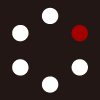
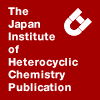
HETEROCYCLES
An International Journal for Reviews and Communications in Heterocyclic ChemistryWeb Edition ISSN: 1881-0942
Published online by The Japan Institute of Heterocyclic Chemistry
e-Journal
Full Text HTML
Received, 24th August, 2013, Accepted, 27th September, 2013, Published online, 16th October, 2013.
DOI: 10.3987/COM-13-12818
■ Efficient Organocatalytic Michael Addition Reaction of β−Ketoesters under High Pressure
Maya Moritaka, Keiji Nakano, Yoshiyasu Ichikawa, and Hiyoshizo Kotsuki*
Laboratory of Natural Products Chemistry, Faculty of Science, Kochi University, 2-5-1, Akebono-cho, Kochi 780-8520, Japan
Abstract
The Michael addition reaction of β−ketoesters was efficiently promoted by a cooperative dual catalyst system composed by DMAP and thiourea A under high-pressure conditions (0.8 GPa) in toluene. The expected relatively congested adducts of 1,5-dicarbonyl compounds were prepared in high to excellent yield.The Michael addition reaction is widely recognized as one of the most important carbon–carbon bond-forming reactions in organic synthesis.2 This reaction can generally be carried out using strong base metal reagents under mostly dry conditions. However, the base metal-catalyzed method sometimes suffers from disadvantages, such as incompatibility with a base-sensitive functionality and the occurrence of side reactions such as multi-condensation, the retro-Michael reaction, and polymerization. In contrast, recent advances in the development of organocatalytic methods offer Michael addition reactions using a fairly mild procedure.3 In fact, organocatalytic synthesis has several important advantages due to the ready availability, non-toxicity, ease of handling, insensitivity to moisture and oxygen, and environmentally friendly nature of the compounds involved. However, significant limitations still remain to be solved in this field, including high catalyst loading, a long reaction period, and harmful organic solvent media. In some cases, the application of high pressure (0.8-1.0 GPa) can strongly promote Michael addition reactions with the use of weakly reactive substrates.4
In our laboratory, we have been working to develop a new method for Michael addition reactions using 4-dimethylaminopyridine (DMAP) and related organocatalysts.5 As an extension of this work, we anticipated that the synergistic effect of DMAP as a mildly basic organocatalyst6 and thiourea catalysts as a strong hydrogen-bonding activator7 could enhance the nucleophilicity of β−ketoesters even for less reactive substrates under high-pressure conditions. We describe here the realization of this expectation.
To find the optimum conditions, the Michael addition reaction of ethyl acetoacetate (2a) with 1.2 equiv of 2-cyclohexen-1-one (1a) was carried out in the presence of a catalytic amount of DMAP together with various thioureas as co-catalysts (Table 1).
An increased pressure dramatically accelerated the reaction: at atmospheric pressure, only a trace amount of 3a was obtained even in the presence of 10 mol% of DMAP and 10 mol% of thiourea A, whereas at 0.8 GPa, the reaction proceeded smoothly to give 3a in nearly quantitative yield (Table 1, entries 1 and 6). As expected, the yields decreased at lower pressures (Table 1, entries 4 and 5).
Next, it became clear that there was a significant catalyst effect: the co-existence of both catalysts was essential, and the use of each catalyst individually had less or no effect (Table 1, entry 6 vs entries 2 and 3). Among the catalysts screened, thiourea A was the best in terms of efficiency (Table 1, entry 6 vs entries 10-12). We briefly examined the solvent effect in this Michael addition reaction: dichloromethane, THF, and EtOH led to insufficient conversion, suggesting that a polar coordinating solvent inhibits the formation of an effective hydrogen-bonding network between 2a and thiourea A (Table 1, entry 6 vs entries 7-9). Interestingly, in EtOH, the bicyclo[2.2.0]octanone compound 4 formed by an intramolecular aldol reaction was isolated in 17% yield (Table 1, entry 9).8
Although the reason for the high efficiency observed in this cooperative dual catalyst system is unclear, we believe that 2a would be activated by a thiourea moiety through double hydrogen-bonding, and enhance the acidity of 2a (pKa = 11 in H2O)9 to effectively cause proton-abstraction by DMAP (pKa = 9.7 for the conjugate acid in H2O)10 (Scheme 1).11 Interestingly, these donor-catalyst interactions as well as C–C bond-forming processes are both favorable under high-pressure conditions, and significantly accelerate the reaction rate to give the final product 3a.
