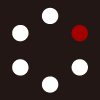
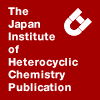
HETEROCYCLES
An International Journal for Reviews and Communications in Heterocyclic ChemistryWeb Edition ISSN: 1881-0942
Published online by The Japan Institute of Heterocyclic Chemistry
e-Journal
Full Text HTML
Received, 28th June, 2013, Accepted, 23rd July, 2013, Published online, 1st August, 2013.
DOI: 10.3987/COM-13-S(S)62
■ Rapid and Convergent Assembly of Natural Benzo[c]phenanthridines by Palladium/Norbornene Catalysis
Pierre-Alexandre Deyris, Tatiana Caneque-Cobo, Filipe Gomes, Vanessa Narbonne, Giovanni Maestri,* and Max Malacria*
ICNS-CNRS UPR2301, Institut Parisien De Chimie Moléculaire (IPCM), Université Pierre et Marie Curie (UPMC), 4 place Jussieu, 75252 Paris Cedex 05 Bâtiment F/74, France
Abstract
A straightforward total synthesis of a small panel of natural benzo[c]phenanthridines is described. The selective coupling of an aryl triflate with a bromobenzylamine by means of palladium/norbornene joint catalysis and a sequential transfer hydrogenation deliver these alkaloids in one pot. Dihydrophenanthridines initially formed undergo dehydrogenation smoothly while norbornene acts both as a catalyst for their assembly and as a sacrificial olefin in their dehydrogenation.Palladium catalysis is a powerful tool for organic chemistry and found wide applications in synthesis owing to its versatility.1 By employing readily available substrates many protocols for the selective formation of C–C and C–Heteroatom bonds were reported in the last two decades. A powerful strategy in the construction of cyclic scaffolds is based on the use of reagents possessing a suitably tethered (masked) nucleophile which could terminate the catalytic cycle by reacting with an electrophilic organopalladium(II) intermediate.2 In this context, the association of a palladium salt with norbornene delivers a remarkable catalytic system that allows the multiple functionalization of an aryl halide in one pot.3 After seminal work by Catellani,4 many synthetic applications were reported which delivers complex polycyclic scaffolds adopting this strategy.3,5 In the context of nitrogen heterocycles, methods that deliver carbazoles,6 phenanthridines7 or dibenzoazepines8 have been reported and this variety clearly demonstrates the large scope of this catalytic system. An efficient synthesis of phenanthridines from aryl iodides and bromobenzylamines is accessible by merging a palladium/norbornene co-catalyzed formation of the corresponding dihydrophenanthridines and their subsequent sequential oxidative dehydrogenation in the presence of oxygen (Scheme 1).7c
Among this class of aromatic heterocycles, benzo[c]phenanthridines are alkaloids which exhibit relevant biological properties.9 We thus decided to test our catalytic methodology towards the synthesis of biologically relevant benzo[c]phenanthridines 1-4 to test its value and potential limitations (Scheme 2).
Our study begun with the attempted synthesis of 1-iodo-6,7-dimethoxynaphthalene to test our previously developed method. Unfortunately, efforts proved fruitless owing to the limited stability of this iodide, which prevents its use as a coupling partner in our cascade. We thus turned our attention to the corresponding triflate, aiming to obtain a comparable reactivity. We were encouraged by the successful exploitation of these substrates by the group of Lautens in a palladium-catalyzed sequence leading to phenanthridines (involving bromoimines as coupling partners).7a Indeed, the desired triflate has been obtained in good yields from 4,5-dibromoveratrole in 3 steps (5, Scheme 3, top). The same strategy readily delivers its naphthodioxole analogue 6. Brominated coupling partners (7,8) were prepared from the corresponding, commercially available aldehydes in two steps (Scheme 3, down).
