synthesis of (-)-ambrox (48) was carried out from (-)-drimenol (65) as shown in Scheme 11.20 (-)-Ambrox (48) is one of the constituents of the
ambergris tincture and possesses a powerful amber-type aroma. Mesylation of (-)-65 followed by treatment with NaCN gave nitrile compound (8aS)-84 in 45% overall yield, which was reduced with Dibal-H to afford an aldehyde (8aS)-85 in 81% yield. NaBH4 reduction of (8aS)-85 provided an alcohol (8aS)-86, which was treated with p-TsOH to give (-)-ambrox (48) in 55% yield.
3.4. Syntheses of (+)-austrochaparol, (+)-coronarin A and (+)-coronarin E
(+)-Austrochaparol (91) was isolated from the aerial parts of Austroeupatorium chaparense and its absolute structure has not been determined yet.27 (+)-Coronarin A (94) and (+)-coronarin E (89) were isolated from rhizomers of the Brazilian antirheumatic medical plant, Hedychium coronarium.28 These compounds exhibit a signifcant cytotoxic effect against Chinese humster V-79 cells and sarcoma 180 ascites in mice. For the synthesis of these compounds, carbon-carbon bond formation between an aldehyde (8aS)-87 and β-furylmethyl heteroaromatic sulfone is necessary as shown in Scheme 12.29 The reaction of 3-furylmethanol with 2-mercaptobenzothiazole (BTSH) in the presence of Ph3P and diethyl azodicarboxylate (DEAD) gave sulfide, which was oxidized with m-CPBA to afford β-furylmethyl benzothiazoly sulfone. Dess-Martin oxidation of (+)-albicanol (64) gave an aldehyde (8aS)-87 in 83% yield, which was reacted with the above mentioned sulfone in the presence of lithium bis(trimethylsilylamide) to afford (10S)-88 (77%) and (+)-coronarin E (89) (11% yield). Hydrogenation
of (10S)-88 gave compound (+)-90 in 86% yield, which was in accord with the natural product (+)-(5S,9S,10S)-15,16-epoxy-8(17),13(16),14-labdatriene. Allylic oxidation of (+)-90 with SeO2 gave (+)-austrochaparol (91) in 50% yield. Consequently, absolute configurations of natural (+)-austrochaparol (91) were confirmed to be 5S, 7R, 9R, 10S. On the other hand, allylic oxidation of (+)-89 with SeO2 afforded (-)-92 in 47% yield, which was subjected to Dess-Martin oxidation to provide α,β-unsaturated a ketone (10S)-93. NaBH4 reduction of (8aS)-93 gave (+)-coronarin A (94) in 78% overall yield from (-)-92.
3.5. Syntheses of (-)-tauranin and (-)-BE-40644
A sesquiterpene quinone (-)-tauranin (99) was isolated from mold Oospora aurantia and fungus Phyllosticta spinarum.30 It has been reported to possess apoptotic activity toward several human solid tumor cell lines,31 and inhibitory activity against the incorporation of acetate-1-14C into cholesterol.32 Meanwhile, sesquiterpene quinone (-)-BE-40644 (103) was isolated from Actinoplanes sp. A 4064433 and reported to inhibit the thioredoxin (TRX) system and to exhibit cytotoxicity against several human tumor cell lines. In addition, strong repression of human immunodeficiency virus (HIV) replication was also reported for this compound.34 In spite of its interesting biological activities, the absolute configurations of (-)-103 have not been determined. The synthesis of (-)-99 and (-)-103 was shown in Scheme 13.35 Common aromatic building block, 4-[(t-butyldimethylsilyl)oxymethyl]-2,6-di(methoxymethyloxy)-benzene for the syntheses of (-)-99 and (-)-103 was prepared from methyl 3,5-dihydroxybenzoate. The previous aldehyde (8aS)-87 was reacted with an anion generated from the above mentioned building block by treatment with n-BuLi to afford a diastereomeric mixture of (10S)-95 in 91% yield, which was subjected to Barton McCombie’s method36 to afford (10S)-96 in 76% overall yield. For preparation of (10S)-98, (10S)-96 was treated with 100 mM of CSA in EtOH for 3.5 days to afford (10S)-97 (18% yield) and (10S)-98 (62% yield). Furthermore, (10S)-97 was again treated with 100 mM of CSA in EtOH to give (10S)-98 in 70% yield. Oxidation of (10S)-98 by Fremy’s salt [(KSO3)2NO] in phosphate buffer gave (-)-tauranin (99) in 63% yield. On the other hand, regioselective acetylation of (10S)-97 with vinyl acetate catalyzed by lipase PS-C to afford (10S)-100 in 92% yield. Treatment of (10S)-100 with N-(phenylseleno)phthalimide afforded a diastereomeric mixture of phenylselenide derivatives, whose phenylselenyl group was removed by tributyltinhydride in the presence of AIBN to afford (10S)-101 as a diastereomeric mixture (6aS : 6aR =11:1) in 82% overall yield. Treatment of (10S)-101 with CSA followed by silicagel column chromatographic separation afforded (10S)-102 as a single isomer in 86% yield. It was treated with Fremy’s salt in phosphate buffer to give (-)-BE-40644 (103) in 43% yield. Synthetic (-)-BE-40644 (103) was identical with natural (-)-BE-40644 (103) and the absolute structure of natural (-)-103 was determined to be 4aS, 6aS, 12aR, 12bS.