To confirm the above speculation regarding the formation of a double hydrogen-bonding network between 2a and thiourea A, 13C NMR experiments (in C6D6) were performed (Figure 1). Downfield shifts of 0.382 ppm and 0.106 ppm for ketone and ester C=O, respectively, and an up-field shift of 0.095 ppm for CH2 were observed when complexed with thiourea A. On the other hand, 1H NMR experiments revealed that there was no significant shift in keto-enol tautomeric equilibria on the NMR time-scale: 16% of the enol content for 2a alone vs 18% in the presence of thiourea A.
With the optimized reaction conditions in hand, we then investigated the general scope of this chemistry by using various donor and acceptor substrates (Table 2). Several β−ketoesters such as 2b and 2c and related donors such as diethyl malonate (2d) and acetylacetone (2e) efficiently reacted with 1a to give the corresponding adducts in good to excellent yields (Table 2, entries 1-4). The procedure is highly sensitive to steric problems: while the reaction between 1a and 2f was retarded, the analogous combinations of 1a and 2g or 1b and 2g were quite successful and the expected highly congested adducts 3g and 3h were obtained in nearly quantitative yields (Table 2, entries 5-7). Finally, methyl acrylate (1c) was used as a moderately reactive Michael acceptor, and highly successful reactions with 2b and 2c were observed (Table 2, entries 8 and 9). As expected, 1c reacted with 2f to afford 3k in moderately good yield (Table 2, entry 10).
The results presented here suggest that the cooperative dual catalyst system composed by DMAP and thiourea A represents a new efficient method for constructing the complex molecules bearing sterically congested stereogenic centers. The reaction is performed under high-pressure conditions and, depending on the substrate, high to excellent yields can be attained. Overall, the present results indicate that hyperbaric Michael addition offers attractive solutions to problems in synthetic methodology that are otherwise often difficult to solve. Finally, it may be possible to extend this method to asymmetric transformations using chiral DMAP or chiral thiourea catalysts, and further studies along these lines are now in progress in our laboratory.12
EXPERIMENTAL
General remarks: 1H and 13C NMR spectra were recorded on a JEOL LA-400 spectrometer (400 MHz and 100 MHz, respectively) or JNM-ECA-500 spectrometer (500 MHz and 125.8 MHz, respectively) for solution in CDCl3. Chemical shifts (δ) in ppm are reported using residual chloroform (7.27 for 1H and 77.20 for 13C) as an internal reference. Coupling constants (J) are given in Hertz. IR spectra were measured with a JASCO FT/IR-460 plus Fourier Transform Infrared spectrophotometer. High-pressure reactions were performed in a Hikari-koatsu HR-15-B3 apparatus, which is designed for pressures up to 1.0 GPa. The silica gel used for flash chromatography was 230-400 Mesh. All reagents were of reagent grade and used as such or distilled prior to use. All solvents were dried according to standard procedures and freshly distilled prior to use. Thiourea catalysts A-D used in this work were prepared as described in the literature.13
Typical Procedure for the Michael Addition Reaction of β−Ketoesters with Enones
A mixture of 1a (1.2 mmol), 2a (1.0 mmol), DMAP (0.1 mmol), and thiourea A (0.1 mmol) in toluene (1.4 mL) was placed in a Teflon reaction vessel and allowed to react at 0.8 GPa and rt for 12 h. After the pressure was released, the mixture was concentrated in vacuo. The crude product was purified by column chromatography (silica gel, elution with hexane–EtOAc).
