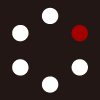
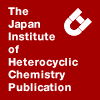
HETEROCYCLES
An International Journal for Reviews and Communications in Heterocyclic ChemistryWeb Edition ISSN: 1881-0942
Published online by The Japan Institute of Heterocyclic Chemistry
e-Journal
Full Text HTML
Received, 25th July, 2013, Accepted, 20th August, 2013, Published online, 23rd August, 2013.
DOI: 10.3987/REV-13-778
■ SYNTHESIS OF 3,7,9- AND 2,6,9-TRIAZABICYCLO[3.3.1]NONANE DERIVATIVES
Ambara R. Pradipta and Katsunori Tanaka*
Biofunctional Synthetic Chemistry Laboratory, RIKEN, 2-1 Hirosawa, Wako, Saitama 351-0198, Japan
Abstract
Due to their interesting chemical and biological properties, nitrogen containing-heterocycles represent indispensable moiety in organic chemistry. From the various types of nitrogen containing-heterocycles, azabicyclic compounds in particular are of considerable importance in many aspects and have attracted attention from wide range of fields, including in the study of natural products, coordination properties, or in the development of biologically and pharmacologically active compounds. This review focuses on the hitherto reported methods for the synthesis of the triazabicyclo[3.3.1]nonane derivatives, the bicyclic compound having two nitrogen atoms at the eight-membered ring and one nitrogen atom at the bridge position, which are less explored in the literature probably due to difficulties in their synthetic accessibility.CONTENTS
1. Introduction
2. Synthesis of 3,7,9-Triazabicyclo[3.3.1]nonanes
3. Synthesis of 2,6,9-Triazabicyclo[3.3.1]nonanes and Epiminodibenzo[b,f][1,5]diazocines
4. Application to Heterocyclic Derivatives and Metal Ligands
5. Summary
1. INTRODUCTION
Molecules having bispidine (3,7-diazabicyclo[3.3.1]nonane) backbone (1) have been intensively studied and considered as an interesting subject in various aspects due to their potential as metal-ligand, chiral organocatalyst, proton sponge, and bioactive properties, e.g., key structural motifs in antiarrhythmic agent, nicotinic acetylcholine receptor, etc.1 Sparteine (2), cytisine (3), and Tröger’s base (4) are representative molecules of this class (Figure 1), which have been widely applied to both organic and medicinal chemistry.2 The analogues of bispidine (1) having one additional nitrogen atom at the bridge position, i.e., 3,7,9-triazabicyclo[3.3.1]-nonanes (5), 2,6,9-triazabicyclo[3.3.1]nonanes (6), and epiminodibenzo[b,f][1,5]diazocines (7), have rarely been disclosed in the literature, although they could be used as the new types of metal-ligands as well as the core skeletons in developing the bioactive and pharmacologically active compounds.
Straightforward synthesis of the triazabicyclo[3.3.1]nonane derivatives is still a challenging task, and so far there are only a few methods have been reported. In this review, we will summarize the synthetic methods of triazabicyclo[3.3.1]nonanes having nitrogen atoms at the 3,7,9- and 2,6,9-positions (derivatives 5, 6, and 7).
2. SYNTHESIS OF 3,7,9-TRIAZABICYCLO[3.3.1]NONANES
Revesz and co-workers have reported the synthesis of 3,7,9-triazabicyclo[3.3.1]nonane derivatives (Scheme 1).3 The starting materials, ethyl acrylate (8) and N,N’-dibromobenzenesulfonamide (9) were reacted to generate the mixture of meso- and racemic isomers. The pure meso-dibromide (10) was obtained in 25% yield after filtration through a silica gel pad, followed by slow crystallization from toluene/hexane. When treated with benzylamine (11), the piperazine (12) was observed in 60% yield. Selective reduction of the diester by LiAlH4, followed by treatment with thionylchloride in DMF at 0 °C to room temperature for 1 h, yielded cis-dichloride (13) in 62% for two steps. Refluxing cis-dichloride (13) in xylene with 3 eq of benzylamine (11) resulted in the clean conversion to the 3,9-dibenzyl-7(phenylsulfonyl)-3,7,9-triazabicyclo[3.3.1]nonane (14) in 75% yield. Alternatively, when this reaction was performed in benzylamine as solvent at 180 °C for 15 min, the desired compound 14 was isolated as the major product in 61% yield, interestingly, together with 10% of 3,6,8-triazabicyclo[3.2.2]nonane (15).