3.6. Synthesis of (+)-pacovatinin A
(+)-Pacovatinin A (109) was isolated from seeds of the Brazilian medicinal plant, Renealmia exaltata L.f. (Zingiberaceae)37 and found to inhibit the increase of vascular permeability induced by acetic acid in mice and nitric oxide production in lipopolysaccharide-activated mouse peritoneal macrophages.38 The straightforward synthesis of (+)-109 from (+)-albicanol (64) is shown in Scheme 14.29 Conversion of (+)-64 to albicanyl cyanide (8aS)-49 was accomplished by displacement of the hydroxyl group with cyanide under Mitsunobu conditions39 in the presence of acetone cyanohydrin to give nitrile (8aS)-49 in 64% yield. Allylic oxidation of (8aS)-49 using a combination of SeO2 and tert-BuOOH gave the 7α-hydroxy compound (8aS)-104 in 79% yield. Stereochemical inversion of 7α-configuration to 7β-configuration was achieved by the Mitsunobu method.39 Treatment of (8aS)-104 with PhCO2H in the presence of Ph3P and diethyl azodicarboxylate afforded 7β-acyloxy nitrile, which was successively treated with K2CO3 in MeOH and tBuMe2SiCl in the presence of imidazole to afford (8aS)-105 in 60% yield. Dibal-H reduction of (8aS)-105 gave an aldehyde (8aS)-106 in 87% yield, which was subjected to Horner-Emmons condensation with diethoxyphosphonobutyrolactone in the presence of tert-BuOK to provide the less polar compound (10S)-107 (27% yield) and the more polar one (10S)-108 (63% yield). Deprotection of silyl group of (10S)-108 provided (+)-pacovatinin A (109) in 92% yield.
3.7. Synthesis of (-)-albaconol
Albaconol (115) was isolated from the fresh fruiting bodies of the basidiomycetes Albatrellus confluents,40 and acts as weak antagonists for human vaniloid-receptor (VRI) without agonistic effect.41 Albaconol (115) inhibits significantly the growth of human tumer cell lines in vitro, and stimulates DNA cleavage, stabilizes and increases the topo II-mediated DNA cleavable complex because of inhibition the religation activity of topo II in a dose-dependent manner.42 Structure of 115 was determined by spectral analysis but the absolute structure of 115 was not determined yet. We report the first synthesis of (8aR)-albaconol (115) from (8aR)-albicanol (64) and determination of the absolute structure of natural albaconol (115) based on chiral synthesis (8aR)-115 as shown in Scheme 15.43 Dess-Martin oxidation of (8aR)-64 gave a (8aR)-albicanal (87) in 92% yield, which was treated with 3,5-bis-(methoxymethyloxy)toluene in the presence of n-BuLi to afford a diastereomeric mixture of (10R)-110. Acetylation of (10R)-110 followed by the Birch reduction provided (10R)-111 in 82% overall yield from (8aR)-87. Dihydroxylation of the exo-olefin part of (10R)-111 using OsO4/K3Fe(CN)6 gave stereoselectively the desired diol (10R)-112 in 83% yield, which is corresponding to the dihydroxylation product from less-hindered β-side. The relative configuration of C(2)-position in (10R)-112 could be S, because the present (10R)-112 was finally converted to the enantiomer of natural albaconol (10S)-115 as mentioned later on. Monotosylation of (10R)-112 gave selectively primary tosylate (10R)-113 in 94% yield, which was treated with LiAlH4 to afford tertiary alcohol (10R)-114 in 96% yield. Finally, (10R)-114 was treated with pyridinium p-toluenesulfonate (PPTS) in the presence of ethylene gycol to afford (10R)-(-)-albaconol (115) in 80% yield. In this case, PPTS was used as weak acid to avoid formation of ether linkage between phenolic hydroxyl group and C(2)-carbon. The physical data of the synthetic (10R)-115 were identical with those of natural albaconol (115) except for the sign of the specific rotation. From these evidences, the absolute structure of natural (+)-albaconol (115) was determined to be 1R,2R,4aS,8aS configurations.
4. SYNTHESIS OF BOTH ENANTIOMERS OF VERSATILE CHIRAL SYNTHON BASED ON OPTICAL RESOLUTION USING (R,R)-CYCLOHEPTANE 1,2-DIOL AND THEIR APPLICATION TO THE SYNTHESIS OF FURANODITERPENE
4.1. Synthesis of (8aR)- and (8aS)-decahydro-5,5,8a-trimethyl-2-oxo-naphthalene-1-methanol
Chiral auxiliary, (R,R)-cycloheptane 1,2-diol was obtained based on enzymatic hydrolysis of meso-1,2-cycloheptane diacetate.44 The reaction of (±)-β-keto ester (62) and (R,R)-cycloheptane 1,2-diol in the presence of p-TsOH followed by LiAlH4 reduction gave a diastereomeric mixture of alcohols 116 and 117, which were separated by silicagel chromatography to give the less polar alcohol 116 in 47% overall yield from (±)-62 and the more polar alcohol 117 in 44% overall yield from (±)-62. Acid treatment of 116 gave the (+)-hydroxy ketone (118) (89% yield) and (R,R)-cycloheptane 1,2-diol (87% yield). The physical data of (+)-118 was identical to those of the previously reported (+)-(8aR)-11845 On the other hand, acid treatment of 117 gave the (-)-hydroxy ketone (118) (97% yield) and (R,R)-cycloheptane 1,2-diol (97% yield). The physical data of (-)-118 was identical to those of the previously reported (-)-(8aS)-11845 and the absolute configuration of the present 117 was determined to 1S,4aS,8aS as shown in Scheme 16.46 The present resolution procedure seemed to be highly advantageous because the (R,R)-cycloheptane 1,2-diol could be recovered in high yield from the chiral acetals 116 and 117 by treatment with acid and could then be again used for chiral acetal formation.
4.2. Synthesis of both (10R)- and (10S)-15,16-epoxy-8(17)13(16)-labdatrienes
(-)-15,16-Epoxy-8(17)13(16)-labdatriene (90) has been isolated from the Philippine sponge, Cacospongia species.47 The ent-type of 90 has been isolated from the aerial part of Blepharispermum zanguebaricum. However, the absolute structure of two (+)- and (-)-furanoditerpenes 90 has not been determined yet. The syntheses of (10R)-90 from (+)-(8aR)-118 and (10S)-90 from (-)-(8aS)-118 were shown in Scheme 16.46 Acetylation of (8aR)-118 followed by treatment with NaCN gave a γ-keto nitrile (8aR)-45 in 97% overall yield from (8aR)-118, of which the physical data were identical with those of the reported (8aS)-4514 except for the sign of specific rotation. Wittig olefination of (8aR)-45 followed by reduction with Dibal-H yielded the corresponding aldehyde (8aR)-50 in 87% overall yield from (8aR)-45. The spectral data of (8aR)-50 were identical with those of the reported (8aR)-50.17 Aldehyde (8aR)-50 was reacted with 3-lithiofuran to afford a diastereomeric mixture of secondary alcohol (10R)-119, which was subjected to acetylation followed by a Birch reduction to give a furanoditerpene (10R)-90 ([α]D -42.6 (CHCl3)) in 38% overall yield from (8aR)-50. Similarly, antipodal furanoditerpene (10S)-90 ([α]D +49.3 (CHCl3)) was also synthesized from (8aS)-118 in 28% overall yield from (8aS)-118 in the same way as for (8aR)-90. The NMR data of both enantiomers (10R)- and (10S)-90 were identical with those of the natural (-)-90 and the sign of (10R)-90 was the same as that of the natural (-)-90. Hence, the absolute structure of the natural (-)-90 was unequivocally determined as (5R,9R,10R) as depicted in Scheme 16.
