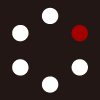
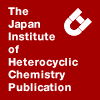
HETEROCYCLES
An International Journal for Reviews and Communications in Heterocyclic ChemistryWeb Edition ISSN: 1881-0942
Published online by The Japan Institute of Heterocyclic Chemistry
e-Journal
Full Text HTML
Received, 29th August, 2013, Accepted, 24th September, 2013, Published online, 27th September, 2013.
DOI: 10.3987/COM-13-S(S)114
■ Palladium-Catalyzed Cross-Coupling Reaction of Trialkylbismuthines with 2-Haloazines and Diazines
Pauline Petiot and Alexandre Gagnon*
Département de chimie, UQAM - Université du Québec à Montréal, C.P. 8888, Succ. Centre-Ville, Montréal, Québec, H3C 3P8, Canada
Abstract
An efficient method for the cross-coupling reaction of primary trialkybismuth reagents with 2-haloazines and diazines is reported. The reaction functions with pyridines, pyrimidines, pyridazines and pyrazines and tolerates many functional groups. This method gives access to 2-alkylazines and diazines, a class of compounds which is important in medicinal chemistry.Azines and diazines are ubiquitous in pharmaceutical industry as they can show a wide diversity of biological activities.1 The introduction of an alkyl group alpha to the nitrogen of an azine or a diazine allows to screen the binding environment surrounding a bioactive compound2 and to modulate its biophysical properties such as its lipophilicity, basicity, and permeability.3 Therefore, the development of efficient and general methods that allow the preparation of 2-alkylazines and diazines is highly important for organic and medicinal chemistry. The cross-coupling of sp3 alkylmetal species4 with azines and diazines bearing an halogen at the 2-position is an attractive approach to access the corresponding 2-alkyl heterocyclic derivatives. However, this type of coupling is notoriously difficult and only isolated examples involving organoboronic acids,5 organozincs,6 organomagnesium7 and organotin8 reagents have been reported. In addition, some of these methods suffer from scope limitation and poor functional group tolerance, or necessitate strict anhydrous conditions or complex and costly catalysts to prevent the undesired β-hydride elimination pathway. To the best of our knowledge, there is currently no comprehensive report on the cross-coupling reaction of alkylmetals with 2-halo pyridines, pyrimidines, pyridazines and pyrazines. Consequently, there is a well justified need to develop a general procedure to accomplish this transformation.
Our group has reported over the past years a portfolio of reactions for the formation of C–C,9 C–O,10 and C–N11 bonds involving organobismuth reagents. Organobismuthanes have found increasing use in bond formation due to their unique properties and reactivity.12 These reagents, which can be easily prepared from inexpensive and non toxic bismuth salts, are very tolerant to a wide diversity of functional groups and thus represent highly attractive species for methodology development.
We recently reported the cross-coupling of trialkylbismuth reagents with aryl and heteroaryl halides and pseudo halides13 and demonstrated that triphenethylbismuthine reacts smoothly with 2-chloro-3-cyanopyridine 1 to furnish the corresponding coupling product 2 in good yield (Scheme 1). Interestingly, and contrary to cross-coupling reactions with other alkymetal species, no product coming from the β-hydride elimination pathway could be detected under these conditions. We would like to report herein the full scope of the cross-coupling reaction of trialkylbismuthines with 2-halo- and 2-triflyl- azines and diazines 3, a process which leads to medicinally relevant 2-alkylazines and diazines 4 (Scheme 1).