Ethyl 3-oxo-2-(3-oxocyclohexyl)butanoate (3a)14
A 1 : 1 mixture of the two diastereomers; pale yellow oil; Rf 0.54 (hexane–AcOEt = 2 : 1); FTIR (KBr): ν = 1737, 1713, 1448, 1423, 1362 cm-1; 1H NMR (400 MHz, CDCl3): δ = 1.28, 1.29 (each 3H, t, J = 6.8 Hz), 1.36-1.52 (2H, m), 1.63-1.76 (2H, m), 1.83-1.94 (2H, m), 2.03-2.18 (4H, m), 2.22, 2.25 (each 3H, s), 2.25-2.43 (6H, m), 2.53-2.64 (2H, m), 3.40 (1H, d, J = 7.8 Hz), 3.41 (1H, d, J = 8.8 Hz), 4.18-4.25 (4H, m); 13C NMR (100 MHz, CDCl3): δ = 13.9(1), 13.9(4), 24.3(6), 24.3(8), 28.4, 28.8, 29.4 (×2), 37.6 (×2), 40.8(5), 40.8(9), 44.8, 45.2, 61.4(2), 61.4(6), 64.5 (×2), 64.8(7), 64.8(9), 167.9, 168.1, 201.4, 201.5, 209.3, 209.4.
Bicyclo[2.2.2]octanone 48
Amorphous solid; Rf 0.25 (hexane–AcOEt = 2 : 1); mp 58-59 °C (lit.,8 80 °C); FTIR (KBr): ν = 3411, 1724, 1467, 1398, 1374 cm-1; 1H NMR (500 MHz, CDCl3): δ = 1.22 (3H, s), 1.31 (3H, t, J = 7.0 Hz), 1.50-1.57 (1H, m), 1.64-1.71 (2H, m), 1.76-1.83 (1H, m), 2.00-2.03 (1H, m), 2.11 (1H, dt, J = 19.5, 2.5 Hz), 2.21-2.36 (2H, m), 2.41 (1H, br), 2.81 (1H, s), 4.21 (2H, m); 13C NMR (125.8 MHz, CDCl3): δ = 14.3, 17.5, 25.6, 27.1, 30.1, 39.6, 54.4, 57.4, 60.6, 71.7, 172.8, 214.3.
Ethyl 2-methyl-3-oxo-2-(3-oxocyclohexyl)butanoate (3b)15
A 1 : 1 mixture of the two diastereomers; colorless oil; Rf 0.22 (hexane–AcOEt = 4 : 1); FTIR (KBr): ν = 1713, 1450, 1425, 1386, 1358, 1243, 1232 cm-1; 1H NMR (400 MHz, CDCl3): δ = 1.27, 1.28 (each 3H, t, J = 6.8 Hz), 1.33, 1.36 (each 3H, s), 1.32-1.51 (2H, m), 1.62-1.86 (4H, m), 2.06-2.13 (4H, m), 2.15, 2.16 (each 3H, s), 2.19-2.28 (4H, m), 2.39-2.45 (2H, m), 2.56-2.66 (2H, m), 4.16-4.26 (4H, m); 13C NMR (100 MHz, CDCl3): δ = 13.9(1), 13.9(4), 15.0, 15.2, 24.7, 24.8, 26.3, 26.4, 26.5, 26.9, 41.0(2), 41.0(5), 41.6, 41.8, 42.9, 43.4, 61.5, 61.6, 62.6, 62.7, 171.2, 171.4, 204.0, 204.1, 210.1 (×2).
Ethyl 3-oxo-2-(3-oxocyclohexyl)-3-phenylpropanoate (3c)16
A 1 : 1 mixture of the two diastereomers; colorless oil; Rf 0.34 (hexane–AcOEt = 4 : 1); FTIR (KBr) ν = 1734, 1713, 1687, 1597, 1580, 1448 cm-1; 1H NMR (400 MHz, CDCl3): δ = 1.16-1.20 (6H, m), 1.35-1.44 (2H, m), 1.51-1.61 (2H, m), 1.66-1.80 (2H, m), 1.90-2.31 (6H, m), 2.40-2.50 (4H, m), 2.79-2.88 (2H, m), 4.10-4.19 (4H, m), 4.27 (1H, d, J = 8.8 Hz), 4.29 (1H, d, J = 10.8 Hz), 7.42-8.03 (10H, m); 13C NMR (100 MHz, CDCl3): δ = 13.9(1), 13.9(4), 24.5, 24.6, 28.6, 29.3, 38.1, 38.2, 41.0 (×2), 45.1, 45.6, 58.9, 59.4, 61.5, 61.6, 128.4 (×2), 128.5 (×2), 128.7(8) (×2), 128.8(3) (×2), 133.7, 133.8, 136.4(1), 136.4(3), 168.0, 168.2, 193.3, 193.5, 209.5(6), 209.6(3).