4.3. Synthesis of both (10R)- and (10S)-15,16-epoxy-7,13(16),14-labdatrienes
(+)-15,16-Epoxy-7,13(16),14-labdatriene (123) has been isolated from the Philippine sponge, Cacospongia sp.48 However, the absolute structure of two (+)-furanoditerpene 123 has not been determined yet. The syntheses of (10R)-123 from (+)-(8aR)-116 and (10S)-123 from (-)-(8aS)-117 were shown in Scheme 17.46 Oxidation of (8aR)-116 with PCC gave an aldehyde, which was sujected to oxidation with NaClO2 followed by esterification to afford methyl ester. The methyl ester was treated with 10% aqueous H2SO4 to provide the (8aR)-β-keto ester (62) in 61% overall yield. Conversion of (8aR)-62 to aldehyde (8aR)-85 was achieved by our previously reported procedure (Scheme 11).20 The reaction of (8aR)-85 with 3-lithiofuran prepared from 3-bromofuran and n-BuLi furnished a diastereomeric mixture of secondary alcohol (10R)-121, which was subjected to acetylation followed by a Birch reduction to give a furanoditerpene (10R)-123 ([α]D -14.2 (CHCl3)) in 46% overall yield from (8aR)-85. Similarly, antipodal furanoditerpene (10S)-123 ([α]D +11.6 (CHCl3)) was also synthesized from (8aS)-117 in the same way as for (8aR)-123. The NMR data of both enantiomers (10R)- and (10S)-123 were identical with those of the natural (+)-123 and the sign of (10S)-123 was the same as that of the natural (+)-123 ([α]D +3.2 (CHCl3)). Hence, the absolute structure of the natural (+)-123 was unequivocally determined as (5S,9S,10S) as depicted in Scheme 17.
5. SYNTHESIS OF BOTH ENANTIOMERS OF VERSATILE CHIRAL SYNTHON BASED ON ENZYMATIC RESOLUTION AND THEIR APPLICATION TO THE SYNTHESIS OF THE RELATED NATURAL PRODUCTS
5.1. Synthesis of (8aR)- and (8aS)-decahydro-5,5,8a-trimethyl-2-oxo-naphthalene-1-methanol-2-ethtylene acetal
The substrate, (±)-decahydro-5,5,8a-trimethyl-2-oxo-naphthalene-1-methanol-2-ethylene acetal (124) for enzymatic reaction was synthesized from (±)-β-keto ester (62) as shown in Scheme 18.49 The reaction of (±)-62 and ethylene glycol in the presence of p-TsOH gave the corresponding acetal, which was reduced with LiAlH4 to afford (±)-124 in 91% overall yield from (±)-62. From a screening experiment using various kinds of lipases, lipase “PL-266” from Alcaligenes sp. was found to be effective lipase. A suspension of (±)-124, isopropenyl acetate and lipase “PL-266” in diisopropyl ether was incubated at
33 oC for 1 day and an acetate (8aS)-125 (49%, 99% ee) and unchanged (8aR)-124 (49%, 98% ee) were obtained. Oxidation of (8aR)-124 with PCC gave an aldehyde, which was sujected to oxidation with NaClO2 followed by esterification to afford methyl ester. The methyl ester was treated with 10% aqueous HCl to provide the (8aR)-β-keto ester (62) in 71% overall yield. The present (8aR)-62 was identical with the previously mentioned (8aR)-62 as shown in Scheme 17.
5.2. Formal synthesis of hyatellaquinone
Hyatellaquinone (129) was reported to inhibit the reverse transcriptase of human immunodeficiency virus (HIV)50 and was formally synthesized from (8aR)-62 as shown in Scheme 18.49 Wittig olefination of (8aR)-62 followed by conseuctive reduction and oxidation gave an aldehyde (8aR)-87. The reaction of (8aR)-87 with an anion prepared from 1,2,4,5-tetramethoxybenzene and n-BuLi furnished a diastereomeric mixture of secondary alcohol (10R)-126, which was subjected to acetylation followed by a Birch reduction to give (10R)-128 in 48% overall yield from (8aR)-62. Conversion of (10R)-128 to hyatellaquinone (129) was already achieved.51
5.3. Synthesis of methyl (5R,10R,13R)-labda-8-en-15-oate
Marine dorid nudibranchs are knowm to contain terpenoid glyceryl esters in their mantle. Among them, a single specimen of the Antractic nudibranch Austrodoris kerguelensis contained two major glyceride ester, 2’-acetoxyglyceryl- and 3’-acetoxyglyceryl-(5R,10R,13R)-labda-8-en-15-oate.52 These terpenoid glyceryl ester are potent activators of protein kinase C and very active in a regenerative test with the fresh water hydrozoan Hydra vulgaris. We tried to synthesize methyl (5R,10R,13R)-labda-8-en-15-oate (133) corresponding to the left carboxylic acid part of the above mentioned terpenoid glyceryl ester as shown in Scheme 18.25 Target molecule (133) could be obtained by coupling reaction of (8aR)-bicyclofarnesyl bromide (130) and chiral isoprene unit (S)-131.25 (8aR)-Bicyclofarnesol (67) was obtained from (8aR)-62 by the same way as the preparation of (±)-67 from (±)-62 as shown in Scheme 9 and was treated with PBr3 to give (8aR)-130. Treatment of (S)-131 with lithium diisopropylamide (LDA) gave the corresponding anion, which was subjected to the coupling reaction with (8aR)-130 to afford the coupling product (132) in 70% overall yield from (8aR)-67. Reduction of 132 with Na/Hg in MeOH followed by deprotection of THP group provided an alcohol, which was subjected to the conseuctive oxidation and esterification to afford the final compound (133) in 10% overall yield from 132.