We began by optimizing the reaction conditions for the cross-coupling reaction between tri-n-butylbismuthine and 2-chloro-6-methylpyridine 5 (Table 1). Tri-n-butylbismuthine was prepared in situ by the addition of n-butylmagnesium bromide over bismuth chloride in THF and was not isolated (see experimental section). The reaction mixture was diluted with DMF to quench any excess Grignard reagent remaining and the trialkylbismuth reagent thus formed was used directly in the cross-coupling reaction. This one-pot protocol avoids any cumbersome isolation of the air sensitive trialkylbismuthine. Using conditions that we previously reported,13 we obtained the desired product 6 in 32% yield (entry 1). Changing the catalyst for systems that are more suited for cross-coupling reactions with aryl chorides proved inefficient in our hands (entry 2 and 3). Replacing the base by potassium phosphate proved to be inconsequential (entry 4) and to our surprise, the reaction proceeded even in the absence of base (entry 5). While these results suggest that the choice of the base is irrelevant, we found that the yield could be substantially improved upon using cesium carbonate as the base instead of potassium carbonate (entry 6). The yield was further ameliorated by using 1.5 or 2.0 equivalents of tributylbismuth (entry 7 and 8). Performing the reaction with 1.1 or 1.5 equivalent of tributylbismuth at 130 ˚C in the presence of cesium carbonate provided the best yields of the coupling product (entries 9 and 10).
Using our optimized conditions, we next investigated the scope of the reaction by coupling different trialkylbismuthines with 2-halo- and 2-triflylazines and diazines bearing a wide range of functional groups (Table 2). In order to obtain optimal yields, we elected to perform the reaction using 1.5 equivalents of the organobismuth reagent. Firstly, the results demonstrate that the reaction functions efficiently with pyrazines (entry 1-3, 10), pyrimidines (entry 4 and 5), pyridazines (entry 6) and pyridines (entry 7-9). Secondly, the reaction furnishes the coupling product in similar yields upon using the chloro or bromo electrophile, but leads to modest yields when a 2-triflyl substrate is used (entry 8). The study indicates that functional groups such as ketones (entry 7), nitriles (entry 9), and amines (entry 10) are well tolerated. These groups can be used for further functionalization of the product, a feature which is important for applications in medicinal chemistry. Enolizable groups that would normally not be tolerated with Grignard reagents, such as a methyl ketone (entry 7), are unaffected in the course of the reaction. Attempt at performing the reaction directly with Grignard species under iron catalysis led to the product of addition on the ketone in low yield. While the reaction functions with most primary linear alkyl groups, we found that cyclopropyl was the only secondary alkyl group capable of undergoing cross-coupling under these conditions (entry 2). Aryl and heteroaryl cyclopropanes are very useful in medicinal chemistry since the cyclopropyl group usually shows higher metabolic stability than acyclic alkyl groups in the presence of cytochromes P450.14
In summary, we have developed a general procedure for the cross-coupling of trialkylbismuth reagents with 2-halo- and 2-triflylazines and diazines. The method operates under simple conditions using a simple catalyst. The procedure tolerates many functional groups and the compounds that are generated are highly valuable in medicinal chemistry. Studies aimed at transferring other substituted and functionalized alkyl groups are in progress in our laboratories and results will be reported in due course.
EXPERIMENTAL
All reactions were run under argon atmosphere in non flame dried glassware. Unless otherwise stated, commercial reagents were used without further purification. Grignard reagents were purchased from Aldrich or prepared using conventional methods with metallic magnesium and were titrated prior use.15 Anhydrous bismuth chloride 99.999% was purchased from Strem Chemicals. Anhydrous solvents were obtained using a MBRAUN (model MB-SPS 800) encapsulated solvent purification system. The evolution of reactions was monitored by analytical thin-layer chromatography using silica gel 60 F254 precoated plates. Flash chromatography was performed employing 230-400 mesh silica (Silicycle) using the indicated solvent system according to standard techniques.16 Nuclear magnetic resonance spectra (1H, 13C) were recorded on a Bruker Avance-III 300MHz spectrometer. Chemical shifts for 1H-NMR spectra are recorded in parts per million from tetramethylsilane with the solvent resonance as the internal standard (chloroform, δ 7.27 ppm, DMSO δ 2.54 ppm). Data are reported as follows: chemical shift, multiplicity (s = singlet, d = doublet, t = triplet, q = quartet, m = multiplet), coupling constant J in Hz and integration. Chemical shifts for 13C spectra are recorded in parts per million from tetramethylsilane using the central peak of deuterochloroform (77.00 ppm) as the internal standard. All 13C spectra were obtained with complete proton decoupling. IR spectra were recorded on a Thermo Scientific Nicolet 6700 PT-IR from thin films and are reported in reciprocal centimeters (cm-1).