We then examined our coupling method (reaction conditions as those of Scheme 4, but with 0.5 eq. of norbornene in DMF at 130 °C for 24 hours, followed by one night with on oxygen ballon to trig the oxidative dehydrogenation) and we were delighted to readily obtain O-methylnorfagaronine 1 in 63% yield, shading away our concerns that the reactivity of the triflate could have outpaced that of the deactivated, electron rich benzylamine (for mechanistic discussion vide infra). Through this convergent approach the alkaloid is obtained over four steps in a comfortable 42% overall yield. The coupling reaction of triflate 6 performed poorly as witnessed by a frustrating 16% yield of Nornitidine (2). This is likely due to partial decomposition of the acetal group in the presence of oxygen and water. The same behavior has been observed attempting the synthesis of norallonitidine 3, which was isolated in 17% yield. We then sought to bypass this inconvenient by performing the coupling in the presence of an excess of norbornene7a,7b in order to exploit its dual behavior as both a catalyst to form a dihydrophenanthridine derivative and as a sacrificial olefin in a sequential palladium catalyzed transfer hydrogenation.10 We reasoned that this would have made unnecessary the addition of any oxidant. Indeed, nornitidine 2 could be smoothly prepared in 72% yield (36% overall). Scrambling of substituent on coupling partners provided Norallonitidine 3 in a slightly lower 60% (35% overall) yield while formation of exacyclic Noravicine 4 gave the best outcome (74%, 37% overall). Alkaloid 1 performed equally well under these reaction conditions (64% yield).
Previous computational and experimental studies (both on stoichiometric and catalytic reactions) let us consider the mechanism depicted in Scheme 5 to be at work in these sequences.
The aryl triflate oxidatively adds on palladium(0) to delivering intermediate I.11 Norbornene could then stereoselectively insert into the carbon-metal bond to afford complex II,12 which undergoes C(sp2)–H activation likely with the assistance of a sufficiently basic anion.13 A second oxidative addition on the electron rich palladacycle III could then take place, yielding Pd(IV) complex IV.14 This step is favored by the presence of a chelating group15 on the aryl halide that allows the former to offset the reaction of a second molecule of the triflate on the metallacycle. We were furthermore positively surprised that the reactivity observed with imine derivatives7a at this stage is comparable with those of benzylamines, possessing both a looser chelating arm and a less polarized carbon–halogen bond. In sharp contrast with bromoanilines,8 Pd(IV) reductive elimination then occurs through a selective aryl–aryl coupling, yielding biarylnorbornenylpalladium complex V.14a,15b Steric hindrance favors norbornene extrusion at this stage, delivering intermediate VI3 which could liberate the dihydrophenanthridine upon intramolecular Buchwald/Hartwig amination.16 This secondary amine aromatizes in the presence of Pd(II) species with the concomitant reduction of a sacrificial olefin.7c,10 Identification of diphenylethane in reactions performed in the presence of stilbene confirms this reactivity. A possible alternative mechanism could involve an initial N–arylation of the triflate and subsequent C–Br oxidative addition/C–H activation and ring closure. However, the absence of product formation in reactions performed without norbornene strongly limit the likeliness of this pathway in our condition. The transfer hydrogenation between 9 and norbornene is likely favored by aromatization of the former. Indeed dehydrogenation of the bromide to the corresponding imine (or its hydrolyzed aldehyde) has never been observed (samples were periodically collected and analyzed by NMR before full conversion).
A concise synthesis of biologically relevant benzo[c]phenanthridines has been developed by successfully coupling an aryl triflate with a bromobenzylamine by means of joint palladium/norbornene organometallic catalysis. A sequential transfer hydrogenation using norbornene as a sacrificial olefin could deliver the desired alkaloids in good yields, in the absence of external oxidants.
EXPERIMENTAL
Reagents were obtained from commercial sources and used as received. Substrates 5–6,7 and 7–817 were prepared according to reported procedures. Catalytic reactions were performed using standard Schlenk technique according to the following general procedure. To a Schlenk-type flask were sequentially added under argon Cs2CO3 (197 mg; 0.6 mmol; 2.3 eq.), triphenylphosphine (12 mg; 0.052 mmol; 0.20 eq.), a solution of MeCN (3 mL) containing the aryl triflate (0.29 mmol; 1.1 eq.), the 2-bromobenzylamine (0.26 mmol; 1 eq.) and norbornene (240 mg, 2.6 mmol; 10 eq.) and one of Pd(OAc)2 (6 mg, 0.026 mmol; 0.1 eq. in 3 mL of MeCN). The resulting suspension was stirred at 90 °C until visible formation of palladium black (usually 16–48 h). Upon full conversion, checked by 1H NMR, the mixture was then allowed to cool, diluted with EtOAc (50 mL), washed with a saturated K2CO3 solution (3 × 30 mL) and dried over MgSO4. The solvent was removed under reduced pressure and the products were isolated by flash column chromatography on silica gel. Spectra of alkaloids 1–4 were consistent with those reported in the literature.18 Details and spectra are available as electronic supplementary material.
