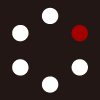
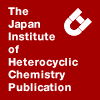
HETEROCYCLES
An International Journal for Reviews and Communications in Heterocyclic ChemistryWeb Edition ISSN: 1881-0942
Published online by The Japan Institute of Heterocyclic Chemistry
e-Journal
Full Text HTML
Received, 30th June, 2013, Accepted, 26th August, 2013, Published online, 6th September, 2013.
■ Hydrazines and Azo-Compounds in the Synthesis of Heterocycles Comprising N-N Bond
Svetlana Tšupova and Uno Mäeorg*
TBD-Biodiscovery, Tiigi 61b, 50410 Tartu, Estonia
Abstract
The synthesis of nitrogen containing heterocycles is of great importance in modern science, owing to their valuable biological properties, and endocyclic hydrazinocycles are no exception. There are methods that enable the transformation of amines to hydrazines, however the use of hydrazine derivatives and azo-compounds as starting materials is both logical and straightforward. In this review we aimed to summarize the methods that use simple hydrazines and azo-compounds for the synthesis of these heterocycles. We begin with simple stoichiometric alkylation of hydrazines and later on move to catalytic systems. Finally, we provide an overview of the advances in the field of azomethine imines chemistry.CONTENTS
1. Introduction 2
2. General considerations of hydrazine alkylation 2
3. Direct alkylation and acylation of hydrazines 3
4. Condensation of hydrazines with carbonyl compounds. 5
5. Mannich-type reaction 7
6. Mitsunobu reaction 8
7. Diels-Alder reaction with azo compounds. 8
8. Synthesis of piperazic acid and its derivatives 14
9. Ring Closing Metathesis of hydrazines for the synthesis of endocycles. 19
10. Formation of azomethine imines from hydrazines 23
11. Cyclizations with azomethine imines to form 5-membered cycles 25
12. [3+3] and other cyclizations of azomethine imines to form larger cycles 34
13. Other hydrazine cyclizations 37
14. Other transition metal catalyzed cyclizations 39
15. Summary 40
16. Acknowledgements 40
17. References 40
1. INTRODUCTION
Hydrazine containing heterocycles are a varied class of compounds and many of them show remarkable biological activity. They are used as anti-inflamatory drugs (e.g. phenylbutazone 1),1 COX (e.g. 2)2 and ACE (e.g. Сilazapril 3)3 inhibitors, pesticides,4 dyes.5 Piperazic acid 4 is an important building block and was used in the synthesis of sanglifehrin A6 and its analogues,7 verucopeptin,8 and antitumor agents (+)-azinothricinand (+)-kettapeptin.9
2. GENERAL CONSIDERATIONS OF HYDRAZINE ALKYLATION
The alkylation of hydrazines face challenges linked to selectivity.10 If a protecting group strategy is employed, the reactions proceed very cleanly, but in poor overall atom economy, which makes it ill-suited to a modern synthetic chemist's mindset.11 Of far greater interest, if more difficult to achieve is the monoalkylation of monosubstituted hydrazines. When performing direct alkylation, the major product is usually hydrazine 6 (a product of 1,2-disubstitution), which often limits the synthesis of 1,2-disubstituted hydrazines 5 to reductive aminations of carbonyl compounds12 or an acylation which is subsequently followed by reduction (Scheme 1).13 In the last few years, several protocols employing iridium10 and palladium14 catalysis were developed for the selective monoallylation of mono- and disubstituted hydrazines, however catalytic alkylations of hydrazines remain still widely unexplored.
The most widely used protocol for the alkylation of hydrazines is the deprotonation of hydrazine with NaH in DMF, subsequently followed by the addition of an alkylating agent. An excess of a mild bases, such as K2CO3,15 or mixtures containing NaOH16 or Cs2CO317 could be used. It was shown that the reaction depends on the solubility of the base,18 which suggests that phase-transfer catalysis (PTC) might be useful. Indeed, the PTC approach enables the use of milder conditions, while being less sensitive to steric hindrance,19 and therefore can be more widely used.
The Mitsunobu approach is very useful as well: especially when a chiral starting alcohol is used, since the reaction proceeds with an inversion at the stereocenter. This enables the synthesis of enantiomerically pure products (in contrast to PTC which generates racemic products).20 A limitation of the Mitsunobu reaction is its sensitivity to the acidity of the hydrazines employed: should the pKa of the starting hydrazine exceed 14 it will be insufficiently active to participate in the reaction.21
3. DIRECT ALKYLATION AND ACYLATION OF HYDRAZINES
Probably the most straightforward method to synthesize cyclic hydrazines is the alkylation of disubstituted hydrazines 7 by dihalides. This method was first mentioned by Overberger22 and still widely used for the synthesis of aliphatic cycles, since suitably protected hydrazines are readily available.
The direct alkylation of hydrazine starts with its deprotonation, usually by a NaH dispersion in DMF or diglyme (Scheme 2).23 The most common alkylating agents are dibromides (both primary, and in some cases, secondary), though ditosylates24 have been used as a cheaper alternative. Reaction yields depend on the size of the generated ring, and examples of the preparation of 5- to 8-membered cycles are found in literature. Similarly, by using a milder base (K2CO3) and 3-chloropropanoyl chloride or 4-chlorobutanoyl chloride, the analogous acylation/alkylation of hydrazines was also performed (Scheme 2).25 PTC conditions are also applicable for cyclizations: NaOH and catalytic Et4NBr in aqueous medium, with heating was used to generate 5- to 7-membered cycles in good yields (78-95%).26
Pyrazolidines 8 could be obtained easily from disubstituted hydrazines by using dihalopropane for the alkylation via the polyanion strategy,27 however attempts to prepare larger heterocycles failed (Scheme 3).28
Very similarly, the dimesylates obtained from 2,2’-oxydiethanol could be used for alkylation. Thiadiazepane 9 was obtained by performing an alkylation using 2 equiv of dichloroethane, under mild PTC conditions and then refluxing with Na2S in EtOH to generate the desired cycle 9 (Scheme 4).29
Acylations have also been performed by using carboxylic acids in conjunction with coupling agents, as was shown by Bihel et al.30 For example, 2-(carboxymethyl)benzoic acid ester was condensed with a 1,2-disubstituted hydrazine, the first step was the condensation of benzoic acid with hydrazine which was achieved through the use of HBTU and, in a second step, acylation with ester occured.
1. CONDENSATION OF HYDRAZINES WITH CARBONYL COMPOUNDS
Cyclic systems are accessible from carbonyl compounds, providing a functionality reactive to the hydrazine’s NH group is available after the hydrazone formation.31 The rearrangement of unstable hydrazones 10 formed in the reaction of hydrazines with α,β-unsaturated ketones, discovered by Fisher and Knoevenagel is such an example.32 However its main limitations are long reaction times and moderate yields (31-54%).
The obtained pyrazolines can be reduced with H2 on Pd/C,32 or with LiBEt3H leading to pyrazolidines.33 Ketoesters have been cyclized with arylhydrazines upon heating with p-TsOH as catalyst.34 The reflux of hydrazine hydrate in EtOH with aldoesters and p-TsOH yielded 6- to 8-membered cyclic hydrazines 11.35 Ketoacids could also participate in the same type of reactions, giving dihydropyridazin-3(2H)-one derivatives upon reflux in EtOH with hydrazine hydrate36 (Scheme 5) or with addition of NaOAc (which accelerates the reaction).37 Reactions proceed slowly (1-2 days),38 and the obtained cyclic structures bear imine bonds which could undergo further reaction, such as reduction by NaBH3CN.39 In principle, cycles containing π-bound prochiral carbon could be interesting substrates for asymmetric reduction, though this remains unexplored at the time of writing.