Although the synthesis of 3,9-dibenzyl-7(phenylsulfonyl)-3,7,9-triazabicyclo[3.3.1]nonane (14) from ethyl acrylate (8) required five steps, this method allowed the functionalization of the nitrogen substituents (Scheme 2). Thus, sulfonamide group was easily removed by reduction with Red-Al®, producing 3,9-dibenzyl-3,7,9-triazabicyclo[3.3.1]nonane (16) in 78% yield. Reductive methylation of the free amine, followed by debenzylation gave 17 in 75% for two steps. A selective 4-fluorobenzylation was successfully performed at the less hindered nitrogen atom (N-3). Alternatively, the treatment of 17 with Boc2O, followed by reaction with 4-fluorobenzyl chloride, gave the N9-alkylated product 20 in 73% yield.
3. SYNTHESIS OF 2,6,9-TRIAZABICYCLO[3.3.1]NONANES AND EPIMINODIBENZO[b,f][1,5]DIAZOCINES
The first synthesis of substituted 2,6,9-triazabicyclo[3.3.1]nonane was described by Katritzky and co-workers in 1979 (Scheme 3).4 They found that dropwise addition of crotonaldehyde (21) to aqueous methylamine (22) at 0 °C and continuous stirring for two days at this temperature produced the 2,3,6,7,9-pentamethyl-2,6,9-triazabicyclo[3.3.1]nonane (23). Although, they did not discuss the reaction mechanism, the conformation of 23 was determined as that shown in Scheme 3, based on the extensive NMR analysis.
Several years after Katritzky’s synthesis, Shim and co-workers isolated a dioxo derivative of 2,6,9-triazabiclyclo[3.3.1]nonane, when they attempted to synthesize the 4-pyrimidone compounds through the thermolysis of β-aminocrotonamide (Scheme 4).5 During the thermolysis of β-aminocrotonamide (24), a mixture of two compounds (70% yields), which were then identified as 2,6-dimethyl-4-pyrimidon-2-ylacetamide (25) and 1,5-dimethyl-2,6,9-triazabiclyclo[3.3.1]nonane-3,7-dione (26), were produced in 2 : 3 ratio, through the intermediate (27). The later was purified by recrystallization from methanol of the initially product mixture, whereas the former was purified by silica gel column chromatography of the remaining mother liquor.
The two intermediates can be converted to each other by heating at 110 °C in dimethyl sulfoxide. Both compounds could also be transformed to 2,6-dimethyl-3H-4-pyrimidone (28) by heating at 200 °C for 10 min in dimethyl sulfoxide.
Another method for the synthesis of the compound (26) was reported by Abonia and co-workers.6 In contrast with Shim’s method that utilized harsh conditions, their method proceeded at room temperature, even though it required a longer reaction time (Scheme 5). Initially, they found that the ammonolysis of alkyl acetoacetates (30) with excess aqueous 15% NH3 at room temperature for 20 min led to the formation of methyl 3-aminocrotonates (31) in 94% yield. Then they found that, when the compound 31 was allowed to stand at room temperature for four weeks, it slowly converted into a new compound, which was identified as 1,5-dimethyl-2,6,9-triazabiclyclo-[3.3.1]nonane-3,7-dione (26) in 23% yield.