5.4. Formal synthesis of (+)-wiedendiol
(+)-Wiedendiol (135) was isolated from the marine sponge Xestospongia wiedenmayeri and inhibits cholesteryl ester transfer protein (CETP).53 Formal synthesis of (+)-135 from (8aS)-bicyclofarnesol (67) was shown in Scheme 18.54 The enzymatic reaction product (8aS)-125 was converted to (8aS)-bicyclofarnesyl bromide (130) via (8aS)-67 in the same way as above mentioned procedurere. The reaction of an ate complex derived from 1,2,4-trimethoxybenzene with (8aS)-130 gave the desired product (10S)-134 in 56% overall yield from (8aS)-67. The synthsis of (+)-135 from (10S)-134 has already been achieved based on selective demethylation.55
5.5. Syntheses of (+)-austrodoral and (+)-austrodoric acid
(+)-Austrodoral (138)56 and (+)-austrodoric acid (139)57 are two related norsesquiterpenes isolated from the skin extract of Antarctic dorid nudibranch Austrodoris kerguelenensis. They possess a bicyclic structure with the rare carbon skeleton, which could biogenetically arise from a drimane type framework by a ring contraction process. Althogh the main products of the acid-catalyzed rearrangement of epoxides are typically carbonyl compounds, other compounds may be also formed depending on the nature of the epoxides, e.q., number of substituents, functional groups and steric structures. Epoxidation of (8aS)-bicyclofarnesol (67) gave a (8aS)-α-epoxybicyclofarnesol (136),58 which was reated with CF3COOH to afford a rearranged product (137) in 96% yield. The structure of 137 was confirmed by the fact that 137 was converted to (+)-austrodoric acid (139) as shown in Scheme 18.59 NaBH4 reduction of 137 followed by treatment NaIO4 gave (+)-austrodoral (138) in 87% yield. Jones oxidation of 138 gave (+)-austrodoric acid (139) in 79% yield.
5.6. Synthesis of (+)-norsesterterpene diene ester
(+)-Norsesterterpene diene ester (144) was isolated from an Australian marine sponge, Latrunculia brevis, and its structure was determined by detailed spectroscopic analysis, chemical derivatization and degradation.60 Our synthetic plan for (+)-144 is based on synthesis of (13E,10S)-α,β-unsaturated aldehyde (141) followed by the selective construction of the (3E,5E)-diene moiety including a C(2)-stereogenic center in (+)-144 as shown in Scheme 19.54 Treatment of (8aS)-67 with PBr3 followed by acetoacetic ester synthesis gave methyl ketone (10S)-140 [40% overall yield from (8aS)-67], which was subjected to a Horner-Emmones reaction to afford a 7:2 mixture of (13E)- and (13Z)-α,β-unsaturated esters. Dibal-H reduction of this mixture gave a mixture of (13E)- and (13Z)-allylic alcohol, which was separated to give (13E)-allylic alcohol. Dess-Martin oxidation of (13E)-allylic alcohol afforded the desired aldehyde (10S)-141 in 62% overall yield from (10S)-140. For selective construction of the (3E,5E)-diene moiety including C(2) stereogenic center in (+)-144, a modified Julia coupling method using a chiral sulfone (143) was thought likely to be effective. Silylation of methyl (S)-3-hydroxy-2-methylpropionate (142) followed by reduction with LiBH4 gave (S)-alcohol, which was treated with 1-phenyl-1H-terazole-5-thiol (PTSH) in the presence of Ph3P and diethyl azodicarboxylate provided the (S)-sulfide. Oxidation of this sulfide gave the desired sulfone (143) in 50% overall yield from (S)-142. A modified Juia coupling of (10S)-141 and 143 in the presence of lithium bis(trimethylsilyl)amide (LHMDS) afforded a 11:1 (E:Z) mixture of diene. Deprotection of the silyl group in this mixture followed by chromatographic separation provided the desired (3E)-diene alcohol, which was subjected to stepwise oxidation (formation of aldehyde and carboxylic acid) to give the desired (3E)-diene carboxylic acid. Finally, treatment of this (3E)-diene carboxylic acid with CH2N2 gave the (+)-norsesterterpene diene ester (144) in 11% overall yield from (10S)-141.
5.7. Synthesis of (-)-subersic acid
(-)-Subersic acid (149) was isolated from the Papua New Guinean sponge, Suberea sp. and is an inhibitor of human 15-lipoxygenase.61 The structure of (-)-149 was determined by extensive NMR analysis, and (5R,10R)-absolute structure of (-)-149 was deduced based on the positive molar rotation of (-)-149. Our synthetic plan for (-)-149 is based on a Stille coupling between allyl trifluoroacetate (10R)-146 and arylstannane congener (148) as shown in Scheme 19.54 The methyl ketone (10R)-140 was synthesized from (8aR)-67 in 37% overall yield from (8aR)-67 in the same way as for preparation of (10S)-140 from (8aS)-67. This methyl ketone was subjected to conseuctive Horner-Emmones reaction and Dibal-H reduction to give (13E)-allylic alcohol (145) in 45% overall yield from (10R)-140 in the same way as for preparation of (10S)-141 from (10S)-140. The (13E)-allylic alcohol (145) was treated with (CF3CO)2O in 2,6-lutidine gave the corresponding trifluoroacetate (10R)-146 in quantitative yield. Treatment of the reported aryl bromide congener (147) with tert-BuLi followed by addition of n-Bu3SnCl gave the desired stannane congener (148) in 62% overall yield from 147. The reaction of allyl trifluoroacetate (10R)-146 and 148 was carried out using Pd2(dba)3·CHCl3 and CuI as an additive to give a 7:1 mixture (E/Z = 7:1) of the coupled products. Deprotection of the THP group in this mixture followed by stepwise oxidation (formation of aldehyde and carboxylic acid) gave a 9:1 mixture (E/Z = 9:1) of the corresponding carboxylic acid. Finally, deprotection of the MOM group in this carboxylic acid with 6M HCl followed by preparative HPLC separation gave (-)-subersic acid (149) in 13% overall yield from (10R)-146.