Diethyl 2-(3-oxocyclohexyl)malonate (3d)17
Colorless oil; Rf 0.28 (hexane–AcOEt = 4 : 1); FTIR (KBr): ν = 1749, 1731, 1369 cm-1; 1H NMR (400 MHz, CDCl3): δ = 1.27(7), 1.28(1) (each 3H, t, J = 6.8 Hz), 1.51 (1H, dq, J = 12.7, 3.9 Hz), 1.69 (1H, tq, J = 12.7, 3.9 Hz), 1.92-2.00 (1H, m), 2.04-2.12 (1H, m), 2.22-2.31 (2H, m), 2.38-2.59 (3H, m), 3.30 (1H, d, J = 7.8 Hz), 4.21, 4.22 (each 2H, q, J = 6.8 Hz); 13C NMR (100 MHz, CDCl3): δ = 13.9(1), 13.9(3), 24.4, 28.6, 37.9, 40.9, 44.9, 56.7, 61.4 (×2), 167.6, 167.7, 209.5.
3-(3-Oxocyclohexyl)pentane-2,4-dione (3e)16
White solid; Rf 0.23 (hexane–AcOEt = 2 : 1); mp 42-44 °C; FTIR (KBr) ν = 1697, 1421, 1361 cm-1; 1H NMR (400 MHz, CDCl3): δ = 1.33-1.43 (1H, m), 1.64-1.84 (2H, m), 2.02-2.09 (2H, m), 2.17, 2.20 (each 3H, s), 2.23-2.45 (3H, m), 2.64-2.74 (1H, m), 3.64 (1H, d, J = 9.8 Hz); 13C NMR (100 MHz, CDCl3): δ = 24.4, 28.7, 29.6, 29.7, 38.3, 41.0, 45.1, 74.7, 202.7, 202.8, 209.1.
Methyl 2-oxo-1-(3-oxocyclohexyl)cyclohexanecarboxylate (3f)
A 1 : 1 mixture of the two diastereomers; colorless oil; Rf 0.30 (hexane–AcOEt = 4 : 1); FTIR (KBr): ν = 1711, 1450, 1235, 1207, 1193 cm-1; 1H NMR (500 MHz, CDCl3): δ = 1.26-1.33 (3H, m), 1.48-2.57 (17H, m), 4.18-4.31 (2H, m); 13C NMR (125.8 MHz, CDCl3): δ = 14.0(6), 14.1(5), 22.4, 22.7, 24.9 (×2), 26.5, 26.9, 27.2, 27.3, 31.8, 33.8, 41.1(8), 41.2(4), 41.4(1), 41.4(5). 41.5(2), 41.6, 42.9, 43.3, 61.3, 61.5, 63.2, 63.4, 170.5, 170.9, 206.3, 206.8, 210.6, 210.8. HRMS calcd. for C15H22O4: 266.1581, found 266.1513.
Methyl 2-oxo-1-(3-oxocyclohexyl)cyclopentanecarboxylate (3g)5d
A 1 : 1 mixture of the two diastereomers; white solid; Rf 0.40 (hexane–AcOEt = 2 : 1); mp 62-64 °C; FTIR (KBr): ν = 1747, 1716, 1455, 1434, 1233, 1223 cm-1; 1H NMR (400 MHz, CDCl3): δ = 1.25-1.69 (5H, m), 1.90-2.70 (25H, m), 3.73, 3.74 (each 3H, s); 13C NMR (100 MHz, CDCl3): δ = 19.4(5), 19.4(9), 24.5(6), 24.5(9), 26.3, 26.7, 28.5, 29.7, 38.6, 38.9, 40.9(5), 40.9(8), 41.8, 41.9, 43.0, 43.6, 52.7, 52.8, 63.7, 64.5, 169.7, 170.3, 209.6, 209.7, 213.3 (×2).