As the reaction mechanism, after the initial formation of methyl 3-aminocrotonate (31), the reaction slowly gave intermediate β-amino-crotonamide (24), a compound which is also utilized in the Shim’s reaction (Scheme 4). However, they did not observe the formation of 2,6-dimethyl-4-pyrimidon-2-ylacetamide (see structure 25 in Scheme 4) as the intermediate, which was isolated as the stable compound. Based on this result, they proposed that the reaction could proceed through the double Michael addition of NH3 to diazocine intermediate (35), which was produced by the dimerization of 24 through bis-N-acylation.
The first synthesis of epiminodibenzo[b,f][1,5]diazocine (38) was reported by Albert and Yamamoto in 1968.7 They reported that reaction between 2-aminobenzaldehyde (36) and aqueous ethylamine (37) gave 38 in 59% yield (Scheme 6). Despite the moderate yield of the reaction, the following reports on the synthesis of the epiminodibenzo[b,f][1,5]diazocine compounds are indeed simply the variations of this reaction.
Ukhin and co-workers have described a method for synthesizing the epiminodibenzo[b,f][1,5]diazocine from o-tosylamino-benzaldehyde (39) and primary amines (40) (Scheme 7).8 The scope of the reaction was evaluated by using the various primary amines. Their study showed that condensation reaction with α-amino acids did not proceed, while utilization of γ-aminobutyric acid (40a), β-alanine (40b), p-aminophenylthioacetic acid (40c), and 4-aminobenzoic acid (40d) gave the corresponding epiminodibenzo[b,f][1,5]diazocine products (41a-d) in moderate yields.
Utilization of o-mesylaminobenzaldehyde (44) instead of o-tosylaminobenzaldehyde (39) did not affect the reactivity profile and provided the epiminodibenzo[b,f][1,5]diazocine derivatives (Scheme 8).8 Mesylaminobenzaldehyde (44) was readily prepared by mesylation of 2-aminobenzohydrazine (42), followed by McFadyen-Stevens reaction of the resulting 43. Reaction of 44 with various primary amines or ammonium acetate yielded the corresponding cyclized products 45 in moderate yields.
Molina and co-workers reported another variant for the synthesis of epiminodibenzo[b,f][1,5]diazocines by utilizing o-(triphenyl-phosphoranyliden)aminobenzaldehyde (47) as the starting material (Scheme 9).9 Iminophosphorane (47), which was obtained from the azide (46) by the reaction with triphenylphosphine, was surprisingly stable and did not give the bis-imine (48) even when heated at 220 °C, but smoothly provided the corresponding epiminodibenzo[b,f][1,5]diazocine (54) in moderate yields, when treated with various primary amines. They proposed the mechanism of the production of 54, which involves the initial reaction of 47 with the primary amine (49) to give the aniline derivative 51 by loss of triphenylphosphine oxide from 50. Intermediate (51) further reacted with another molecule of iminophosphorane (47) by the same sequences of the reactions to give bis-imine (53). Finally, the acetal formation of 53 led to the stable and rigid molecules (54).
In attempt to synthesize the bromazepam building blocks, Zonta and co-workers noticed that during the preparation of ketimine 55, the reaction mixture is always contaminated by small quantity of the corresponding epiminodibenzo[b,f][1,5]diazocine 59 (Scheme 10).10 A detailed investigation of the reaction mixture revealed that the trace amount of the ketone 57 was responsible for the formation of the dimer. After optimization of the reaction conditions, they found that the addition of 0.06 eq of ketone 57 to the reaction mixture allowed the production of 59 in 72% yield. The role of the ketone 57 in the catalytic process could be attributed to the scavenger of ammonia. The presences of bromine atoms at the aromatic ring in product 59 could permit further structural modification. The reaction could proceed through similar mechanism that proposed by Molina and co-workers (Scheme 9).