General procedure for the cross-coupling reaction of trialkylbismuthines with 2-halo and 2-triflylazines and diazines
In a round-bottom flask equiped with a condenser and sparged with argon, BiCl3 (144 mg, 0.46 mmol) was suspended in anhydrous THF (2.3 mL) and cooled to 0 °C. The organomagnesium reagent (1.37 mmol, THF solution) was slowly added dropwise, and the solution was stirred at 0 °C for 5 min, warmed to rt, and then heated at 65 °C for 30 min to assure complete addition of all three alkyl groups on the bismuth center. The reaction mixture was cooled to rt and diluted with anhydrous DMF (1 mL). A DMF solution of the heteroaryl halide (0.31 mmol in 3 mL) was then added, followed by Cs2CO3 (0.6 mmol) and Pd(PPh3)4 (18 mg, 0.015 mmol). The solution was degassed by bubbling argon during 15 min and then stirred at 130 °C for 18 h. The reaction mixture was cooled to rt, diluted with sat. aq. NaHCO3 (10 mL) and extracted with EtOAc (2 x 10 mL). The combined organic phases were washed with brine (3 x 10 mL), dried over Na2SO4, filtered, and concentrated under reduced pressure. The residue was purified by flash chromatography using the indicated solvent system to afford the desired product. When necessary, the compound was further purified by preparative thin layer chromatography using the same solvent system. The alcohol derived from the oxidation of the trialkylbismuth is sometimes observed as a side product.
2-n-Butyl-6-methylpyridine (6)
The general procedure was followed on a 0.39 mmol scale starting from 2-chloro-6-methylpyridine 5. The crude material was purified on silica gel (20% EtOAc/hexanes) to afford 6 as a colorless oil (38 mg, 66%). Spectral data was identical to literature compound.17 1H-NMR (300 MHz, CDCl3) δ 7.44 (t, J = 6.2 Hz, 1H), 6.92 (d, J = 8.8 Hz, 2H), 2.73 (t, J = 9.1 Hz, 2H), 2.50 (s, 3H), 1.69-1.63 (m, 2H), 1.40-1.33 (m, 2H), 0.91 (t, J = 9.2 Hz, 3H).
2-Methylquinoxaline (4a)
The general procedure was followed on a 0.31 mmol scale starting from 2-chloroquinoxaline 3a. The crude material was purified on silica gel (40% EtOAc/hexanes) to afford 4a as a colorless oil (38 mg, 86%). Spectral data was identical to literature compound.18 1H-NMR (300 MHz, CDCl3) δ 8.72 (s, 1H), 8.07-7.98 (m, 2H), 7.75-7.65 (m, 2H), 2.76 (s, 3H).
2-Cyclopropylquinoxaline (4b)
The general procedure was followed on a 0.31 mmol scale starting from 2-chloroquinoxaline 3a. The crude material was purified on silica gel (15% EtOAc/hexanes) to afford 4b as a colorless oil (36 mg, 70%). Spectral data was identical to literature compound.19 1H-NMR (300 MHz, CDCl3) δ 8.71 (s, 1H), 8.03 (dd, J = 8.3, 1.2 Hz, 1H), 7.92 (dd, J = 8.3, 1.2 Hz, 1H), 7.70-7.58 (m, 2H), 2.29-2.21 (m, 1H), 1.26-1.23 (m, 2H), 1.18-1.13 (m, 2H).
2-Phenethylquinoxaline (4c)
The general procedure was followed on a 0.31 mmol scale starting from 2-chloroquinoxaline 3a. The crude material was purified on silica gel (15% EtOAc/hexanes) to afford 4c as a colorless oil (60 mg, 85%). Spectral data was identical to literature compound.20 1H-NMR (300 MHz, CDCl3) δ 8.69 (s, 1H), 8.13 (dd, J = 8.0, 2.0 Hz, 2H), 7.83-7.73 (m, 2H), 7.35-7.26 (m, 5H), 3.42-3.37 (m, 2H), 3.27-3.22 (m, 2H).