The treatment of 4-hydrazinoquinazoline 12 with 2,4-diketoesters, after cyclization and a Dimroth-like rearrangement leads to a build-up of 2H-[1,2,4]triazino[2,3-c]quinazolin-2-one core 13 (Scheme 6). The reflux of obtained compound with hydrazine hydrate in i-PrOH resulted in the formation of spiro-heterocycles 14.40
Hydrazine could also be employed in cyclizing 2-hydroxy-1,4-diketones: in a reported procedure, ketoesters 16 were reacted with glyoxals 15 and in the presence of hydrazine cyclized to pyridazines 17 (Scheme 7). 41
The intramolecular formation of a hydrazone employing a substituted hydrazine, bearing an aldehyde in its side-chain, could be envisioned as an another method of cyclization. For example, aldohydrazines for cyclization were generated by the oxidation of alcohol 18 using a periodane such as IBX, in the reported case, the cyclization yields to derivatives of 19 (Scheme 8). 42
A sequentional proccess can be employed, in this example, a reductive hydrazination with hemiacetal 20 and subsequent mesylation of OH functionality were done. Then an intramolecular alkylation of the NH produced cyclic hydrazines 21, though the synthesis generated a mixture of 2 diastereomers (Scheme 9). 43
5. MANNICH-TYPE REACTION
Hydrazines 22, bearing a hydroxyl group on their side-chain can participate in a condensation with formaldehyde. The 1,3,4-oxadiazinane or 1,3,4-oxadiazepane 23 cores were prepared by heating with paraformaldehyde or formaline (Scheme 10).50
6. MITSUNOBU REACTION
Mitsunobu chemistry is well developed for hydrazine alkylation, however to the best of our knowledge, was only once it was used for cyclization, generating 24 (Scheme 11).44
7. DIELS-ALDER REACTION WITH AZO COMPOUNDS
Azodicarboxylates 25 are among most reactive dienophiles, so the rate of hydrazine Diels-Alder reaction is mostly dependent on the employed diene, with reaction times varying from 1 hour to several days, in normally good yields.45,46
Both acyclic46,47 and cyclic47,48 dienes are known to couple with azo-compounds, however the reaction is limited, in that it only allows for the synthesis of 6-membered rings (Scheme 12). Piperidazines can be further reduced with Pd/C or PtO2 as hydrogenation catalysts47 or subjected to other types of reactions by capitalizing on the reactivity of its double bonds: racemic and stereoselective Rh-catalyzed hydroboration yielding to 26,48 formation of cis-diols 27 (oxidation with Os-reagents)49 and trans-diols 28 via an epoxydation with dimethoxyoxirane50 or by using TFD directly50 or generated in situ.51 Additionnaly, the formation of aminoalcohols 29,49,52 bromination to 3053 and the hydroxyfluorination to 3154 have all been reported (Figure 2).
The Diels-Alder reaction of DEAD with 2,9-dilakylpentacenes 32 proceeded smoothly in refluxing toluene (37-63% yields), as shown in Scheme 13.55 The obtained compounds 33 are stable at room temperature, but underwent a retro-Diels-Alder reaction when heated to 300 oC.
The azo-compounds used as starting materials for hetero-Diels-Alder reactions can be generated in situ via the oxidation of hydrazines. Phthalazinedione 34 was treated with NBS and a diene to give the corresponding cycloadduct 35.56 Azo-diene for intramolecular Diels-Alder reaction could be obtained from hydrazide 36 by oxidation with TBA-IO4, the Diels-Alder trapping of obtained azo-compounds gives bicyclic hydrazines 37 (55-78%).57 In situ oxidation was accomplished also by means of an aerobic Cu(II)-catalysis; in the example shown for this transformation, the bicyclic hydrazine 37 was obtained in excellent yield (Scheme 14).58
The facial selectivity of the Diels-Alder reaction was investigated, using cis-3,5-cyclohexadiene-1,2-diol derivatives as dienes.59 In the case of azo-dienophiles, most reactions gave anti-products 39-40 (Table 1). The formation of some syn-products 38 was explained by hydrogen bonding between OH groups of the diene and the dienophile (Figure 3).
It was shown, that the aza-Diels-Alder reaction could be done with Wang-resin-bound dienes 41.60 This was performed in three steps: in situ generation of azo-compound, cyclization and hydrolytic removal of the product 42 from resin to give yields in the range 8-53%. However, the simple isolation of the products is advantageous in this case. Aromatic triazolediones give the best yields in this reaction (Scheme 15).
Enantioselective Diels-Alder reaction
Though some previous achievements in this area are reviewed elsewhere,61 the field has advanced in the past six years. Among the most recent advances, cationic gold(I) bearing chiral phosphoramitide ligands were shown to catalyze enantioselective hetero-Diels-Alder reaction.62 These reactions tolerate a variety of different dienes (43) and proceed in toluene at -78 °C to give cycloadducts 45 in excellent yields and enantioselectivity. More interestingly, cascade gold-catalyzed enyne cycloisomerization/Diels-Alder reaction proceeds very efficiently for a variety of enynes 46 to give 47 in very good yields and ee-s (Scheme 16). In enyne isomerization, the gold-catalyst acts as a π-acid and the Diels-Alder reaction is affected by gold acting as a σ-acid. By these means, the coordination of heteroatom to Au lowers the LUMO energy of dienophile.
Proton driven Diels-Alder reaction
Dialkyldiimines 48-49 in contrast with azodicarboxylates are poor dienophiles for Diels-Alder reaction, so the protonation of dialkylazocompounds was shown to facilitate the reaction greatly.63 When the α-proton of the azo-compound is abstractable, the protonation would lead to a rearrangement to a hydrazone, but in the cyclic dialkyldiimines this reaction is suppressed due to strain in putative hydrazone. Therefore, protonated cyclic dilakylazocompounds could be used in Diels-Alder reactions. The bicyclic compounds 50 could be synthesized in this manner (Figure 4). It is useful to keep in mind that reduction of Diels-Alder adducts sometimes lead to retro-Diels-Alder reaction and reduction with diimine is shown to proceed better than catalytic hydrogenation.64
Inverse azo-Diels-Alder reaction
The hydrazine moiety could be incorporated into a cycle via Diels-Alder reactions, when the starting azo-compound 52 is part of a diene.65 Azo-alkenes are obtained by treatment of β-chlorohydrazone 51 with base, and can be reacted with methoxystyrene to generate cyclic hydrazone.66 Interestingly, pyrrole could be used as a dienophile in the reaction with azoalkenes. In this case non-cyclic products 53 are obtained (Scheme 17). Similarly, the use of tetrazines as cyclic azo-derivatives lead to inverse Diels-Alder adducts.67
Tandem Diels-Alder and other transformations
A tandem Diels-Alder reaction and ozonolysis, which would be followed by a reduction was envisioned as a synthetic method towards linear diamino diols.68 Additionally, following a similar method, N-N endocycles are also accessible (Scheme 18). After the Diels-Alder coupling, a reductive ozonolysis was performed to yield diether 54, the reaction was very sensitive to temperature and as such it was necessary to carefully control it.
Ellis and King suggested combining the Diels-Alder reaction with a sequential ring-opening cross metathesis to produce cyclic hydrazines (Scheme 19).69 In the case of [2.2.1] bicycle 55, the reaction furnished the desired diene 56 as a 2.5:1 mixture of the E and Z isomers in nearly quantitative yield. These results were obtained by the slow addition of alkenes to a dichloromethane solution containing Grubbs’ first generation catalyst, but when a mixture of alkenes and Grubbs’ catalyst were stirred in benzene, oligomers were formed instead. As always, the ring strain is the driving force of this ring opening metathesis reaction, as the less strained [2.2.2] bicycles don’t react.
8. SYNTHESIS OF PIPERAZIC ACID AND ITS DERIVATIVES
Piperazic acid 4 and its derivatives are important compounds. Their skeleton is often found in biologically active compounds and therefore it is important to have methods for the stereoselective synthesis of piperazic acid and its derivatives, that can be readily scaled up.
Gomez de la Oliva et al. performed the synthesis of 59, shown in Scheme 20.70 It consisted of the addition of imine 57 to alkynoates followed by a cyclization using hydrazine hydrochloride in the presence of NaOAc in boiling EtOH. Substituents in the 5-position could be introduced in the intermediate 58 under PTC conditions, whereupon the cis-product 61 is obtained after cyclization. Alternatively, cyclic compounds 59 could be alkylated, giving rise to trans-products 60. The resulting dihydropyridazinones 59 were deprotonated with LiHMDS to allow an alkylation of the carbon. Furthermore, the obtained protected cyclic hydrazones could be reduced with NaBH3CN in methanol to tetrahydropyridazines. Noticeably, the stereoselectivity of the reduction is dictated by the substituent on the 4-position of the ring, as the hydride attack occurred from the opposite site giving rise to 3,4-cis isomers from compound 61 (de > 98%).