Aubé and co-workers reported that Lewis acid catalyzed formation of epiminodibenzo[b,f][1,5]diazocine (65) from (2’-amino-N’-tert-butoxycarbonylbenzylidene)-3-alkenylamines (60) (Scheme 11).11 They reported that the addition of TMSOTf to the starting imines 60 led to the exclusive formation of the desired compounds (65), while utilizing other Lewis acids, i.e., TiCl4, interestingly resulted in the preferential production of hexahydropyridol[1,2-c]quinazolin-6-ones (68).
By treating with TMSOTf, the Boc group of 60 could be fully deprotected to give the corresponding amines (61), which subsequently participated in the condensation reaction as shown in scheme 11A, giving rise to the production of 65. On the other hand, when TiCl4 was used as the milder Lewis acid, the Boc group in 60 could not be fully deprotected, and the alternative aza-Prins-type pathway could dominate (Scheme 11B).
Bergman and co-workers also reported the synthesis of epiminodibenzo[b,f][1,5]diazocine (72) from 2-aminobenzonitrile (69) and Grignard reagent (Scheme 12).12 The 2-aminobenzonitrile (69) reacted with methylmagnesium bromide to produce the intermediate 70, which in turn gave 72 in 41% yield by heating in THF and acidic work-up procedure.
Recently, Tanaka and co-workers unexpectedly found an efficient preparation of the substituted 2,6,9-triazabicyclo[3.3.1]nonanes during their study of new reactivity of N-allyl conjugated imines, for its application to alkaloid synthesis and bioconjugation.13 When methyl fumaraldehydate (73) was simply treated with excess amount of benzylamine in chloroform at room temperature, instead of the expected conjugated benzylimine, a single product (quantitative yield), which by the 1H-NMR analysis, has no vinyl protons, was obtained (Scheme 13). The X-ray crystallographic analysis elucidated the structure of the product, 2,6,9-triazabicyclo[3.3.1]nonane (75), as well as its stereochemistry and conformation. The stereochemistry was shown based on ORTEP data, as shown in Scheme 13.
The experimental data suggested that the extra amine could mediate the conjugate addition of an imino nitrogen to another molecule of imine, namely, by enhancing the nucleophilicity of the nitrogen atom by forming the transient aminoacetal (Scheme 14). Subsequent eight-membered ring-closing and bis-acetal formation under the equibrilium process gave the rigid 2,6,9-triazabicyclo[3.3.1]nonane (75) as the thermodynamically most stable product. The acetal formation was therefore considered to be the rate-limiting step of whole [4+4] process, and hence the triazabicyclononane formation.
The scope of this amine-mediated formal [4+4] reaction has been examined by using variously substituted conjugated aldehydes and primary amines. While acrolein (80), methacrolein (82), and crotonaldehyde (21) can participate in the production of the 2,6,9-triazabicyclo[3.3.1]nonane derivatives in moderate to good yields (Scheme 15A), the primary amines could be restricted to the p-substituted benzylamines and allylamine. n-Propylamine or propargylamine, only gave the corresponding unsaturated imines. Hetero [4+4] reaction could also be achieved (Scheme 15B); Thus, the reaction of benzylimines derived from 73 and 21 with benzylamine stereoselectively produced the compound (85) in 48% yield.
Thus, their preparation method constitutes one of the most efficient routes to the various 2,6,9-triazabicyclo[3.3.1]nonane derivatives under quite mild conditions using extremely simple operations, namely, by simply treating the substituted aldehydes with excess amines at ambient temperature.
4. APPLICATION TO HETEROCYCLIC DERIVATIVES AND METAL LIGANDS
The 2,6,9-triazabicyclo[3.3.1]nonane skeleton has also be tried to incorporate into the natural- or biologically active molecules. Quintela and co-workers observed expectedly that the reaction of 5-amino-3,4-diphenylthieno[2,3-c]pyridazine-6-carbaldehyde (86) as the pharmacologically important molecule, with aromatic primary amines in hot EtOH-AcOH (15 : 1) produced the corresponding imines (Scheme 16A, condition 1).14 However, when the compound (86) was reacted with the aliphatic primary amines under the same conditions, the dimeric compounds containing the 2,6,9-triazabicyclo[[3.3.1]nonanes were isolated in moderate to good yield (Scheme 16B). The use of PTSA or piperidine instead of acetic acid, on the other hand, gave the imine derivatives as the only products regardless of using the substituted anilines or primary amines (Scheme 16A, condition 2).