5.8. Syntheses of (+)-totarol, (+)-podototarin and (+)-sempervirol
(+)-Totarol (152), a rare tricyclic diterpene phenol possessing an isopropyl group at the C(14) position, was first isolated as a major constituent of the heartwood of Podocarpus totara G. Benn and can also be isolated from a variety of other sources. It has been shown to have antibacterial activity against gram-positive bacteria, particularly methicillin-resistant Staphylococcus aureus (MRSA).62 The structure of (+)-152 was deduced on the basis of chemical and spectroscopic analysis, while the absolute structure was determined by ORD measurement and by direct correlation with dehydroabietic acid (6). (+)-Podototarin (153) was also isolated from the heartwood of Podocarpus totara,63 and its structure was determined based on the synthesis from natural (+)-152. Straighforward syntheses of (+)-152 and (+)-153 are shown in Scheme 20.64 The consecutive treatment of enzymatic reaction product (8aS)-125 with 10% HCl and p-TsOH gave α,β-unsaturated ketone (8aS)-55 in qutantitative yield, which was subjected to a Michael reaction with the anion obtained from the reaction of methyl 5-methyl-3-oxohexanoate with NaOMe to give a 2:1 diastereomeric mixture of (10S)-150 in quantitative yield. Alkaline hydrolysis of this mixture followed by treatment with 5M HCl gave the aldol condensation product (10S)-151 in 55% overall yield from (8aS)-125. Treatment of (10S)-151 with CuBr2 and LiBr gave (+)-totarol (152) in 76% yield. Oxidation of (+)-152 with alkaline potassium ferricyanide [K3Fe(CN)6] gave (+)-podototarin (153) in 45% yield. (+)-Semperviol (156), a rare tricyclic diterpene phenol possessing an isopropyl group at the C(12) position, was isolated from Cupressus sempervirens.65 Straighforward syntheses of (+)-156 from (8aS)-125 is shown in Scheme 20.64 A Michael reaction of (8aS)-55 derived from (8aS)-125 with the anion obtained from the reaction of methyl acetoacetate with NaOMe gave a Michael addition product, which was subjected to alkaline hydrolysis followed by treatment with 5M HCl to provide the aldol
condensation product (10S)-154 in 46% overall yield from (8aS)-125. Lithiation of (10S)-154 followed by treatment with acetone in the presence of ZnCl2 gave aldol product (10S)-155 in 59% yield. Finally, treatment of (10S)-155 with a combination of CeCl3 and NaI, followed by dehydration and aromatization, afforded (+)-semperviol (156) in 82% yield.
5.9. Syntheses of (+)-jolkinolide D and (+)-jolkinolide E
Jolkinolides A, B, C, D (2) and E (159) are diterpenoids, which were isolated from the roots of Euphorbia jolkini Boiss.66 The absolute structure of these compounds was determined by a chemical transformation to ferruginol (8), which possesses an abietane-type skeleton. The absolute structure of (+)-jolkinolide D (2) was also confirmed by X-ray crystallographic analysis. (+)-Jolkinolide D (2) exhibits cytotoxicity, inhibits tumor invasion into the basement membrane, and induces apoptosis in tumor cells.67 Straighforward syntheses of (+)-2 and (+)-159 from the enzymatic reaction product (8aR)-124 are shown in Scheme 21.64 Conseuctive treatment of (8aR)-124 with 10% HCl and p-TsOH gave α,β-unsaturated ketone (8aR)-55 in qutantitative yield. A Michael reaction of (8aR)-55 with the anion obtained from the reaction of methyl acetoacetate with NaOMe gave a Michael addition product, which was subjected to alkaline hydrolysis followed by treatment with 5M HCl to provide the aldol condensation product (10R)-154 in 46% overall yield from (8aR)-124. Lithiation of (10R)-154 followed by treatment with trimethylsilyl chloride (TMSCl) gave a silyl enol ether, which was treated with m-chloroperbenzoic acid (m-CPBA) followed by treatment with tetrabutylammonium fluoride (TBAF) to afford α-hydroxy ketone (10R)-157 in 51% overall yield from (10R)-154. Esterification of (10R)-157 with 2-(diethylphosphono)propanoic acid in the presence of 4-dimethylaminopyridine (DMAP) and 1,3-dicyclohexylcarbodiimide (DCC) gave ester (10R)-158, which was treated with NaH to afford (+)-jolkinolide E (159) in 48% yield. Silylation of (10R)-157 followed by treatment with a dianion
obtained by the reaction of 2-iodoallyl alcohol and n-BuLi gave a diol (10R)-161 [27% yield from (10R)-157] and a diol (10R)-162 [32% yield from (10R)-157]. Desilylation of (10R)-162 followed by treatment with MnO2 gave (+)-jolkinolide D (2) in 76% overall yield from (10R)-162.
6. SYNTHESIS OF BOTH ENANTIOMERS OF EPOXYALBICANOL BASED ON ENZYMATIC RESOLUTION AND THEIR APPLICATION TO THE SYNTHESIS OF THE RELATED NATURAL PRODUCTS
6.1. Synthesis of (+)-ambrein
(+)-Ambrein (163) is a major constituent of ambergris, which is a metabolic of the sperm whale, and is used for the production of expensive perfumes.68 However, the supply of ambergris has become very difficult, as commercial whaling is prohibited, and thus development of efficient synthesis of (+)-163 is strongly desirable. Our synthetic plan for (+)-163 is based on the double bond formation between the left-half A and the right-half B at the dotted line by the modified Julia coupling method as shown in Scheme 22.69 In order to make two chiral building blocks, left-half (10S)-A and right-half (S)-B, both chiral (8aS)-epoxy alcohol 72 and (1S,6S)-β-hydroxy ester 166 appear to be useful starting materials, respectively.