O-Methylnorfagaronine) (1): Following the general procedure, from triflate 5 (97 mg) and amine 7 (64 mg), 58 mg were obtained as a solid. (64%). Spectral data corresponds to those reported in the literature.18c 1H NMR (300 MHz, CDCl3): δ (ppm) 9.26 (s, 1H, H6), 8.74 (s, 1H), 8.31 (d, 1H, J = 8.9 Hz), 7.91 (s, 1H), 7.87 (d, 1H, J = 8.9 Hz), 7.40 (s, 1H), 7.30 (s, 1H), 4.20 (s, 3H), 4.17 ppm (s, 3H), 4.10 (s, 3H), 4.08 (s, 3H). 13C NMR (75.4 MHz, CDCl3): δ 153.0, 150.0, 149.9, 149.7, 149.6 (C6), 140.3, 128.9, 128.3, 127.5, 126.1, 122.1, 119.8, 118.0, 107.3, 107.0, 104.2, 101.6, 56.2, 56.1(x2), 56.0.
Norallonitidine (2): Following the general procedure, from triflate 5 (97 mg) and amine 8 (60 mg), 52 mg were obtained as a solid (60%). Spectral data corresponds to those reported in the literature.18b 1H NMR (500 MHz, CDCl3): δ (ppm) 9.29 (s, 1H), 8.63 (s, 1H), 8.51 (d, 1H, J = 9.0 Hz), 8.33 (s, 1H), 7.96 (d, 1H, J = 9.0 Hz), 7.69 (s, 1H), 7.54 (s, 1H), 6.29 (s, 2H), 4.02 (s, 3H) 3.96 (s, 3H). 13C NMR (75.4 MHz, CDCl3): δ 151.5, 149.8, 149.5, 147.7, 139.6, 130.4, 128.0, 126.4, 126.1, 123.1, 119.9, 118.8, 107.5, 104.8, 103.7, 102.1, 100.0, 55.5 (x2).
Nornitidine (3): Following the general procedure, from triflate 6 (93 mg) and amine 7 (64 mg), 62 mg were obtained as solid (72%). Spectral data corresponds to those reported in the literature.18d 1H NMR (300 MHz, CDCl3): δ (ppm) 9.33 (s, 1H), 8.65 (d, 1H, J = 9.0 Hz), 8.56 (s, 1H), 8.18 (s, 1H), 7.97 (d, 1H, J = 9.0 Hz), 7.73 (s, 1H), 7.53 (s, 1H), 6.22 (s, 2H), 4.10 (s, 3H), 3.99 (s, 3H). 13C NMR (75.4 MHz, CDCl3): δ 153.3, 149.7, 149.6, 148.0 (x2), 138.4, 129.3, 128.4, 127.9, 126.4, 121.8, 119.8, 119.2, 107.8, 104.5, 102.4, 101.4, 100.9, 56.2, 55.7.
Noravicine (4): Following the general procedure from the triflate 6 (93 mg) and the amine 8 (60 mg), 61 mg were obtained as solid (74%). Spectral data corresponds to those reported in the literature.18a 1H NMR (500 MHz, CDCl3): δ (ppm) 9.29 (s, 1H), 8.55 (s, 1H), 8.53 (d, 1H, J = 9.2 Hz), 8.34 (s, 1H), 7.95 (d, 1H, J = 9.2 Hz), 7.70 (s, 1H), 7.52 (s, 1H), 6.29 (s, 2H), 6.22 ppm (s, 2H). 13C NMR (75.4 MHz, CDCl3): δ 151.8, 149.7, 148.1, 147.9, 141.9, 130.5, 129.6, 129.4, 126.7, 123.1, 122.4, 120.3, 119.2, 104.9, 104.6, 102.2, 101.5, 101.0, 100.1.