A proposed mechanism for the conversion of 86 to 90 is shown in Scheme 16B. The initial reaction of the o-aminoaldehyde (86) with the primary amino groups (88) produced the corresponding imine derivatives (87), which underwent further reaction with another molecule of aminoaldehyde (86) to give the key intermediates (89). Subsequent ring-closure provided the desired thieno-heterocyclic 2,6,9-triazabicyclo[3.3.1]nonanes (90).
The substituted 2,6,9-triazabicyclo[3.3.1]nonane derivative could also be designed and synthesized as the new metal-ligand. Thus, Redshaw and co-workers reported that treatment of 2 eq of 2-amino-3,5-dibromobenzaldehyde (91) with o- or m-anthranilic acid in hot ethanol afforded the epiminodibenzo[b,f][1,5]diazocine (96) in 80-90% yield (Scheme 17).15 In the absence of anthranilic acids, 2-amino-3,5-dibromobenzaldehyde (91) is recovered, suggesting that the reaction may proceed through the imine intermediates (94), and not the dibenzo[b,f][1,5]diazocine (92). Then, they synthesized the tungsten complex 97a (53%) and 97b (30%), by reacting the corresponding in situ-generated 96a or 96b with [W(η-C5Me5)Cl4] in hot toluene. The coordination geometry of complexes (97) had been extensively studied by the same group, and thus opened a synthetic application of the 2,6,9-triazabicyclo[3.3.1]nonane derivatives to the metal complexes (93).
5. SUMMARY
Although the triazabicyclo[3.3.1]nonanes containing the nitrogen atoms at 3,7,9- or 2,6,9-positions are very attractive motifs for various synthetic and biological applications, as discussed in this review, there has been reported only one example for the synthesis of the 3,7,9-triazabicyclo[3.3.1]nonanes,3 and twelve examples for those of the 2,6,9-congeners.4-15 In case of the epiminodibenzo[b,f][1,5]-diazocines, the currently available methods all utilize the variations of Albert’s synthesis,7 where the aniline derivatives are used as the starting materials.
Recently, the benzyl bispidine, a carbon analog of the triazabicyclo[3.3.1]nonanes derivatives (see structures 2-4 in Figure 1), could show the comparable catalytic activity to the (-)-spartein (2), toward the ring-opening polymerization of the lactide, when combined with the thiourea co-catalyst.16 The combination of the metal- and organo-catalysts with the diverse structural modification could thus open a new era of the synthetic & coordination chemistry as well as the biological & pharmacological research. The efficient methods for preparing the variously substituted triazabicyclo[3.3.1]nonanes, a structural variant of the bispidine derivatives with additional nitrogen atom, are obviously highly desirable.
ACKNOWLEDGEMENTS
We thank Prof. Koichi Fukase at Osaka University, for motivating us to interest in this class of the compounds. The authors' research cited in this review was supported in part by Grants-in-Aid for Scientific Research from the Japan Society for the Promotion of Science, 22651081, 23681047, and 25560410, by a Research Grant from the Mizutani Foundation for Glycoscience, and by a MEXT Grant-in-Aid for Scientific Research on Innovative Areas “Chemical Biology of Natural Products: Target ID and Regulation of Bioactivity”.