Azafagomine analogue of L-fucose was prepared according to Scheme 21. To begin with, D-ribose was protected with acetone and tosylated, subsequently underwent the Finkelstein reaction to generate the iodide and finally hydrogenated to 62. The reductive amination of the obtained hemiacetal 62 with BocNHNH2 gave a disubstituted hydrazine 63 which after protection/deprotection steps was cyclized by an intramolecular alkylation of hydrazine, with mesylate and using K2CO3 as a base, to give compound 64 and after deprotection 4,5-dihydroxy-3-methylhexahydropyridazine.71
In the work of Danishefsky and coworkers,72 the synthesis started from 65, which was obtained from D-glutamic acid (Scheme 22). Then 65 was converted to a hydroxyl ester via the epoxy ester. After a protection of the hydroxyl group (66), the ester was deprotonated and treated with BocN=NBoc which lead to the trisubstituted hydrazine 67, bearing Br on its side chain. A treatment of the obtained compound with NaH in DMF supplied the desired cycle 68 as a 1:1 mixture of two isomers.
Synthesis with Evans chiral auxiliaries
Jogiya and coworkers reported the first synthesis of piperazic acid using (R)-4-benzyloxazolidin-2-one as chiral auxiliary.73
After deprotonation of the oxazolidinone, using BuLi, and an acylation with 5-bromovaleryl chloride to give 69, an electrophilic hydrazination with BocN=NBoc was performed. The obtained lithium aza-enolate was cyclized using an excess of DMPU (26 eq.) as additive to give 70 (Scheme 23). After hydrolysis, 67% of the auxiliary was recovered. Additionally, a synthesis employing another oxazolidine, (4S,5R)-4-methyl-5-phenyloxazolidin-2-one was attempted. In this case the S-piperazic acid was obtained in 88-93% ee, however thanks to an easier work-up procedure and the possibility to separate the R isomer by recrystallization, this method has remained attractive. It was shown that catalytic amounts of tetrabutylammonium halide (15 mol %) could induce cyclization of aza-enolate to 70. Furthermore, cyclization was observed without additives when a THF/DCM/hexane mixture was used as a solvent and the reaction warmed to room temperature. However, probably due to long reaction times (up to 18 h), an erosion of ee was observed. A similar DMPU-induced cyclization procedure was used in the work of Coats and coworkers,74 with cyclization product 70 obtained this way in 99% yield.
Proline-catalyzed reactions
The (S)-proline catalyzed hydrazination of 6-bromohexanal 71 with BocN=NBoc was shown to proceed in excellent yield and > 99% ee.75 The cyclization of the obtained hydrazine 72 via deprotonation with NaH in DMF gave the desired cyclic product 73, which after hydrolysis, oxidation and deprotection provided (R)-piperazic acid. It was also shown that this procedure could be scaled up (Scheme 24).
Aza-Diels-Alder reaction for the synthesis of piperazic acid
As it shown above, the Diels-Alder reaction is widely used for the synthesis of 6-membered cycles, and its variation with aza-compounds used as dienophile is valuable for the synthesis of piperazine derivatives. The reaction with dienophile 75 was modified to generate cycles 76 in a stereoselective manner.76 Evans auxiliary was used with diene 74, but gave rise to almost racemic mixture. It was found that TiCl4 exerted a tremendous effect on the reaction (Scheme 25). The use of 3 equiv of TiCl4 gave 76 with a diastereomeric ratio of 97:3 with 95% isolated yield. In this reaction, 2 equiv of TiCl4 complex with the dienophile and the third equivalent is chelated by the diene (74), ensuring high diastereoselectivity. The reduction of 76, followed by a reductive cleavage of the chiral auxiliary, the manipulation of protecting groups on nitrogen and oxidation of the obtained alcohol gave di-Cbz-(S)-piperazic acid. However direct conversion of 76 to piperazic acid proved itself impossible due to the lack of solubility of the starting material.
Piperazinephosporic acids and phosphonates
In the report by Yoshifuji and coworkers,77 1-methoxybutadiene was reacted with azo compounds and after successive Lewis acid-assisted phosphorylation, hydrogenation and hydrolysis, racemic piperidazine-3-phosphonic acid was obtained. An alternative synthesis of (S)-piperidazine-3-phosphonic acids was accomplished: (buta-1,3-dien-1-yloxy)trimethylsilane was used as diene and di-(−)-menthyl azodicarboxylate as dienophile in the presence of TMSOTf and P(OMe)3.78 After hydrolysis the two isomers, arose from phosphorylation (in a ratio 2:1), were separated by column chromatography (Scheme 26, top). Marchard-Brynaert et al.79 reported a method for the synthesis of aminophosphonates, making use of the aza-Diels-Alder reaction between BocN=NBoc and 1-diethoxyphosphorylbyta-1,3-diene 77 under microwave activation (Scheme 26, bottom).
2-Phosphonated dienes could be obtained from Peterson synthesis and it was shown that Diels-Alder reaction (77% yield) is also possible in this case.80
9. RING CLOSING METATHESIS OF HYDRAZINES FOR THE SYNTHESIS OF ENDOCYCLES
There are only a few examples of Ring Closing Metathesis (RCM) of 1,2-disubstituted hydrazines in literature, a possible reason could be the problematic synthesis of the substrates.
In Tae and Hahn’s work,81 dienes were prepared by the 2-step sequential alkylation of diprotected hydrazines. Cbz-NHNH-Boc was used as starting material, NaH as a base and bromide bearing olefins as alkylating agents (Scheme 27). The first alkylation to 78 encountered problems with its selectivity, yielding a mixture of monoalkylated and dialkylated hydrazines due to insufficient difference in acidity between the two N-H groups (estimated to be less than 0.4 units of pKa).22 However the second alkylation supplied the desired dienes 79 in good yields. The RCM employed 10 mol % of Grubbs first generation catalyst, with more diluted DCM solutions and longer reaction times for larger cycles. The 6- to 10-membered cycles 80 were obtained in good to excellent yields. Three examples of ring-closing enyne metathesis were also shown to give corresponding 6- to 8-membered cycles in good yields (70-99%).
The polyanion strategy was also applied for the synthesis of RCM precursors.82 Various hydrazines with allyl substituent on the Boc-bearing nitrogen 81 were prepared by the double alkylation of dianions, but difficulties appeared with an increase in the chain length of the substituents. Therefore a 2-step strategy was devised: a monoalkylation of the dianion to 82, followed by a second alkylation under PTC conditions were conducted, which resulted in the synthesis of hydrazinic dienes 83 with different substitution pattern. Further reaction by RCM furnished 6- to 9-membered heterocycles in good yields using either Grubbs’ first or second generation catalysts (Scheme 28).
Ring closing metathesis was recently applied to the synthesis of hydrazinoacids (Scheme 29). The tetrasubstituted hydrazinic dienes 84 were obtained by the alkylation of the trisubstituted hydrazine with bromides, using NaH in DMF. Products 85 having undergone the metathesis using Grubbs second generation catalyst, were used to synthesize hydrazino acids 86 by reduction, deprotection and finally hydrolysis.83
Callens et al. prepared the metathesis substrates by sequential allylation and acylation of hydrazines. The yield of the first step was good for all reported compounds, and the efficiency of the acylation of 87 to 88 depended strongly on the bulk of substituent R3 (Scheme 30). The RCM worked well with substrates with low steric hindrance employing Hoveyda-Grubbs second generation catalyst, but attempts to cyclize sterically demanding substrates failed.84
Rutjes et al. (Scheme 31) started from BocNHNH2 which upon condensation with benzaldehyde and successive coupling with vinylmagnesium bromide gave allylated hydrazine 89. It was protected and after treatment with NaH, alkylated with chlorides or tosylates to 90. Yields for the second alkylation were 40-76%. The ring-closure was performed with 20% Grubbs second generation catalyst at 100 oC and gave differently fluorinated pyridazines 91 with 52-83% yield.85 The requirement for high catalyst loadings in this reaction could be linked to the difficulty of the metathesis of electron-deficient double bonds.