Synthesis of epoxynaphthalenes:7a A dried round-bottom flask was charged with the corresponding dibromoaryl (16.89 mmol, 1 eq) and furan (84.47 mmol, 5 eq) in dry toluene (50 mL) under argon atmosphere. The solution was cooled at -78 ºC and n-BuLi 1.6M in THF (18.58 mmol, 1.1 eq) was added dropwise. After complete addition, the solution was warmed up to -40 ºC, extracted with EtOAc (3x20 mL), dried over magnesium sulfate and concentrated under vacuum. The residue was purified by chormatography column (EtOAc/heptane). From 4,5-dibromoveratrol (5 g), 1.91 g of 1,4-dihydro-6,7- dimethoxy-1,4-epoxynaphthalene (56% yield) were obtained as a white solid (¹H-NMR (500 MHz, CDCl3): δ (ppm) 7.01 (s, 21H), 6.94 (s, 21H), 5.65 (s, 21H), 3.82 (s, 6H)). From 5,6-dibromo-1,3- benzodioxole (4.73 g) were obtained 3.17 g of 5,8-dihydro-5,8-epoxynaphtho[2,3-d][1,3]dioxole (81% yield) as a white solid (¹H-NMR (300 MHz, CDCl3): δ (ppm) 7.07 (s, 2H), 6.86 (s, 2H), 5.96 (d, 1H, J = 1.4 Hz), 5.91 (d, 1H, J = 1.4 Hz), 5.66 (s, 2H)).
Synthesis of naphtols:7a To a solution of the corresponding epoxynaphthalene (7.35 mmol) in DCE (50 mL) was added dropwise at 0 °C a solution of p-toluenesulfonic acid (1.47 mmol, 0.2 eq.) in DCE (2 mL). The reaction mixture was stirred at rt overnight. The mixture was diluted with DCM, washed with water and brine, dried over magnesium sulfate and concentrated under vacuum. The products were used for the next step without further purification.
Synthesis of triflates:7a To a solution of the desired naphthol (6.86 mmol) and pyridine (13.72 mmol) in DCM (40 mL) at 0 ºC, was slowly added Tf2O (10.29 mmol). After complete addition the mixture was warmed to rt and stirred until complete conversion of the starting material (1 h). The mixture then was diluted with Et2O (20 mL), quenched with 10% aq HCl and washed with sat. NaHCO3 and brine. After drying (Mg2SO4), the solvent was removed and the residue was purified by column chromatography (EtOAc/heptane).
6,7-Dimethoxynaphthalen-1-yl trifluoromethanesulfonate (5) was obtained (2.04 g, 89% yield) as a pale yellow solid. 1H-NMR (500 MHz, CDCl3): δ (ppm) 7.67 (dd, 1H, J = 6.9, 1.6 Hz), 7.32-7.28 (m, 3H), 7.14 (s, 1H), 4.00 (s, 3H), 3.99 (s, 3H). 13C-NMR (75 MHz, CDCl3): δ (ppm) 151.1, 150.5, 144.7, 131.1, 126.6, 123.5, 122.0, 118.7 (q, JC-F = 319.8 Hz), 116.4, 106.4, 99.3, 56.0, 55.9.
Naphtho[2,3-d][1,3]dioxol-5-yl trifluoromethanesulfonate (6) was obtained (2.19 g, 89% yield) as a pale yellow solid. 1H-NMR (500 MHz, CDCl3) δ (ppm): 7.66 (dd, 1H, J = 5.5, 3.6 Hz), 7.33-7.31 (m, 3H), 7.16 (s, 1H), 6.11 (s, 2H). 13C-NMR (75 MHz, CDCl3): δ (ppm) 149.4, 148.8, 145.3, 132.5, 127.3, 123.8, 123.5, 118.7 (q, JC-F = 320.5 Hz), 116.4, 104.7, 101.7, 97.4.
Synthesis of oximes:17 Hydroxylamine hydrochloride (0.775 mg; 11.15 mmol; 1.2 eq.) was added to a solution of NaHCO3 (0.915 g; 10.9 mmol; 1 eq.) in 25 mL of water. The solution was added to a vigorously stirred suspension of a bromobenzylamine (10.9 mmol; 1 eq.) in EtOH (25 mL). The mixture was stirred for 5 h and then left at 20 °C overnight. The precipitate formed was filtered, washed with a few mL of cold ethanol and dried under vacuum to give the desired hydroxylamine, which is used without further purification.