2-Phenethylpyrimidine (4d)
The general procedure was followed on a 0.44 mmol scale starting from 2-chloropyrimidine 3d. The crude material was purified on silica gel (30% EtOAc/hexanes) to afford 4d as a colorless oil (54 mg, 68%). Spectral data was identical to literature compound.20 1H-NMR (300 MHz, CDCl3) δ 8.73 (d, J = 6.3 Hz, 2H), 7.35-7.22 (m, 5H), 7.13 (t, J = 8.9 Hz, 1H), 3.36-3.29 (m, 2H), 3.24-3.18 (m, 2H).
5-Ethyl-2-n-propylpyrimidine (4e)
The general procedure was followed on a 0.35 mmol scale starting from 2-chloro-5-ethylpyrimidine 3e. The crude material was purified on silica gel (20% EtOAc/hexanes) to afford 4e as a colorless oil (28 mg, 53%): Rf 0.30 (25% EtOAc/hexanes); 1H NMR (300 MHz, CDCl3) δ 8.46 (s, 2H), 2.88 (t, J = 8.1 Hz, 2H), 2.60 (q, J = 8.2 Hz, 2H), 1.85-1.77 (m, 2H), 1.24 (t, J = 7.9 Hz, 3H), 0.96 (t, J = 8.2 Hz, 3H); 13C-NMR (75 MHz, CDCl3) δ 168.9, 156.4, 133.2, 41.0, 23.3, 22.1, 15.0, 13.9; IR (neat) 3431, 2960, 2926, 1713, 1633, 1466.
3-n-Butyl-6-phenylpyridazine (4f)
The general procedure was followed on a 0.26 mmol scale starting from 3-chloro-6-phenylpyridazine 3f. The crude material was purified on silica gel (15% EtOAc/hexanes) to afford 4f as a colorless oil (24 mg, 46%). Spectral data was identical to literature compound.21 1H-NMR (300 MHz, CDCl3) δ 8.05 (dd, J = 8.2, 1.9 Hz, 2H), 7.75 (d, J = 8.8 Hz, 1H), 7.49-7.46 (m, 3H), 7.35 (d, J = 8.8 Hz, 1H), 3.01 (t, J = 7.7 Hz, 2H), 1.82-1.74 (m, 2H), 1.46-1.39 (m, 2H), 0.96 (t, J = 7.3 Hz, 3H).
2-Acetyl-6-phenethylpyridine (4g)
The general procedure was followed on a 0.25 mmol scale starting from 2-acetyl-6-bromopyridine 3g. The crude material was purified on silica gel (15% EtOAc/hexanes) to afford 4g as a colorless oil (27 mg, 48%): Rf 0.56 (20% EtOAc/hexanes); 1H-NMR (300 MHz, CDCl3) δ 7.91 (dd, J = 7.9, 0.7 Hz, 1H), 7.73 (t, J = 7.7 Hz, 1H), 7.35-7.23 (m, 6H), 3.24-3.15 (m, 4H), 2.78 (s, 3H); 13C-NMR (75 MHz, CDCl3) δ 200.8, 160.7, 153.3, 141.4, 136.9, 128.5, 128.4, 126.4, 126.1, 119.0, 39.6, 35.4, 25.8; IR (neat) 3027, 2920, 1697, 1586, 1453, 1355.
2-Ethyl-5-methylpyridine (4h)
The general procedure was followed on a 0.21 mmol scale starting from 2-triflyl-5-methylpyridine 3h. The crude material was purified on silica gel (20% EtOAc/hexanes) to afford 4h as a colorless oil (6 mg, 24%). Spectral data was identical to literature compound.22 1H-NMR (300 MHz, CDCl3) δ 8.34 (d, J = 1.5 Hz, 1H), 7.41 (dd, J = 7.8, 1.9 Hz, 1H), 7.06 (d, J = 7.9 Hz, 1H), 2.79 (q, J = 7.3 Hz, 2H), 2.29 (s, 3H), 1.28 (t, J = 7.6 Hz, 3H).