In a report by Tae et al. (Scheme 32), RCM substrates 95 were prepared by (S)-proline catalyzed amination of aldehydes 92 with azo-compounds, followed by indium-mediated allylation of 93. The obtained hydrazinic alkene 94 was protected with TBSOTf, subjected to deprotonation with NaH and either alkylation with allyl or propargyl bromide, or acylation with acroyl chloride. The coupling of both alkenes to hydrazine proceeded with good yields (67 to 90%) and the obtained dienes 95 were cyclized with 10% of Grubbs first generation catalyst in refluxing DCM, to furnish 8-membered cycles 96 in 66 to 92% yield. An example of enyne metathesis gave the desired cyclic diene 97 in 75% yield. Despite the long synthesis of dienes, the advantage of this strategy is that proline catalyzed amination coupled with In allylation opens up a route towards the synthesis of enantioenriched cycles (98-99% ee), from achiral starting material.86
Kim and Lee87 reported a double RCM strategy with the subsequent reductive cleavage of the N-N bond to form macrocycles. They, however, mainly focused on the first RCM (Scheme 33). The preparation of dienes started with the Rh-catalyzed coupling of unsaturated aldehydes 98 to azo-compounds, followed by an allylation of 99 with allyl bromide (to 100) or alkenyl esters of bromoacetic acid (to 101) using Cs2CO3 in DMF as base. Attempts to perform the RCM were successful and gave 8- to 13-membered cyclic compounds with 42-93% yields. Enyne metathesis proceeded more sluggishly. 12- and 13-membered cycles products were obtained as mixture of E and Z isomers, with a preference for Z-products, which is consistent with RCM mechanism.
RCM was also used as a key step in the synthesis of analogues of Sanglifehrin.88 The macrocyclization to yield 22-membered cycle on Figure 5 proceeded with Grubbs’ first generation catalyst in 47-57% yield.
10. FORMATION OF AZOMETHINE IMINES FROM HYDRAZINES
A general synthetic scheme for the formation of azomethine inimes (Scheme 34) is showed below. As can be seen from this scheme, azomethine imines are derivatives of hydrazines.
The synthesis of azomethine imines has been reviewed elsewhere,89 however some recent developments are discussed below. Recently, a new route starting from alkenes was reported (Scheme 35). Hydrazones 102 are starting compounds for these reactions. When treated with alkenes under microwave irradiation, they form N-N cyclic azomethine imines 103-104. These reactions proceed with different leaving groups and hydrazone substituents, but the best yields are achieved for bulky substituents. Additionally different alkenes, dienes, styrenes and dihydrofurans are tolerated. Despite the use of an excess of alkene, a probable explanation for the non-occurrence of the competing [3+2] cycloaddition (see below) is steric shielding.90
In a cascade process, azomethine imines 106 were formed and cyclized into a system of 3 fused rings 107 (Scheme 36, top). For this, chloro-enals 105 were treated with monosubstituted hydrazines in presence of DiPEA in boiling toluene. The formation of hydrazone and alkylation was followed by [3+2] cycloaddition to the azomethine imine 106 which furnished the aforementioned product. Analogously, 2-(2-bromoethyl)benzaldehyde 108 was treated with monosubstituted hydrazines in the presence of alkenes, which gave products 109.91
Acyclic azomethine imines (formed in situ from aldehydes and disubstituted hydrazines) underwent an Ugi-type reaction with isocyanides. Catalyst 110 was used in m-xylene, giving products in excellent yield and ee. Aromatic aldehydes with different aryl-isocyanides are excellent substrates for this reaction (Scheme 37).92
11. CYCLIZATIONS WITH AZOMETHINE IMINES TO FORM 5-MEMBERED CYCLES
Though partly within the scope of this paper, the enantioselective copper-catalyzed [3+2] cycloadditions have already been reviewed elsewhere and consequently will not be dealt with.93
Cyclizations with N-N cyclic Azomethine imines
The stereoselective [3+2] cycloadditions of azomethine imines with α,β-unsaturated aldehydes catalyzed by chiral secondary amines were reported.94 In this strategy, an acrylaldehyde derivative reacts with a chiral amine to form an iminium compound, which then participates in a cycloaddition, which enables stereoselective reactions. Amine 111 (10 mol %) with TFA (10 mol %) as additive in wet THF proved to be optimal catalytic system. Azomethine imines derived from aromatic aldehydes participate in the reaction with ketones bearing β-aliphatic substituents, giving bicycles 112 in good yields and in preference to exo-cycles (81:19 to 98:2). Exo-cycles were synthesized in good to excellent ee-s. Cinnamaldehyde didn’t participate in this reaction (Scheme 38).
Similar reactions of unsubstituted acrolein and azomethine imines catalyzed by (S)-proline were demonstrated to provide the endo-product 113a preferentially. The use of azomethine imines derived from aromatic aldehydes, and therefore differently substituted, was probed but ee-s drop dramatically for aliphatic azomethine imines. Interestingly, when (S)-indoline-2-carboxylic acid is used as a catalyst, the formation of the exo-product 113b is observed with excellent selectivities and ee-s (Scheme 39). Finally it was shown that in the presence of (S)-proline 113a isomerize to 113b (92:8 to 6:94 after 65 h).95
Reactions between azomethine imines and 3-acryloyl-2-oxazolidinone catalyzed by Ni(II)-binaphthyldiimine complexes were shown to proceed with high eneatioselectivity (Scheme 40).96 A selection of different aromatic azomethine imines proved effective in this cyclization, giving good yields as well as good to excellent dr-s and ee-s. Sterically encumbered nickel-intermediates allow only Si-attack of azomethine imine to 3-acryloyl-2-oxazolidinone, which is the source of the enantioselectivity (Figure 6).
Homoallylic alcohols were used as cyclization partners in an asymmetric form of this reaction (Scheme 41), in which 20 mol % of (R,R)-diisopropyl tartrate and 1.5 equivalents of Grignard reagent were employed. As a result, cyclization products 115 were obtained with good yields and good ee-s.97
Arynes have also been cyclized with azomethine imines.98 It was observed that benzynes are formed from 116 when treated with 1.25 eq. of TBAT, and that the reaction with azomethine imines yields aryl-fused tricyclic systems 117. Reactions with three arynes were attempted, and gave average yields, but a wide variety of azomethine imines could be used in those reactions. Surprisingly, aliphatic azomethine imines generate products in better yields (Scheme 42, right).
N-Arylmaleimides 118 could also serve as partners for [3+2] cyclization with azomethine imines (Scheme 42, left). A variety of maleimides that bore different substitution patterns were reacted with azomethine imines in non-catalytic process, to form tricyclic systems 118 as a mixture of cis and trans isomers (1:1.4 to 1:3.1 respectively) in good overall yields.99
Alkynes constitute another class of compounds that can be used as partners for these reactions (Scheme 43). A copper catalyst was used to cyclize propiolates 120 with azomethine imines. A selection of bases was screened and the best ee (74%) was obtained with DiPEA at -20 oC.100
When arylacetylenes 121 were used with AgHMDS and racemic BINAP as catalyst in THF at 40 oC, the 5,7-substituted product 122 was formed. Excellent yields were obtained for aromatic azomethine imines and arylacetylenes. An asymmetric version of this reaction was designed, and CuHMDS with (S)-DIP-BINAP was used to generate excellent yields and ee-s for aromatic and aliphatic alkynes and azomethine imines. It was postulated that Cu-acetylenide forms in the reaction, which adds to azomethine imine and leads to a subsequent intramolecular cyclization. Interestingly, the use of BOX ligands lead to an inversion of chemoselectivity, giving 5,6-disubstituted products 123 (Scheme 44).101
In a final example of [3+2] cycloadditions, allenes were reacted with azomethine imines. The use of ethyl allenoate 124 in conjunction with aromatic azomethine imines in a thermal process, generated 125 in 73-92% yields.102 Similarly Au(I) catalysis, lead to the formation of 127 when employing allenyl amines 126 as starting materials in reaction with azomethine imines. It was found that cationic Ph3PAuOTf is the best catalyst, and that when used in DCM for wide variety of aromatic aldehyde derived azomethine imines along with allenyl amines bearing an electron-withdrawing group on nitrogen. Yields are usually good to excellent and good dr were achieved (Scheme 45).103
Cyclizations with C-N cyclic azomethine imines
Enals 128 were used for [3+2] cycloadditions with C-N cyclic azomethine imines by Maruoka and coworkers. A catalytic system of Ti(Oi-Pr)4 (10%) with (S)-BINOL gave the best exo:endo ratio (>95:1) and 92% ee of 129. It was determined by screening that substitutions on the aryl moiety of azomethine imine were tolerated and that aromatic, aliphatic and cyclic unsaturated aldehydes are good partners in this reaction. The enantiomeric excess is affected by the substitution in the 8-position of the azomethine imine, additionally it was found that unsubstituted acrylaldehyde lead to reduced ee compared to other groups. The diastetreoselectivity was affected negatively by having a methyl group in the 7-position in comparison with hydrogen (Scheme 46, right).104
Following another investigation by Maruoka and coworkers, it was determined that vinyl tert-butyl ether is also a suitable candidate for cycloadditions. With catalyst 130, the reaction proceeded with a variety of azomethine imines to provide 131 in excellent yields and ee-s. Although a different catalyst was employed, (E)-N-allylidenepyrrolidin-1-amine followed the same pathway, but with a much reduced exo:endo ratio compared to vinyl ether (Scheme 46, left).105
Finally, allenes have also been used for cyclization.103 The heating of β’-arylsubstituted allenoates 132 with azomethine imines in i-PrOH gave products 133 in very good yields and dr-s ranging from 81:19 to 92:8. Furthermore, screening showed that substitutions of the aryl moiety of the azomethine imine also were tolerated (Scheme 47).