Synthesis of amines:17 To a solution of the desired hydroxylamine (7 mmol, 1 eq.) in THF (80 mL) was added HCl (2N, 35.3 mL; 70.7 mmol, 10 eq.), followed by zinc (4.62 g, 70.7 mmol, 10 eq.). The mixture was vigorously stirred under reflux until complete conversion. The mixture was then cooled, filtered on a celite pad and concentrated under reduce pressure to remove THF. The aqueous layer was extracted with EtOAc (30 mL) and the pH of the aqueous layer was adjusted to >10 by addition of a saturated ammonia solution, then extracted with EtOAc (3x 30 mL). These last organic layers were combined and dried over anhydrous K2CO3, filtered and evaporated to give a crude, which was purified by flash column chromatography on silica gel (eluent: EtOAc/2% Et3N).
2-Bromo-4,5-dimethoxybenzylamine (7) was obtained (1.43 g, 83% yield) as a white powder. 1H NMR (300 MHz, CDCl3): δ (ppm) 6.98 (s, 1H), 6.89 (s, 1H), 3.85-3.81 (m, 8H). 13C-NMR (75 MHz, CDCl3) δ (ppm) 148.5, 148.4, 134.2, 115.6, 113.2, 112.0, 56.2, 56.0, 46.6.
(6-Bromobenzo[d][1,3]dioxol-5-yl)methanamine (8) was obtained (1.28 g, 80% yield) as a pale yellow solid. 1H NMR (300 MHz, CDCl3): δ (ppm) 6.99 (s, 1H), 6.88 (s, 1H), 5.96 (s, 2H), 3.80 (s, 2H), 1.56 (s, 2H). 13C-NMR (75 MHz, CDCl3) δ (ppm) 147.5, 147.2, 135.5, 113.6, 112.8, 109.1, 101.7, 46.8.
ACKNOWLEDGEMENTS
We thank the CNRS and IUF (M.M.) for financial support.
References
1. (a) J. Tsuji, 'Palladium Reagents and Catalysts–New Perspectives for the 21st Century’, John Wiley & Sons: Chichester, 2004, pp. 409-415; CrossRef (b) F. Diederich, A. de Meijere Eds., 'Metal-Catalyzed Cross–Coupling Reactions', 2nd ed., Wiley-VCH: Weinheim, 2004; (c) T. W. Lyons and M. S. Sanford, Chem. Rev., 2010, 110, 1147; CrossRef (d) D. Alberico, M. E. Scott, and M. Lautens, Chem. Rev., 2007, 107, 174. CrossRef
2. (a) G. Zeni and R. C. Larock, Chem. Rev., 2006, 106, 4644; CrossRef (b) P. Thansandote and M. Lautens, Chem Eur. J., 2009, 15, 5874; CrossRef (c) T. Newhouse and P. S. Baran, Angew. Chem. Int. Ed., 2011, 50, 3362; CrossRef (d) L. Ackermann, R. Vicente, and A. R. Kapdi, Angew. Chem. Int. Ed., 2009, 48, 9792. CrossRef
3. (a) M. Catellani, E. Motti, and N. Della Ca’, Acc. Chem. Res., 2008, 41, 1512; CrossRef (b) A. Martins, B. Mariampillai, and M. Lautens, Top. Curr. Chem., 2010, 292, 1. CrossRef
4. (a) M. Catellani and M. C. Fagnola, Angew. Chem., Int. Ed. Engl., 1994, 33, 2421; CrossRef (b) M. Catellani, F. Frignani, and A. Rangoni, Angew. Chem., Int. Ed. Engl., 1997, 36, 119. CrossRef
5. Recent examples: (a) E. Motti, N. Della Ca’, D. Xu, A. Piersimoni, E. Bedogni, Z.-M. Zhou, and M. Catellani, Org. Lett., 2012, 14, 5792; CrossRef (b) H. Weinstabl, M. Suhartono, Z. Qureshi, and M. Lautens, Angew. Chem. Int. Ed., 2013, 52, 5305; CrossRef (c) L. Jiao, E. Herdtweck, and T. Bach, J. Am. Chem. Soc., 2012, 134, 14563. CrossRef