Methyl 2-oxo-1-(3-oxocyclopentyl)cyclopentanecarboxylate (3h)18
A 3 : 1.3 mixture of the two diastereomers; white solid; Rf 0.42 (hexane–AcOEt = 2 : 1); mp 61-63 °C (lit.18 60-61 °C); FTIR (KBr) ν = 1748, 1731, 1720, 1256, 1240 cm-1; 1H NMR (400 MHz, CDCl3): δ = 1.57-1.81 (1.4H, m), 1.91-2.07 (6.4H, m), 2.12-2.37 (5.6H, m), 2.43-2.54 (3.8H, m), 2.84-2.93 (1.4H, m), 3.73 (3H, s), 3.74 (1.3H, s); 13C NMR (100 MHz, CDCl3): δ = 19.5(1), 19.5(4), 24.2, 25.1, 30.2, 30.5, 38.1(6), 38.2(4), 38.4, 38.6, 39.9, 40.1, 40.2, 40.8, 52.6 (×2), 61.7, 62.0, 170.8, 170.9, 213.8, 214.0, 217.0, 217.2.
Methyl 4-ethoxycarbonyl-4-methyl-5-oxohexanonate (3i)5d
Colorless oil; Rf 0.45 (hexane–AcOEt = 2 : 1); FTIR (KBr): ν = 1739, 1713, 1438, 1360 cm-1; 1H NMR (400 MHz, CDCl3): δ = 1.27 (3H, t, J = 6.8 Hz), 1.35 (3H, s), 2.06-2.13 (1H, m), 2.17 (3H, s), 2.19-2.35 (3H, m), 3.67 (3H, s), 4.20 (2H, q, J = 6.8 Hz); 13C NMR (125.8 MHz, CDCl3): δ = 14.0, 18.9, 26.1, 29.2, 29.6, 51.7, 58.7, 61.5, 172.3, 173.2, 204.9.
Methyl 4-ethoxycarbonyl-4-benzoylbutanoate (3j)5d
Colorless oil; Rf 0.45 (hexane–AcOEt = 4 : 1); FTIR (KBr) ν = 1738, 1685, 1597, 1580, 1448, 1370 cm-1; 1H NMR (400 MHz, CDCl3): δ = 1 .17 (3H, t, J = 7.1 Hz), 2.26-2.35 (2H, m), 2.40-2.53 (2H, m), 3.68 (3H, s), 4.10-4.21 (2H, m), 4.50 (1H, t, J = 7.1 Hz ), 7.44-7.54 (2H, m), 7.60 (1H, t, J = 7.3Hz), 7.99-8.07 (2H, m); 13C NMR (125.8 MHz, CDCl3): δ = 13.9, 23.9, 31.1, 51.6, 52.6, 61.5, 128.6 (×2), 128.7, 133.6 (×2), 135.7, 169.5, 173.1, 194.9.
Methyl 2-oxo-1-(2’-methoxycarbonylethyl)cyclohexanecarboxylate (3k)19
Colorless oil; Rf 0.49 (hexane–AcOEt = 2 : 1); FTIR (KBr): ν = 1739, 1713, 1439 cm-1; 1H NMR (400 MHz, CDCl3): δ = 1.28 (3H, t, J = 6.8 Hz), 1.46 (1H, dt, J = 12.7, 3.9 Hz), 1.59-1.70 (2H, m), 1.73-1.79 (1H, m), 1.88-2.05 (2H, m), 2.15-2.29 (2H, m), 2.37-2.54 (4H, m), 3.67 (3H, s), 4.17-4.27 (2H, m); 13C NMR (125.8 MHz, CDCl3): δ = 14.1, 22.5, 27.5, 29.3, 29.6, 36.3, 41.0, 51.6, 59.9, 61.4, 171.6, 173.4, 207.6.
SUPPORTING INFORMATION
1H and 13C NMR spectra for all adducts.
ACKNOWLEDGEMENTS
This work was supported in part by the Yamada Science Foundation, and by a Grant-in-Aid for Scientific Research from the Ministry of Education, Culture, Sports, Science and Technology (MEXT) in Japan. We are also grateful to Prof. Y. Fukuyama of Tokushima Bunri University for MS/HRMS measurements.