References
1. J. Liu, Z. Yang, Z. Wang, F. Wang, X. Chen, X. Liu, X. Feng, Z. Su, and C. Hu, J. Am. Chem. Soc., 2008, 130, 5654; CrossRef L. Toom, A. Kütt, I. Kaljurand, I. Leito, H. Ottosson, H. Grennberg, and A. Gogoll, J. Org. Chem., 2006, 71, 7155; CrossRef A. A. Jensen, B. Frølund, T. Liljefors, and P. Krogsgaard-Larsen, J. Med. Chem., 2005, 48, 4705; CrossRef P. Comba, M. Kerscher, M. Merz, V. Müller, H. Pritzkow, R. Remenyi, W. Schiek, and Y. Xiong, Chem. Eur. J., 2002, 8, 5750; CrossRef G. L. Garrison, K. D. Berlin, B. J. Scherlag, R. Lazzara, E. Patterson, T. Fazekas, S. Sangiah, C.-L. Chen, F. D. Schubot, and D. van der Helm, J. Med. Chem., 1996, 39, 2559. CrossRef
2. Ö. V. Rúnarsson, J. Artacho, and K. Wärnmark, Eur. J. Org. Chem., 2012, 7015; CrossRef C. Hirschhäuser, C. A. Haseler, and T. Gallagher, Angew. Chem. Int. Ed., 2011, 50, 5162; CrossRef B. T. Smith, J. A. Wendt, and J. Aubé, Org. Lett., 2002, 4, 2577; CrossRef D. Hoppe and T. Hense, Angew. Chem. Int. Ed., 1997, 36, 2282; and references cited therein. CrossRef
3. L. Revesz, E. Blum, and R. Wicki, Tetrahedron Lett., 2005, 46, 5577. CrossRef
4. A. L. Katritzky, V. J. Baker, I. J. Ferguson, and R. C. Patel, J. Chem. Soc., Perkin Trans. 2, 1979, 143. CrossRef
5. S. C. Shim, D.-W. Kim, S.-S. Moon, and Y. B. Chae, J. Org. Chem., 1983, 49, 1449. CrossRef
6. R. Paredes, R. Abonia, J. Cadavid, R. M. Fuquen, A. Jaramillo, A. Hormaza, A. Ramirez, and A. Kennedy, Tetrahedron, 2002, 58, 55. CrossRef
7. A. Albert and H. Yamamoto, J. Chem. Soc., C, 1968, 1944. CrossRef
8. L. Y. Ukhin and L. G. Kuz’mina, Russ. Chem. Bull., 2004, 53, 2262. CrossRef
9. P. Molina, A. Arques, I. Cartagena, and R. Obón, Tetrahedron Lett., 1991, 32, 2521; CrossRef P. Molina, A. Arques, A. Tárraga, and M. d. R. Obón, Tetrahedron, 1998, 54, 997. CrossRef
10. A. Leganza, C. Bezze, C. Zonta, F. Fabris, O. De Lucchi, and A. Linden, Eur. J. Org. Chem., 2006, 2987. CrossRef
11. K. E. Frank and J. Aubé, Tetrahedron Lett., 1998, 39, 7239; CrossRef K. E. Frank and J. Aubé, J. Org. Chem., 2000, 65, 655. CrossRef
12. B. Pettersson, J. Bergman, and P. H. Svensson, Tetrahedron, 2013, 69, 2647. CrossRef
13. K. Tanaka, E. R. O. Siwu, S. Hirosaki, T. Iwata, R. Matsumoto, Y. Kitagawa, A. R. Pradipta, M. Okumura, and K. Fukase, Tetrahedron Lett., 2012, 53, 5899. CrossRef
14. J. M. Quintela, R. Alvarez-Sarandés, C. Peinador, and M. Maestro, J. Chem. Soc., Perkin Trans. 1, 1998, 3557. CrossRef
15. C. Redshaw, P. T. Wood, and M. R. J. Elsegood, Organometallics, 2007, 26, 6501. CrossRef
16. R. Todd, G. Rubio, D. J. Hall, S. Tempelaar, and A. P. Dove, Chem. Sci., 2013, 4, 1092. CrossRef