6.1.1 Syntheses of (8aS)-epoxyalbicanol (72) and (1S,6S)-β-hydroxy ester (166)
Epoxidation of (±)-albicanol (64) with m-CPBA gave (±)-epoxyalbicanol (72) in 98% yield, which was subjected to enzymatic esterification using isopropenyl acetate and lipase MY-30 from Candida rugosa to afford an acetate (+)-164 (48% yield, 98% ee) and unchanged alcohol (-)-72 (48% yield, 98% ee) and (50% yield, 91% ee). Enrichment of the enantiomeric excess (ee) of (-)-72 was achieved by repeated enzymatic reaction. The absolute configuration at the C(8a)-position of (-)-72 ([α]D -26.9 (c = 0.92, CHCl3)) was determined to be S by the direct comparison with the sign of [α]D of the reported (-)-(8aS)-72 ([α]D -28.8 (c = 1.0, CHCl3)), thence that of (+)-164 was confirmed to be R.69 On the other hand, reduction of (±)-β-keto ester (165) with n-Bu4NBH4 gave (±)-trans-β-hydroxy ester (166) in 69% yield, which was subjected to enzymatic esterification using isopropenyl acetate and lipase QL from Alcaligenes sp. to afford an acetate (167) (46% yield, >99% ee) and unchanged alcohol (+)-166 (48% yield, 96% ee). Enrichment of the enantiomeric excess (ee) of (+)-166 was achieved by repeated enzymatic reaction. The absolute structure of (+)-166 was determined to be 1S, 6S by the fact that the present (+)-166 was converted to (S)-γ-cyclogeraniol, thence that of an acetate (167) was confirmed to be 1R,6R.70
6.1.2 Synthesis of left-half A (168)
Reduction of enantiomerically pure (8aS)-72 followed by Swern oxidation gave an aldehyde (8aS)-169 in 86% overall yield, which was subjected to Wittig reaction to afford α,β-unsaturated ester. Catalytic hydrogenation of α,β-unsaturated ester gave δ-lactone (10S)-170 in 55% overall yield from (8aS)-169. LiAlH4 reduction of (10S)-170 gave a diol, which was subjected to selective acetylation of the primary hydroxyl group of the diol to afford a mono-acetate. Treatment of the mono-acetate with methoxymethyl chloride (MOM-Cl) gave MOM ether, which was subjected to consecutive alkaline hydrolysis and PDC oxidation to provide left-half A (168) in 39% overall yield from (10S)-170.
6.1.3 Synthesis of right-half B (171)
Treatment of enantiomerically pure (1S,6S)-166 with MOM-Cl gave the corresponding MOM ether, which was reduced with LiAH4 to give an alcohol. Conversion of the alcohol to an iodide (172) using I2/Ph3P/imidazole reagent system followed by acetoacetic ester condensation gave a ketone (173) in 68% overall yield from (1S,6S)-166. NaBH4 reduction of 173 followed by treatment with 1-phenyl-1H-tetrazole-5-thiol (PTSH) in the presence of Ph3P and diethyl azodicarboxylate provided a diastereomeric mixture of (S)-sulfide (174) in 65% overall yield from 173, which was subjected to oxidation to give the desired right-half B (171) in 90% yield.
6.1.4 Modified Julia coupling of left-half A (168) with right-half B (171)
The reaction of left-half A (168) with right-half B (171) in the presence of 1M solution of lithium bis(trimethylsilyl)amide (LHMDS) in THF gave a mixture (E/Z = 1/1) of 175 in 89% yield. Deprotection of the MOM group in 175 gave (E)-(+)-ambrein (163) (43% yield) and (Z)-(+)-ambrein (163) (38% yield).
6.2. Synthesis of (-)-(8R,10S)- and (+)-(8S,10R)-8-hydroxpolypoda-13,17,21-triene
Novel triterpene alcohol 8α-hydroxpolypoda-13,17,21-triene (179) was isolated from a fern, Polypodiodes fomtosana.71 The occurrence of this type of alcohol from nature is important from the mechanistic point of view of the biosynthesis of lanosterol from 2,3-oxidosqualene via a cationic intermediate. The structure of 179 including its absolute configuration has been elucidated as 8α-hydroxpolypoda-13,17,21-triene on the basis of spectral and chemical evidence. In order to reconfirm the absolute structure of natural 179, the synthesis of both enantiomers of 179 was necessitated because of the low value of optical rotation ([α]D -0.9). The key optically active intermediate for the synthesis of (-)-179 and (+)-ent-179 appeared to be the enzymatic products (8aS)-72 and (8aR)-164. An outline of the synthesis of (8R,10S)-8-hydroxpolypoda-13,17,21-triene (179) from (8aS)-epoxyalbicanol (72) is depicted in Scheme 23,72 which involves the preparation of α,β-unsaturated ester (10S)-177 by Wittig condensation followed by coupling reaction of the sulfone (10S)-178 derived from (10S)-177 and trans-geranyl bromide. The synthesis of (+)-ent-179 from (8aR)-164 is fundamentally carried out in the same way as for the synthesis of (8R,10S)-179 and the synthesis of (+)-ent-179 was omitted. Dibal-H reduction of δ-lactone (10S)-170 derived from (8aS)-72 (Scheme 22) gave lactol (10S)-176 in 81% yield, which was subjected to Wittig condensation with Ph3P = C(Me)CO2Et to afford trans-α,β-unsaturated ester (10S)-177 in 83% yield. The reaction of (10S)-177 with trimethylsilyl trifluoromrthanesulfonate (TMSOTf) in the presence of (i-Pr)2NEt and Et3N gave the corresponding silyl ether, which was reduced with Dibal-H to provide an allyl alcohol. The reaction of the allyl alcohol with MsCl in the presence of LiCl and 2,6-lutidine gave the corresponding allyl chloride, which was treated with PhSO2Na·2H2O to afford the desired sulfone (10S)-178 in 50% overall yield from (10S)-177. Lithiation of sulfone (10S)-178 with LDA followed by treatment with trans-geranyl bromide gave a diastereomeric mixture of alkylated product, which was treated with 5% Na-Hg to afford (-)-(8R,10S)-8-hydroxpolypoda-13,17,21-triene (179) ([α]D -0.6, (c = 0.75, CHCl3)) in 18% overall yield from (10S)-178. On the other hand, the synthesis of (+)-ent-179 from (8aR)-164 was carried out. Alkaline hydrolysis of the enzymatic reaction product
(8aR)-164 gave (8aR)-epoxyalbicanol (72) in 98% yield, which was converted to (+)-(8S,10R)-8-hydroxpolypoda-13,17,21-triene (179) ([α]D +0.5, (c = 0.72, CHCl3)). Thus, the absolute structure of natural (-)-179 was determined to be 5S,8R,9R,10S configurations.