6. N. Della Ca’, G. Sassi, and M. Catellani, Adv. Synth. Catal., 2008, 350, 2179. CrossRef
7. (a) M. Blanchot, D. A. Candito, F. Larnaud, and M. Lautens, Org. Lett., 2011, 13, 1486; CrossRef (b) N. Della Ca’, E. Motti, and M. Catellani, Adv. Synth. Catal., 2008, 350, 2513; CrossRef (c) G. Maestri, M.-H. Larraufie, E. Derat, C. Ollivier, L. Fensterbank, E. Lacôte, and M. Malacria, Org. Lett., 2010, 12, 5692. CrossRef
8. N. Della Ca’, G. Maestri, M. Malacria, E. Derat, and M. Catellani, Angew. Chem. Int. Ed., 2011, 50, 12257. CrossRef
9. (a) T. Ishikawa, Med. Res. Rev., 2001, 21, 61; CrossRef (b) W. A. Denny, Curr. Med. Chem., 2002, 9, 1655; CrossRef (c) P. H. Bernardo, K.-F. Wan, T. Sivaraman, J. Xu, F. K. Moore, A. W. Hung, H. Y. K. Mok, V. C. Yu, and C. L. L. Chai, J. Med. Chem., 2008, 51, 6699; CrossRef (d) I. Kock, D. Heber, M. Weide, U. Wolschendorf, and B. Clement, J. Med. Chem., 2005, 48, 2772. CrossRef
10. (a) J. E. Bercaw, N. Hazari, and J. A. Labinger, J. Org. Chem., 2008, 73, 8654; CrossRef (b) J. Muzart, Chem. Asian J., 2006, 1, 508. CrossRef
11. L. M. Alcazar-Roman and J. F. Hartwig, Organometallics, 2002, 21, 491. CrossRef
12. C.-S. Li, C.-H. Cheng, F.-L. Liao, and S.-L. Wang, Chem. Commun., 1991, 710.
13. (a) G. P. Chiusoli, M. Catellani, M. Costa, E. Motti, N. Della Ca’, and G. Maestri, Coord. Chem. Rev., 2010, 254, 456; CrossRef (b) D. García-Cuadrado, A. A. C. Braga, F. Maseras, and A. M. Echavarren, J. Am. Chem. Soc., 2006, 128, 1066. CrossRef
14. (a) G. Maestri, E. Motti, N. Della Ca’, M. Malacria, E. Derat, and M. Catellani, J. Am. Chem. Soc., 2011, 133, 8574; CrossRef (b) J. Vicente, A. Arcas, F. Julia-Hernandez, and D. Bautista, Angew. Chem. Int. Ed., 2011, 50, 6896. CrossRef
15. (a) M.-H. Larraufie, G. Maestri, A. Baume, E. Derat, C. Ollivier, L. Fensterbank, C. Courillon, E. Lacôte, M. Catellani, and M. Malacria, Angew. Chem. Int. Ed., 2011, 50, 12253; CrossRef (b) M. Malacria and G. Maestri, J. Org. Chem., 2013, 78, 1323. CrossRef
16. (a) J. P. Wolfe, S. Wagaw, J.-F. Marcoux, and S. L. Buchwald, Acc. Chem. Res., 1998, 31, 805; CrossRef (b) J. F. Hartwig, Acc. Chem. Res., 2008, 41, 1534. CrossRef
17. B. D. Chapsal and I. Ojima, Org. Lett., 2006, 8, 1395. CrossRef
18. (a) Y. Ishihara, S. Azuma, T. Choshi, K. Kohno, K. Ono, H. Tsutsumi, T. Ishizu, and S. Hibino, Tetrahedron, 2011, 67, 1320; CrossRef (b) G. R. Geen, I. S. Mann, M. V. Mullane, and A. McKillop, Tetrahedron, 1998, 54, 9875; CrossRef (c) M. Treus, M. A. Gonazalez, J. C. Estevez, R. J. Estevez, C. O. Salas, and R. A. Tapia, Tetrahedron, 2010, 66, 9986; CrossRef (d) K. Kohno, S. Azuma, T. Choshi, J. Nobuhiro, and S. Hibino, Tetrahedron Lett., 2009, 50, 590. CrossRef