Tandem reactions
Very recently the formation of β-methylene-β-silyloxy-β-amido-α-diazoacetates 134 from azomethine imines was achieved by using Lewis acid catalysis. These reactions proceeded with excellent yields for a variety of azomethine imines derived from aromatic aldehydes. Some decreases in yields were observed for non-aromatic R substituents (Scheme 48). It was observed that in some cases a 1,2-migration occurred, giving rise to the formation of 6-membered rings, provided that 134 included a suitably substituted quaternary carbon in the 5-position. The migration reactions were highly dependent on the catalyst employed, as can be seen in Scheme 47 (C-C migration with formation of 135, O-C migration with formation of 136 or N-C migration with formation of 137). Firstly, Rh2(TFA)4 and Rh2(cap)4 gave the C-C migration and 135 as major product. Secondly, Rh2(esp)2 furnished O-C migration. Finally, Cu catalysts showed themselves superior for the N-C migration: while Rh catalysts always gave a mixture of products (in 88:12 to 81:19 135:137 ratio), CuPF6 gave N-C products 136 only (regardless of substituents).106
The chemoselectivity depends on the phosphane catalyst employed and the structure of allenoate 138, when they are used in cyclizations with C-N cyclic azomethine imines. It was shown that with PMe3 as catalyst, [3+2] cycloaddition is preferable, albeit in lower yields of 13 for aromatic allenoates, and PBu3 favors [3+3] cycloaddition with aromatic allenoates (Scheme 49 and Table 2).107 An analogous trend is observed in another report with N-N cyclic azomethine imines.108
Cycloaddition was observed for β’-unsubstituted phenyl or alkyl enoates in a [3+2] manner to give 141. Notably the presence of a second -CO2Et group in the allene lead to a change in reactivity based on the catalyst's alkyl chain length: with PBu3, [3+4] cycloaddition to 143-144 was preferred, whereas with PMe3 [3+2] and [3+3] cycloadditions proceeded with same efficiency. Whichever catalyst was employed, for all reactions a mixture of products were obtained (Scheme 50 and Table 3).
Finally, when PCy3 was used as catalyst, a [3+2+3] cycloaddition was observed, with ethyl allenoate giving mixtures of two isomeric products 145 in very good combined yields (Scheme 51).
12. [3+3] AND OTHER CYCLIZATIONS OF AZOMETHINE IMINES TO FORM LARGER CYCLES
6-membered rings from N-N cyclic azomethine imines
Chan and Scheidt109 showed that 2-enals with N-heterocyclic carbenes (NHC) form Breslow ene-intermediate, which can add to the azomethine imine double bond and after a subsequent cyclization form a hexahydro-1H-pyrazolo[1,2-a]pyridazin-1-one core 147. It was necessary to employ 20 mol % of the NHC formed from 146 and DBU in boiling DCM to conduct the reaction. Short reaction times were sufficient to generate products 147 with excellent diastereoselectivity (dr >20:1 in all cases). Different azomethine imines derived from aromatic aldehydes are tolerated, and a variety of enals could be used in this reaction, though non-aromatic ones give rise to lower yields (Scheme 52).
Research conducted by Shintani and Hayashi110 showed that the trimethylenemethane generated from 148 is a suitable partner for [3+3] cycloadditions with azomethine imines in presence of Pd-catalysts. It was found that Pd(PPh3)4 in DCM was the best catalytic system, generating 7-methylene-5-phenylhexahydro-1H-pyrazolo[1,2-a]pyridazin-1-one 149 in 81% yield. Different azomethine imines derived from aromatic aldehydes made for good substrates (Scheme 53).
Toste et al.111 demonstrated the use of Au(III)-catalysts in the [3+3]-cyclization of propargyl esters 150 (Scheme 54). After a 1,2-shift and the formation of carbenoid intermediate from propargyl ester, reaction with azomethine imine takes place, giving rise to 151. Following screening, it was found that PicAuCl2 in DCM at 0 oC proved to be the best catalytic system for this reaction. Reactions occurred between 2-methylbut-3-yn-2-yl benzoate and a variety of azomethine imines, allowing for different substitution on pyrazolidin-3-one ring, for aromatic aldehydes based azomethine imines.
Diazo compounds could be used as partners in cycloadditions with azomethine imine, if a Rh-catalyst is utilized. Enoldiazoacetates 152 with Rh2(OAc)4 generate carbenoid intermediates which are reactive towards nucleophilic side of the dipole. If a carbene addition occurs, the N-N bond cleavage furnishes diimine compounds. Should the vinylogous addition to azomethine imine take place, the cyclization to 153 occurs. Aryl and ester substitution on the position 5 of the azomethine imine favors cycloaddition path. These reactions occur with excellent yields for aryl R1 groups, whereas cyclohexyl leads to a drop in yield implying that electronics are more at play than sterics (Scheme 55, top).112
If a diazoketone 154 is used, then a formal [3+2+1] cyclization happens.113 In this case it is proposed that the Rh catalyst acts as a Lewis acid on the azomethine imine, rather than generating a carbene from the diazoketone. Then a stepwise diazoketone addition and N2 extrusion occurs, to finally generate the 6-membered cyclization product 155. The reaction is applicable to different azomethine imines furnishing products in good yields and with very good dr (Scheme 55, bottom).
6-membered rings from N-C cyclic azomethine imines
Charette et al.114 showed that aromatic C-N cyclic azomethine imines could be reacted with 1,1-cyclopropane diesters 156 to give [3+3] cycloaddition products. Using Ni(ClO4)2 as Lewis acid catalyst, a selection of substituted cyclopropanes reacted with azomethine imine, giving the fused tricyclic products 157. The transfer of chirality doesn’t occur from the cyclopropanes. It is proposed instead that the diester chelates to the Ni-catalyst and then the nuclephilic end of the azomethine imines dipole attacks the cyclopropane, after which, the obtained enol cyclizes with imine function. However, when a BOX ligand was used, a strereoselective process takes place (Scheme 56).
The [5+1] cycloaddition can occur between N-C cyclic N’-acyl azomethine imines and isocyanides.115 This transformation doesn’t require catalysts, as one of the resonance forms of azomethine imine could be considered as an “isocyanophile”. The products 158 are obtained in good to excellent yields with different aliphatic and aromatic isocyanides and substitution on benzoate and 3,4-dihydroisoquinoline are tolerated. Azomethine imine (even without a fused aromatic ring) also react readily and N-N cyclic azomethine imines are not reactive in this transformation (Scheme 57).
13. OTHER CYCLIZATIONS
Hydrazones were reported to cyclize with alkenes to yield pyrazolidines 159-160.116 In this case, chirality was induced by using binol-based N-trifylphosphoramide 161 as a catalyst. This reaction tolerates both aromatic and aliphatic hydrazones as well as different alkenes, to give good ee-s and syn:anti ratios (Scheme 58).
Ketenes can also react with azo-compounds to give cyclic hydrazines. Interestingly, when ferrocene derivative 162 was used as catalyst, an enantioselective [2+2] cycloaddition occurs, giving rise to products 163.117 On the other hand, with the NHC-catalyst 164, a [4+2] cycloaddition occured, producing 166. Finally, a similar catalyst 165 gave the opposite enantiomer of the product 166 (Scheme 59).118
Recently a cationic [2+2] cycloaddition was reported for hydrazines.119 Cycloaddition substrate 167 was prepared by the double alkylation of hydrazine with allylic bromides, using NaH for deprotonation. Furthermore, the reaction proceeded upon addition of Lewis or Brønsted acids, with SnCl4 being the most effective, leading to the desired cyclic product 168 as single diastereomer (Scheme 60).