7. CONCLUSION
The practical synthesis of both enantiomers of chiral intermediate for the syntheses of the natural products possessing the bicyclo[4.4.0]ring system with 4,4,10-trimethyl groups and their application to the total syntheses of the related natural products possessing biologically activity were achieved. Chiral induction was carried out based on enzymatic resolution or optical resolution using chiral auxiliary.
ACKNOWLEDGMENTS
This work was carried out from 1991 to 2010 at the Faculty of Pharmaceutical Sciences, Toho University. H.A. thanks his devoted students and research associates as shown in references for their great contributions to the research projects.
References
1. B. J. M. Jansen and A. de Groot, Nat. Prod. Rep., 1991, 319. CrossRef
2. B. J. M. Jansen and A. de Groot, Nat. Prod. Rep., 2004, 21, 449. CrossRef
3. T. Ohsawa, Y. Ohtsuka, T. Nakata, H. Akita, and M. Shimagaki, J. Synth. Org. Chem. Jpn., 1976, 34, 920. CrossRef
4. H. Akita and T. Oishi, Chem. Pharm. Bull., 1981, 29, 1567. CrossRef
5. H. Akita and T. Oishi, Tetrahedron Lett., 1978, 19, 3733. CrossRef
6. H. Akita and T. Oishi, Chem. Pharm. Bull., 1981, 29, 1580. CrossRef
7. H. Akita, A. Anazawa, and T. Oishi, Chem. Pharm. Bull., 1981, 29, 1588. CrossRef
8. H. Akita, T. Naito, and T. Oishi, Chem. Lett., 1979, 1365. CrossRef
9. H. Akita, T. Naito, and T. Oishi, Chem. Pharm. Bull., 1980, 28, 2166. CrossRef
10. T. Nakata, H. Akita, T. Naito, and T. Oishi, J. Am. Chem. Soc., 1979, 101, 4400. CrossRef
11. T. Nakata, H. Akita, T. Naito, and T. Oishi, Chem. Pharm. Bull., 1980, 28, 2172. CrossRef
12. T. Naito, T. Nakata, H. Akita, and T. Oishi, Chem. Lett., 1980, 445. CrossRef
13. T. Naito, T. Nakata, H. Akita, and T. Oishi, Nippon Kagaku Kaishi, 1981, 825. CrossRef
14. H. Akita, M. Nozawa, Y. Futagami, M. Miyamoto, and C. Saotome, Chem. Pharm. Bull., 1997,
45, 824. CrossRef
15. H. Akita, M. Nozawa, and H. Shimizu, Tetrahedron: Asymmetry, 1998, 9, 1789. CrossRef
16. M. Nozawa, Y. Murakami, K. Noda, R. Tamatsukuri, K. Kato, and H. Akita, Chem. Pharm. Bull., 2000, 48, 1176. CrossRef
17. M. Nozawa, E. Ono, and H. Akita, Heterocycles, 2000, 53, 1811. CrossRef
18. M. Jung, S. Lee, and B. Yoon, Tetrahedron Lett., 1997, 38, 2871. CrossRef
19. K. Mori and M. Komatsu, Bull. Soc. Chim. Belg., 1986, 95, 771. CrossRef
20. H. Akita, M. Nozawa M, A. Mitsuda, and H. Ohsawa, Tetrahedron: Asymmetry, 2000, 11, 1375. CrossRef
21. M. Fujii, S. Ishii, R. Saito, and H. Akita, J. Mol. Catal. B: Enzymatic, 2009, 59, 254. CrossRef
22. M. L. Oyarzún, M. Cortés, and J. Sierra, Synth. Commun., 1982, 12, 951. CrossRef
23. M. Toyota, Y. Asakawa, and T. Takemoto, Phytochemistry, 1981, 20, 2359. CrossRef
24. K. Shiojima, Y. Arai, K. Masuda, T. Kamada, and H. Ageta, Tetrahedron Lett., 1983, 24, 5733. CrossRef
25. M. Kinoshita, T. Miyake, Y. Arima, M. Oguma, and H. Akita, Chem. Pharm. Bull., 2008, 56, 118. CrossRef
26. B. M. Tincusi, L. A. Jimenez, I. L. Bazzocchi, L. M. Moujir, Z. A. Mamani, J. P. Barroso, A. G. Ravelo, and B. V. Hernandez, Planta Med., 2002, 68, 808. CrossRef
27. F. Bohlmann, C. Zdero, R. K. Gupta, R. M. King, and H. Robinson, Phytochemistry, 1980, 19, 2695. CrossRef
28. H. Itokawa, H. Morita, I. Katou, K. Takya, A. J. Cavalheiro, R. C. B. de Oliveira, M. Ishige, and
M. Motidome, Planta Med., 1988, 311. CrossRef
29. T. Miyake, K. Uda, M. Kinoshita, M. Fujii, and H. Akita, Chem. Pharm. Bull., 2008, 56, 398. CrossRef
30. K. Kawashima, K. Nakanishi, and M. Tada, Tetrahedron Lett., 1964, 5, 122. CrossRef
31. W. E. M. Kithsiri, A. P. Priyani, T. M. Marilyn, K. G. Malkanthi, A. A. Elizabeth, and G. A. A. Leslie, J. Nat. Prod., 2008, 71, 218. CrossRef
32. H. Ozawa and H. Ichikawa, Yakugaku Zasshi, 1970, 90, 480.
33. K. Torigoe, N. Wakasugi, N. Sakaizumi, T. Ikejima, H. Suzuki, K. Kojiri, and H. Suda, J. Antibiot., 1996, 49, 314. CrossRef
34. K. Torigoe, N. Wakasugi, N. Sakaizumi, H. Suzuki, K. Kojiri, and H. Suda, Jpn. Patent, JPO 07304762, A 19951121 (1995).