6-Ethyl-3-cyanopyridine (4i)
The general procedure was followed on a 0.36 mmol scale starting from 6-chloro-3-pyridinecarbonitrile 3i. The crude material was purified on silica gel (20% EtOAc/hexanes) to afford 4i as a colorless oil (17 mg, 40%). Spectral data was identical to literature compound. 1H-NMR (300 MHz, CDCl3) δ 8.73 (d, J = 1.6 Hz, 1H), 7.80 (dd, J = 8.1, 2.2 Hz, 1H), 7.21 (d, J = 8.2 Hz, 1H), 2.83 (q, J = 7.6 Hz, 2H), 1.26 (t, J = 7.6 Hz, 3H); 13C-NMR (75 MHz, CDCl3) 168.1, 152.1, 139.4, 122.2, 117.0, 107.2, 31.8, 13.3; IR (neat) 3432, 2972, 2933, 2232, 1594, 1484.
2-Ethyl-6-dimethylaminepyrazine (4j)
The general procedure was followed on a 0.32 mmol scale starting from 2-chloro-6-dimethylamine pyridine 3j. The crude material was purified on silica gel (35% EtOAc/hexanes) to afford 4j as a colorless oil (34 mg, 71%): Rf 0.36 (20% EtOAc/hexanes); 1H-NMR (300 MHz, CDCl3) δ 7.80 (s, 1H), 7.65 (s, 1H), 3.08 (s, 6H), 2.62 (q, J = 7.6 Hz, 2H), 1.25 (t, J = 7.6 Hz, 3H); 13C-NMR (75 MHz, CDCl3) δ 155.6, 154.5, 130.0, 126.7, 37.4, 28.5, 13.3; IR (neat) 2967, 2933, 1575, 1529, 1423, 1194.
ACKNOWLEDGEMENTS
This work was supported by Natural Sciences and Engineering Research Council of Canada (NSERC) and by the Université du Québec à Montréal. P.P. is grateful to Université du Québec à Montréal for a FARE scholarship.
References
1. C. Shilpa, S. Dipak, S. Vimukta, and D. Arti, J. Pharm. Biomed. Sci., 2012, 21, 1; T. P. Selvam, C. R. James, P. V. Dniandev, and S. K. Valzita, Res. Pharm., 2012, 2, 1; A. Verma, L. Sahu, N. Chaudhary, T. Dutta, D. Dewangan, and D. K. Tripathi, Asian J. Biochem. Pharm. Res., 2012, 2, 1; S. B. Ferreira and C. R. Kaiser, Exp. Opin. Ther. Pat., 2012, 22, 1033; CrossRef C. G. Wermuth, Med. Chem. Commun., 2011, 2, 935; CrossRef R. Mishra and I. Tomar, Int. J. Pharm. Sci. Res., 2011, 2, 758.
2. C. Bissantz, B. Kuhn, and M. Stahl, J. Med. Chem., 2010, 53, 5061. CrossRef
3. I. Yusof and M. D. Segall, Drug Disc. Today, 2013, 18, 659; CrossRef B. Testa, A. Pedretti, and G. Vistolli, Drug Disc. Today, 2012, 17, 549. CrossRef
4. For reviews on cross-coupling reactions involving alkylmetal species, see: R. Jana, T. P. Pathak, and M. S. Sigman, Chem. Rev., 2011, 111, 1417; CrossRef T.-Y. Luh, M.-K. Leung, and K.-T. Wong, Chem. Rev., 2000, 100, 3187; CrossRef A. C. Frisch and M. Beller, Angew. Chem. Int. Ed., 2005, 44, 674; CrossRef J. Terao and N. Kambe, Acc. Chem. Res., 2008, 41, 1545. CrossRef
5. I. Kondolff, H. Doucet, and M. Santelli, Tetrahedron, 2004, 60, 3813; CrossRef G. A. Molander and C.-S. Yun, Tetrahedron, 2002, 58, 1465; CrossRef A. Ohta, M. Ohta, Y. Igarashi, K. Saeki, K. Yuasa, and T. Mori, Heterocycles, 1987, 26, 2449. CrossRef