14. OTHER TRANSITION METAL CATALYZED CYCLIZATIONS
In Gagosz’ group a protocol for the Au(I)-catalyzed arylation of hydrazine containing substrates was developed (Scheme 61).120 The substrate synthesis started with phenyl-hydrazine 169, which was protected, then oxidized with MnO2 to azo-compound 170. Subsequently it was subjected to a Grignard reagent, followed by propargylation. The obtained hydrazines 171 were exposed to a cationic Au(I) catalyst (in 4 mol % loading) to furnish exo-methylene tetrahydrocinnolines 172 in excellent yields and in short reaction times. This reaction is applicable for differently substituted aromatics, whereas meta-substituted compounds give 1:1 mixture of the 2 regioisomers 173 and 174and ortho-substitution results in the mixture of exo and endo products 175 and 176 (Figure 7).
15. SUMMARY
In this review we aimed to summarize methods for the synthesis of endocyclic hydrazines starting from acyclic hydrazines and azo-compounds. Of particular interest, section 8 shows methods of enantioselective synthesis of piperazic acid, which is an important building block for the synthesis of biologically active molecules. Additionally, the application of transition-metal catalysis to hydrazine chemistry was highlighted. And finally, recent advances in the field of azomethine imines were reviewed.
16. ACKNOWLEDGEMENTS
This work was supported by Estonian Science Foundation (Grant nr. 8794), the European Regional Development Fund (Center of Excellence ‘‘Mesosystems: Theory and Applications’’, TK114) and the Estonian Science Targeted Project no. SF0180032s12. We would like to thank Alban Cadu (Uppsala University, Sweden) for proof-reading the manuscript.
References
1. K. Frank, J. Bucher, J. Haseman, S. Eustis, and J. Huff, Cancer Science, 1995, 86, 252. CrossRef
2. C. Cucurou, J. P. Battioni, D. C. Thang, N. H. Nam, and D. Mansuy, Biochemistry, 1991, 30, 8964. CrossRef
3. T. Szucs, Drugs, 1991, 41, 18. CrossRef
4. E. M. Kosower, A. E. Radkowsky, A. H. Fairlamb, S. L. Croft, and R. A. Nea, Eur. J. Med. Chem., 1995, 30, 659. CrossRef
5. G. Varvounis, Y. Fiamegos, and G. Pilidis, Adv. Heterocycl. Chem., 2001, 80, 75. CrossRef
6. L. A. Paquette, M. Duan, I. Konetzki, and C. Kempmann, J. Am. Chem. Soc., 2002, 124, 4257; CrossRef K. C. Nicolaou, J. Xu, F. Murphy, S. Barluenga, O. Baudoin, H. Wei, D. L. F. Gray, and T. Ohshima, Angew. Chem. Int. Ed., 1999, 38, 2447. CrossRef
7. J. Wagner, L. M. M. Cabrejas, C. M. Crossmith. C. Papageorgiou, F. Senia, D. Wagner, J. France, and S. P. Nolan, J. Org. Chem., 2000, 65, 9255. CrossRef
8. K. L. Hale, L. Lazarides, and J. Cai, Org. Lett., 2001, 3, 2927. CrossRef
9. K. L. Hale, S. Manaviazar, J. H. George, M. A. Alters, and S. M. Dalby, Org. Lett., 2009, 11, 733. CrossRef
10. R. Matunas, A. J. Lai, and C. Lee, Tetrahedron, 2005, 61, 6298. CrossRef
11. U. Mäeorg and U. Ragnarsson, Tetrahedron Lett., 1998, 39, 681; CrossRef U. Mäeorg, L. Grehn, and U. Ragnarsson, Angew. Chem. Int. Ed., 1996, 108, 2626; CrossRef U. Ragnarsson, B. Fransson, and L. Grehn, J. Chem. Soc., Perkin Trans. 1, 2000, 1405; CrossRef O. Tšubrik and U. Mäeorg, Org. Lett., 2001, 3, 2297; CrossRef G. Lawton, C. J. Moody, C. J. Pearson, and D. J. Williams, J. Chem. Soc., Perkin Trans. 1, 1987, 885; CrossRef K. G. Meyer, Synlett, 2004, 2355; CrossRef N. Sakamoto, E. Miyazawa, and Y. Kikugawa, Synth. Commun., 2003, 33, 87. CrossRef
12. D. Bonnet, J.-F. Margathe, S. Radford, E. Pflimlin, S. Riché, P. Doman, M. Hibert, and A. Ganesan, Comb. Sci., 2012, 14, 323; CrossRef J.-G. Roveda, C. Clavette, A. D. Hunt, S. Gorelsky, C. J. Whipp, and A. M. Beauchemin, J. Am. Chem. Soc., 2009, 131, 8740; CrossRef C. Sund, O. Belda, N. Borkakoti, J. Lindberg, D. Derbyshire, L. Vrang, E. Hamelink, C. Åhgren, E. Woestenenk, K. Wikström, A. Eneroth, E. Lindström, and G. Kalayanov, Bioorg. Med. Chem. Lett., 2012, 22, 6721; CrossRef H. L. Lochte, J. A. Noyes, and J. R. Bailey, J. Am. Chem. Soc., 1922, 44, 2556; CrossRef R. Renaud and L. C. Leitch, Can. J. Chem., 1954, 32, 545; CrossRef J. Gante, Synthesis, 1989, 405. CrossRef
13. R. L. Hinman, J. Am. Chem. Soc., 1956, 78, 1645; CrossRef G. Verardo, N. Toniutti, and A. G. Giumanini, Can. J. Chem., 1998, 76, 1180. CrossRef
14. A. M. Johns, Z. Liu, and J. F. Hartwig, Angew. Chem. Int. Ed., 2007, 46, 7259; CrossRef S. Tšupova and U. Mäeorg, Org. Lett., 2013, 15, 3381. CrossRef
15. P. Nun, C. Martin, J. Martinez, and F. Lamaty, Tetrahedron, 2011, 67, 8187. CrossRef
16. U. Mäeorg, T. Pehk, and U. Ragnarsson, Acta Chem. Scand., 1999, 53, 1127. CrossRef
17. L. K. Rasmussen, J. Org. Chem., 2006, 71, 3627. CrossRef
18. Y. Garcia-Ramos, C. Proulx, and W. D. Lubell, Can. J. Chem., 2012, 90, 985. CrossRef
19. N. Brosse, M.-F. Pinto, and B. Jamart-Gregoire, Eur. J. Org. Chem., 2003, 4757. CrossRef