35. S. Ishii, M. Fujii, and H. Akita, Chem. Pharm. Bull., 2009, 57, 1103. CrossRef
36. D. H. Barton and S. W. McCombis, J. Chem. Soc., Perkin Trans. 1, 1975, 1574. CrossRef
37. M. Sekiguchu, H. Shigenori, A. Ohsaki, and J. Kobayashi, J. Nat. Prod., 2001, 64, 1102. CrossRef
38. T. Morikawa, H. Matsuda, Y. Sakamoto, K. Ueda, and M. Yoshikawa, Chem. Pharm. Bull., 2002, 50, 1045
. CrossRef
39. O. Mitsunobu, Synthesis, 1981, 1. CrossRef
40. Z.-H. Ding, Z.-J. Dong, and J.-K. Liu, Helv. Chim. Acta, 2001, 84, 259. CrossRef
41. H. Veronika, N. Reilinde, M. Frank, F. Joachim, J.-K. Liu, Z.-H. Ding, and S. Marc, Arch. Pharmazie, 2003, 336, 119. CrossRef
42. C. Qing, M.-H. Liu, W.-M. Wang, Y.-L. Zhang, L. Wang, and J.-K. Liu, Planta Med., 2004, 70, 792. CrossRef
43. M. Fujii, S. Ishii, R. Saito, and H. Akita, Tetrahedron, 2008, 64, 5147. CrossRef
44. Z.-F. Xie, I. Nakamura, O. Suemune, and K. Sakai, J. Chem. Soc., Chem. Commun., 1988, 966. CrossRef
45. N. Furuichi, T. Hata, H. Soetjipto, M. Kato, and S. Katsumura, Tetrahedron, 2001, 57, 8425. CrossRef
46. H. Akita, Y. Amano, K. Kato, and M. Nozawa, Tetrahedron: Asymmetry, 2004, 15, 725. CrossRef
47. D. Tasdemir, G. P. Concepcion, G. C. Mangalindan, M. K. Harper, E. Hajdu, and C. M. Ireland, Tetrahedron, 2000, 56, 9025. CrossRef
48. M. A. Aal, F. Bohlmann, T. Sarg, M. El-Domiaty, and B. Nordenstam, Phytochemistry, 1988, 27, 2599. CrossRef
49. Y. Amano, M. Kinoshita, and H. Akita, J. Mol. Catal. B: Enzymatic, 2005, 32, 141. CrossRef
50. R. Talpir, A. Rudi, Y. Kashman, Y. Loya, and A. Hizi, Tetrahedron, 1994, 50, 4179. CrossRef
51. S. Poigny, T. Huor, M. Guyot, and M. Samadi, J. Org. Chem., 1999, 64, 9318. CrossRef
52. M. T. Davies-Coleman and D. J. Faulkner, Tetrahedron, 1991, 47, 9743. CrossRef
53. S. J. Coval, M. A. Conover, R. Mierzwa, A. King, M. S. Puar, D. W. Phife, J.-K. Pai, R. E. Burrier, H.-S. Ahn, G. C. Boykow, M. Patel, and S. A. Pomponi, Bioorg. Med. Chem. Lett., 1995, 5, 605. CrossRef
54. Y. Arima, M. Kinoshita, and H. Akita, Tetrahedron: Asymmetry, 2007, 18, 1701. CrossRef
55. S. Chackalamannil, Y. Wang, Y. Xia, and M. Czarmiecki, Tetrahedron Lett., 1995, 36, 5315. CrossRef
56. E. Alvarez-Manzaneda, R. Chahboum, I. Barranco, E. Cabrera, E. Alvarez, A. Lara, R. Alvarez-Manzaneda, M. Hmamouchi, and H. Es-Samti, Tetrahedron, 2007, 63, 11943. CrossRef
57. V. Kulcitki, N. Ungur, M. Gavagnin, M. Carbone, and G. Cimino, Tetrahedron: Asymmetry, 2004, 15, 423. CrossRef
58. J. H. George, J. E. Baldwin, and R. M. Adlington, Org. Lett., 2010, 12, 2394. CrossRef
59. N. Fujiwara, M. Kinoshita, A. Uchida, M. Ono, K. Kato, and H. Akita, Chem. Pharm. Bull., 2012, 60, 562. CrossRef
60. M. S. Bulter and R. J. Capon, Aust. J. Chem., 1991, 44, 77. CrossRef
61. J. Carroll, E. N. Jonsson, R. Ebel, and M. S. Holman, J. Org. Chem., 2001, 66, 6847. CrossRef
62. H. Muroi and I. Kubo, Biosci. Biotechnol. Biochem., 1994, 58, 192.
63. R. C. Cambie, W. R. J. Simpson, and L. D. Colebrook, Tetrahedron, 1963, 19, 209. CrossRef
64. T. Miyake, H. Kigoshi, and H. Akita, Tetrahedron: Asymmetry, 2007, 18, 2915. CrossRef
65. L. Mangomi and R. Caputo, Tetrahedron Lett., 1967, 8, 673. CrossRef
66. D. Uemura and Y. Hirata, Chem. Lett., 1974, 819. CrossRef
67. H. Kigoshi, T. Ichino, K. Yamada, Y. Ijuin, S. Makita, and D. Uemura, Chem. Lett., 1982, 1689.
68. G. Ohlofl, Fragrance Chemistry., Theimer E. T. Ed., Academic Press, New York, 1982, 535.
69. N. Fujiwara, M. Kinoshita, and H. Akita, Tetrahedron: Asymmetry, 2006, 17, 3037. CrossRef
70. N. Fujiwara, M. Kinoshita, and H. Akita, J. Mol. Catal. B: Enzymatic, 2006, 40, 64. CrossRef
71. Y. Arai, M. Hirohara, Y. Ageta, and H. Hsü, Tetrahedron Lett., 1992, 33, 1325. CrossRef
72. M. Kinoshita, D. Nakamura, N. Fujiwara, and H. Akita, J. Mol. Catal. B: Enzymatic, 2003, 22, 161. CrossRef