6. M. Haberberger, C. I. Someya, A. Company, E. Irran, and S. Enthaler, Catal. Lett., 2012, 142, 557; CrossRef T. Sakamoto, S. Nishimura, Y. Kondo, and H. Yamanaka, Synthesis, 1988, 485. CrossRef
7. J. Kleimark, P.-F. Larsson, P. Emamy, A. Hedström, and P.-O. Norrby, Adv. Synth. Catal., 2012, 354, 448; CrossRef H. Ohmiya, H. Yorimitsu, and K. Oshima, Chem. Lett., 2004, 33, 1240; CrossRef A. Fürstner and A. Leitner, Angew. Chem. Int. Ed., 2002, 41, 609; CrossRef A. Fürstner, A. Leitner, M. Mendez, and H. Krause, J. Am. Chem. Soc., 2002, 124, 13856; CrossRef O. Piccolo and T. Martinengo, Syn. Commun., 1981, 11, 497; CrossRef B. Scheiper, M. Bonnekessel, H. Krause, and A. Fürstner, J. Org. Chem., 2004, 69, 3943; CrossRef B. D. Sherry and A. Fürstner, Acc. Chem. Res., 2008, 41, 1500; CrossRef H. Hamaguchi, M. Uemura, H. Yasui, H. Yorimitsu, and K. Oshima, Chem. Lett., 2008, 37, 1178. CrossRef
8. T. Watanabe, K. Hayashi, J. Sakurada, M. Ohki, N. Takamatsu, H. Hirohata, K. Takeuchi, K. Yuasa, and A. Ohta, Heterocycles, 1989, 29, 123. CrossRef
9. P. Petiot and A. Gagnon, Eur. J. Org. Chem., 2013, 5282; CrossRef A. Gagnon, M. Duplessis, P. Alsabeh, and F. Barabé, J. Org. Chem., 2008, 73, 3604. CrossRef
10. C. Crifar and A. Gagnon, unpublished work.
11. A. Gagnon, M. St-Onge, K. Little, M. Duplessis, and F. Barabé, J. Am. Chem. Soc., 2007, 129, 44. CrossRef
12. For reviews on organobismuthanes, see: S. Shimada and M. L. N. Rao, Top. Curr. Chem., 2012, 311, 199; CrossRef H. Suzuki, T. Ikegami, and Y. Matano, Synthesis, 1997, 249; CrossRef J.-P. Finet, Chem. Rev., 1989, 89, 1487; CrossRef D. H. R. Barton and J.-P. Finet, Pure Appl. Chem., 1987, 59, 937; CrossRef L. D. Freedman and G. O. Doak, Chem. Rev., 1982, 82, 15; CrossRef H. Gilman and H. L. Yale, Chem. Rev., 1942, 30, 281. CrossRef
13. A. Gagnon, V. Albert, and M. Duplessis, Synlett, 2010, 2936. CrossRef
14. A. Gagnon, M. Duplessis, and L. Fader, Org. Prep. Proc. Int., 2010, 42, 1. CrossRef
15. Y. Aso, H. Yamashita, T. Otsubo, and F. Ogura, J. Org. Chem., 1989, 54, 5627. CrossRef
16. C. W. Still, M. Kahn, and A. Mitra, J. Org. Chem., 1978, 43, 2923. CrossRef
17. T. Rizk, E. J.-F. Bilodeau, and A. M. Beauchemin, Angew. Chem. Int. Ed., 2009, 48, 8325. CrossRef
18. Z. Li, J. Zhang, Y. Li, and H. Gao, J. Mol. Structure, 2013, 1035, 69. CrossRef
19. G. A. Molander and P. E. Gormisky, J. Org. Chem., 2008, 73, 7481. CrossRef
20. Y. Zhang, M. R. Sheets, E. K. Raja, K. N. Boblak, and D. A. Klumpp, J. Am. Chem. Soc., 2011, 133, 8467. CrossRef
21. F. Buron, N. Plé, A. Turck, and F. Marsais, Synlett, 2006, 1586. CrossRef
22. B. Adler, C. Burtzlaff, C. Duschek, J. Ohl, and W. Zech, J. Fuer Praktische Chem., 1978, 320, 904. CrossRef