20. D. G. Dunford, F. Chaudhry, B. Kariuki, D. W. Knight, and R. C. Wheeler, Tetrahedron Lett., 2012, 53, 7006; CrossRef U. Ragnarsson, L. Grehn, J. Koppel, O. Loog, O. Tšubrik, A. Bredikhin, U. Mäeorg, and I. Koppel, J. Org. Chem., 2005, 70, 5916. CrossRef
21. I. Koppel, J. Koppel, F. Degerbeck, L. Grehn, and U. Ragnarsson, J. Org. Chem., 1991, 56, 7172. CrossRef
22. C. G. Overberger and M.-S. Chi, J. Org. Chem., 1981, 46, 303; CrossRef C. G. Overberger and T. F. Merkel, J. Org. Chem., 1981, 46, 442. CrossRef
23. D. E. Wilkinson, B. E. Thomas, D. C. Limburg, A. Holmes, H. Sauer, D. T. Ross, R. Soni, Y. Chen, H. Guo, P. Howorth, H. Valentine, D. Spicer, M. Fuller, J. P. Steiner, G. S. Hamilton, and Y.-Q. Wu, Bioorg. Med. Chem., 2003, 11, 4815; CrossRef K. Wang, W. Wang, Q. Wang, and R. Huang, Lett. Org. Chem., 2008, 5, 383; CrossRef S. P. East, A. Ayscough, I. Toogood-Johnson, S. Taylor, and W. Thomas, Bioorg. Med. Chem. Lett., 2011, 21, 4032; CrossRef C. Hemmerlin, M. T. Cung, and G. Boussard, Tetrahedron Lett., 2001, 42, 5009; CrossRef T. Toya, K. Yamaguchi, and Y. Endo, Bioorg. Med. Chem., 2002, 10, 953; CrossRef M. Kamata, T. Yamashita, A. Kina, M. Tawada, S. Endo, A. Mizukami, M. Sasaki, A. Tani, Y. Nakano, Y. Watanabe, N. Furuyama, M. Funami, N. Amano, and K. Fukatsu, Bioorg. Med. Chem. Lett., 2012, 22, 4769. CrossRef
24. Y. Ju and R. Varma, J. Org. Chem., 2006, 71, 135. CrossRef
25. R. Di Fabio, G. Araldi, D. Baraldi, A. Cugola, D. Donati, P. Gastaldi, S. A. Giacobbe, F. Micheli, and G. Pentassuglia, Il Farmaco, 2001, 56, 791. CrossRef
26. J. H. Ahn, M. S. Shin, M. A. Jun, S. H. Jung, S. K. Kang, K. R. Kim, S. D. Rhee, N. S. Kang, S. J. Kim, S.-K. Sohn, S. G. Kim, and J. O. Lee, Bioorg. Med. Chem. Lett., 2007, 17, 2622; CrossRef J. B. Braizer, J. L. Cavill, R. L. Elliott, G. Evans, T. J. K. Gibbs, I. L. Jones, J. A. Platts, and N. C. O. Tomkinson, Tetrahedron, 2009, 65, 9961. CrossRef
27. A. Bredihhin and U. Mäeorg, Org. Lett., 2007, 9, 4975; CrossRef A. Bredihhin, U. Groth, and U. Mäeorg, Org. Lett., 2007, 9, 1097; CrossRef A. Bredihhin and U. Mäeorg, Tetrahedron, 2008, 64, 6788. CrossRef
28. O. Lebedev, A. Bredihhin, S. Tšupova, and U. Mäeorg, Tetrahedron, 2009, 65, 5438. CrossRef
29. M. Muehlebach, M. Boeger, F. Cederbaum, D. Cornes, A. A. Friedmann, J. Glock, T. Niderman, A. Stoller, and T. Wagner, Bioorg. Med. Chem., 2009, 17, 4241. CrossRef
30. F. J.-J. Bihel, M. Hellal, and J.-J. Bourguignon, Synthesis, 2007, 3791. CrossRef
31. A. Nakhai and J. Bergman, Tetrahedron, 2009, 65, 2298. CrossRef
32. L. C. Raiford and W. J. Peterson, J. Org. Chem., 1937, 1, 544. CrossRef
33. M. Kaname, M. Yamada, S. Yoshifuji, and H. Sashida, Chem. Pharm. Bull., 2009, 57, 49. CrossRef
34. W. Wan, J. Hou, H. Jiang, Y. Wang, S. Zhu, D. Deng, and J. Hao, Tetrahedron, 2009, 65, 4212; CrossRef V. T. Humme, S. G. Konda, K. Hasanzadeh, and P. D. Lokhande, Chinese Chem. Lett., 2011, 22, 1435. CrossRef
35. N. Haider and A. Wobus, ARKIVOC, 2008, ii, 16.
36. D. A. Rudenko, S. N. Shurov, M. I. Vakhrin, V. I. Karmanov, and Y. A. Shchurov, Chem. Heterocycl. Compd., 2013, 48, 1522. CrossRef
37. P. Budhlakoti, Y. Kumar, A. Verma, and S. Alok, Int. J. Pharm. Sci. Res., 2013, 4, 1524.
38. W. Wan, J. Hou, H. Jiang, Y. Wang, S. Zhu, D. Deng, and J. Hao, Tetrahedron, 2009, 65, 4212. CrossRef
39. E. C. Taylor and J. S. Hinkle, J. Org. Chem., 1987, 52, 4107. CrossRef
40. A. V. Karpenko, S. I. Kovalenko, and O. V. Shishkin, Tetrahedron, 2009, 65, 5964. CrossRef
41. M. Rimaz and J. Khalafy, ARKIVOC, 2010, ii, 110.
42. J.-C. Hannachi, J. Vidal, J.-C. Mulatier, and A. Collet, J. Org. Chem., 2004, 69, 2367. CrossRef
43. E. Moreno-Clavijo, A. T. Carmona, A. J. Moreno-Vargas, M. A. Rodríguez-Carvajal, and I. Robina, Bioorg. Med. Chem., 2010, 18, 4648. CrossRef
44. Y. Aoyagi, Y. Saitoh, T. Ueno, M. Horiguchi, K. Takeya, and R. M. Williams, J. Org. Chem., 2003, 68, 6899. CrossRef
45. J.-C. Monbaliu and J. Marchand-Brynaert, Synthesis, 2009, 1876; CrossRef D. L. Comins, J. T. Kuethe, T. M. Miller, F. C. Fevrier, and C. A. Brooks, J. Org. Chem., 2005, 70, 5221. CrossRef
46. S. Cohen, R. Zand, and C. Steel, A Novel Reduction, 1961, 83, 2895.
47. Z. Zatan, L. Lazar, and F. Fülöp, Curr. Org. Chem., 2005, 9, 357. CrossRef
48. A. Perez Luna, M.-A. Ceschi, M. Bonin, L. Micoulin, H.-P. Husson, S. Gougeon, G. Estenne-Bouhtou, B. Marabout, M. Sevrin, and P. George, J. Org. Chem., 2002, 67, 3522. CrossRef
49. T. Tschamber, C. J. Craig, M. Muller, and J. Streith, Tetrahedron, 1996, 37, 6201. CrossRef
50. M. Bols, R. G. Hazell, and I. B. Thomsen, Chem. Eur. J., 1997, 3, 940. CrossRef
51. S. U. Hansen and M. Bols, J. Chem. Soc., Perkin Trans. 1, 1999, 3323; CrossRef X. Liang and M. Bols, J. Org. Chem., 1999, 64, 8485. CrossRef
52. A. Lohse, H. Jensen, P. Bach, and M. Bols, J. Chem. Soc., Perkin Trans. 1, 2000, 659. CrossRef
53. B. T. Gillis and P. E. Beck, J. Org. Chem., 1962, 1947.
54. I. Thomsen, B. V. Ernholt, and M. Bols, Tetrahedron, 1997, 53, 9357. CrossRef
55. K. Okamoto, K. Shiodera, T. Kawamura, and K. Ogino, J. Phys. Org. Chem., 2008, 21, 257. CrossRef
56. T. Sheradsky and R. Moshenberg, J. Org. Chem., 1985, 50, 5604. CrossRef
57. C. L. Molina, C. P. Chow, and K. J. Shea, J. Org. Chem., 2007, 72, 6816. CrossRef
58. D. Chaiyaveij, L. Cleary, A. S. Batsanov, T. B. Marder, K. J. Shea, and A. Whiting, Org. Lett., 2011, 13, 3442. CrossRef
59. S. M. Ogbomo and D. J. Burnell, Org. Biomol. Chem., 2006, 4, 3838. CrossRef
60. A. Kiriazis, T. Rüffer, S. Jäntti, H. Lang, and J. Yli-Kauhaluoma, J. Comb. Chem., 2007, 9, 263. CrossRef
61. H. Yamamoto and M. Kawasaki, Bull. Chem. Soc. Jpn., 2007, 80, 595. CrossRef
62. B. Liu, K.-N. Li, S.-W. Luo, J.-Z. Huang, H. Pang, and L.-Z. Gong, J. Am. Chem. Soc., 2013, 135, 3323. CrossRef
63. S. F. Nelsen, S. C. Blackstock, and T. B. Frigo, J. Am. Chem. Soc., 1984, 106, 336. CrossRef
64. S. F. Nelsen, T. B. Frigo, and Y. Kim, J. Am. Chem. Soc., 1989, 111, 5387. CrossRef
65. S. Sommer, Tetrahedron Lett., 1977, 1, 117. CrossRef
66. N. A. M. Pereira, A. Lemos, A. C. Serra, and T. M. V. D. Pinho e Melo, Tetrahedron Lett., 2013, 54, 1553. CrossRef
67. A. Kotschy, D. M. Smith, and A. C. Benyei, Tetrahedron Lett., 1998, 39, 1045. CrossRef
68. J. Armbruster, S. Grabowski, T. Ruch, and H. Prinzbach, Angew. Chem. Int. Ed., 1998, 37, 2242. CrossRef
69. J. M. Ellis and S. B. King, Tetrahedron, 2002, 43, 5833. CrossRef
70. C. Alvarez-Ibarrra, A. G. Csaky, and C. Gomes de la Oliva, J. Org. Chem., 2002, 67, 2789. CrossRef
71. H. H. Jensen, A. Jensen, R. G. Hazell, and M. Bols, J. Chem. Soc., Perkin Trans. 1, 2002, 1190. CrossRef
72. K. Depew, T. M. Kamenecka, and S. J. Danishefsky, Tetrahedron Lett., 2000, 41, 289. CrossRef
73. K. J. Hale, J. Cai, V. Delisser, S. Manaviazar, S. A. Peak, G. S. Bhatia, T. C. Collins, and N. Jogiya, Tetrahedron, 1996, 52, 1047. CrossRef
74. R. A. Coats, S.-L. Lee, K. A. Davis, K. M. Patel, E. K. Rhoads, and M. H. Howard, J. Org. Chem., 2004, 69, 1734. CrossRef
75. Y. Henmi, K. Makino, Y. Yoshitomi, O. Hara, and Y. Hamada, Tetrahedron: Asymmetry, 2004, 15, 3477. CrossRef
76. K. Makino, Y. Henmi, M. Terasawa, O. Hara, and Y. Hamada, Tetrahedron Lett., 2005, 46, 555. CrossRef
77. M. Kaname, K. Yoshinaga, Y. Arakawa, and S. Yoshifuji, Tetrahedron Lett., 1999, 40, 7993. CrossRef
78. M. Kaname, Y. Arakawa, and S. Yoshifuji, Tetrahedron Lett., 2001, 42, 2713. CrossRef
79. J.-C. Monbaliu and J. Marchand-Brynaert, Tetrahedron Lett., 2008, 49, 1839. CrossRef
80. H. Al-Badri, E. About-Jaudet, and N. Collignon, Tetrahedron Lett., 1996, 37, 2951. CrossRef
81. J. Tae and D.-W. Hahn, Tetrahedron Lett., 2004, 45, 3757. CrossRef
82. S. Tšupova, O. Lebedev, and U. Mäeorg, Tetrahedron, 2012, 68, 1011. CrossRef
83. I. Duttagupta, K. Goswami, and S. Sinha, Tetrahedron, 2012, 68, 8347. CrossRef
84. T. J. Donohoe, J. F. Bower, J. A. Basutto, L. P. Fishlock, P. A. Procopiou, and C. K. A. Callens, Tetrahedron, 2009, 65, 8969. CrossRef
85. V. De Matteis, F. L. van Delft, J. Tiebes, and F. P. J. T. Rutjes, Synlett, 2008, 351. CrossRef
86. A. Lim, J. H. Choi, and J. Tae, Tetrahedron Lett., 2008, 49, 4882. CrossRef
87. Y. J. Kim and D. Lee, Org. Lett., 2004, 6, 4351. CrossRef
88. L. M. M. Cabrejas, S. Rohrbach, D. Wagner, J. Kallen, G. Zenke, and J. Wagner, Angew. Chem. Int. Ed., 1999, 38, 2443. CrossRef
89. J. G. Schantl, Adv. Heterocycl. Chem., 2010, 99, 185. CrossRef
90. C. Clavette, W. Gan, A. Bongers, T. Markiewicz, A. B. Toderian, S. I. Gorelsky, and A. M. Beauchemin, J. Am. Chem. Soc., 2012, 134, 16111; CrossRef W. Gan, P. J. Moon, C. Clavette, N. D. Neves, T. Markiewitz, A. B. Toderian, and A. M. Beauchemin, Org. Lett., 2013, 15, 1890. CrossRef
91. H. D. S. Guerrand, H. Adams, and I. Coldham, Org. Biomol. Chem., 2011, 9, 7921. CrossRef
92. T. Hashimoto, H. Kimura, Y. Kawamata, and K. Maruoka, Angew. Chem. Int. Ed., 2012, 51, 7279. CrossRef
93. L. M. Stanley and M. P. Sibi, Chem. Rev., 2008, 108, 2887. CrossRef
94. W. Chen, X.-H. Yuan, R. Li, W. Du, Y. Wu, L.-S. Ding, and Y.-C. Chen, Adv. Synth. Catal., 2006, 348, 1818. CrossRef
95. H. Suga, T. Arikawa, K. Itoh, Y. Okumura, A. Kakehi, and M. Shiro, Heterocycles, 2010, 81, 1669. CrossRef
96. H. Suga, A. Funyu, and A. Kakehi, Org. Lett., 2007, 9, 97. CrossRef
97. K. Tanaka, T. Kato, S. Fujinami, Y. Ukaji, and K. Inomata, Chem. Lett., 2010, 39, 1036. CrossRef
98. F. Shi, R. Mancuso, and R. C. Larock, Tetrahedron Lett., 2009, 50, 4067. CrossRef
99. Y. B. Koptelov and M. V. Sednev, Rus. J. Org. Chem., 2011, 47, 547. CrossRef
100. T. Arai and Y. Ogino, Molecules, 2012, 17, 6170. CrossRef
101. T. Imaizumi, Y. Yamashita, and S. Kobayashi, J. Am. Chem. Soc., 2012, 134, 20049. CrossRef
102. R. Na, H. Liu, Z. Li, B. Wang, J. Liu, M.-A. Wang, M. Wang, J. Zhong, and H. Guo, Tetrahedron, 2012, 68, 2349. CrossRef
103. W. Zhou, X.-X. Li, G.-H. Li, Y. Wu, and Z. Chen, Chem. Commun., 2013, 49, 3552. CrossRef
104. T. Hashimoto, Y. Maeda, M. Omote, H. Nakatsu, and K. Maruoka, J. Am. Chem. Soc., 2010, 132, 4076. CrossRef
105. T. Hashimoto, M. Omote, and K. Maruoka, Angew. Chem. Int. Ed., 2011, 50, 3489. CrossRef
106. X. Xu, Y. Qian, P. Y. Zavalij, and M. P. Doyle, J. Am. Chem. Soc., 2013, 135, 1244. CrossRef
107. C. Jing, T. Na, B. Wang, H. Liu, L. Zhang, J. Liu, M. Wang, J. Zhong, O. Kwon, and H. Guo, Adv. Synth. Catal., 2012, 354, 1023. CrossRef
108. R. Na, C. Jing, Q. Xu, H. Jiang, X. Wu, J. Shi, J. Zhong, M. Wang, D. Benitez, E. Tkatchouk, W. A. Goddard III, H. Guo, and O. Kwon, J. Am. Chem. Soc., 2011, 133, 13337. CrossRef
109. A. Chan and K. A. Scheidt, J. Am. Chem. Soc., 2007, 129, 5334. CrossRef
110. R. Shintani and T. Hayashi, J. Am. Chem. Soc., 2006, 128, 6330. CrossRef
111. N. D. Shapiro, Y. Shi, and F. D. Toste, J. Am. Chem. Soc., 2009, 131, 11654. CrossRef
112. Y. Qian, P. J. Zavalij, W. Hu, and M. P. Doyle, Org. Lett., 2013, 15, 1564. CrossRef
113. X. Xu, X. Xu, P. Y. Zavalij, and M. P. Doyle, Chem. Comm., 2013, 49, 2762. CrossRef
114. C. Perreault, S. R. Goudreau, L. E. Zimmer, and A. B. Charette, Org. Lett., 2008, 10, 689. CrossRef
115. T. Soeta, K. Tamura, and Y. Ukaji, Org. Lett., 2012, 14, 1226. CrossRef
116. M. Rueping, M. S. Maji, H. B. Kücük, and I. Atodiresei, Angew. Chem. Int. Ed., 2012, 51, 12864. CrossRef
117. J. M. Berlin and G. C. Fu, Angew. Chem. Int. Ed., 2009, 48, 192. CrossRef
118. X.-L. Huang, L. He, P.-L. Shao, and S. Ye, Angew. Chem. Int. Ed., 2008, 47, 7048. CrossRef
119. J. Deng, R. P. Hsung, and C. Ko, Org. Lett., 2012, 14, 5562. CrossRef
120. I. D. Jurberg and F. Gagosz, J. Organomet. Chem., 2011, 696, 37. CrossRef