References
1. High-Pressure Organic Chemistry. Part 38. For Part 37, see: K. Mori, T. Yamauchi, J. Maddaluno, K. Nakano, Y. Ichikawa, and H. Kotsuki, Synlett, 2011, 2080. CrossRef
2. Conjugate Addition Reactions in Organic Synthesis; P. Perlmutter, Pergamon Press: Oxford, 1992.
3. For reviews, see: a) D. Almasi, D. A. Alonso, and C. Nájera, Tetrahedron: Asymmetry, 2007, 18, 299; CrossRef b) S. B. Tsogoeva, Eur. J. Org. Chem., 2007, 1701; CrossRef c) J. L. Vicario, D. Badía, and L. Carrillo, Synthesis, 2007, 2065; CrossRef d) S. Sulzer-Mossé and A. Alexakis, Chem. Commun., 2007, 3123; CrossRef e) S. Jautze and R. Peters, Synthesis, 2010, 365; CrossRef f) Organocatalytic Enantioselective Conjugate Addition Reactions; J. L. Vicario, D. Badía, L. Carrillo, and E. Reyes, RSC Publishing: Cambridge, 2010.
4. a) J. D’Angelo and J. Maddaluno, J. Am. Chem. Soc., 1986, 108, 8112; CrossRef b) G. Jenner, New. J. Chem., 1995, 19, 173; c) A. Yu. Rulev, J. Maddaluno, G. Plé, J.-C. Plaquevent, and L. Duhamel, J. Chem. Soc., Perkin Trans. 1, 1998, 1397; CrossRef d) A. Yu. Rulev and J. Maddaluno, Eur. J. Org. Chem., 2001, 2569; CrossRef e) A. Yu. Rulev and J. Maddaluno, J. Phys. Org. Chem., 2002, 15, 590; CrossRef f) A. Yu. Rulev, N. Yenil, A. Pesquet, H. Oulyadi, and J. Maddaluno, Tetrahedron, 2006, 62, 5411; CrossRef g) Md. I. Uddin, K. Nakano, Y. Ichikawa, and H. Kotsuki, Synlett, 2008, 1402; CrossRef h) S. Azad, T. Kobayashi, K. Nakano, Y. Ichikawa, and H. Kotsuki, Tetrahedron Lett., 2009, 50, 48; CrossRef i) A. Yu. Rulev, S. Azad, H. Kotsuki, and J. Maddaluno, Eur. J. Org. Chem., 2010, 6331; CrossRef j) A. Yu. Rulev, H. Kotsuki, and J. Maddaluno, Green Chem., 2012, 14, 503. CrossRef
5. a) H. Kotsuki, H. Sakai, and T. Shinohara, Synlett, 2000, 116; CrossRef b) H. Kotsuki, H. Sakai, J.-G. Jun, and M. Shiro, Heterocycles, 2000, 52, 661; CrossRef c) T. Ishii, S. Fujioka, Y. Sekiguchi, and H. Kotsuki, J. Am. Chem. Soc., 2004, 124, 9558; CrossRef d) K. Ko, K. Nakano, S. Watanabe, Y. Ichikawa, and H. Kotsuki, Tetrahedron Lett., 2009, 50, 4025. CrossRef
6. a) G. Höfle, W. Steglich, and H. Vorbrüggen, Angew. Chem., Int. Ed. Engl., 1978, 17, 569; CrossRef b) E. F. V. Scriven, Chem. Soc. Rev., 1983, 129; CrossRef c) U. Ragnarsson and L. Grehn, Acc. Chem. Res., 1998, 31, 494; CrossRef d) D. J. Berry, C. V. Digiovanna, S. S. Metrick, and R. Murugan, ARKIVOC, 2001, i, 201; e) R. Murugan and E. F. V. Scriven, Aldrichimica Acta, 2003, 36, 21; f) A. C. Spivey and S. Arseniyadis, Angew. Chem. Int. Ed., 2004, 43, 5436. CrossRef
7. a) P. R. Schreiner, Chem. Soc. Rev., 2003, 32, 289; CrossRef b) Y. Takemoto, Org. Biomol. Chem., 2005, 3, 4299; CrossRef c) T. Akiyama, J. Itoh, and K. Fuchibe, Adv. Synth. Catal., 2006, 348, 999; CrossRef d) M. S. Taylor and E. N. Jacobsen, Angew. Chem. Int. Ed., 2006, 45, 1520; CrossRef e) S. J. Connon, Chem. Eur. J., 2006, 12, 5418; CrossRef f) A. G. Doyle and E. N. Jacobsen, Chem. Rev., 2007, 107, 5713; CrossRef g) H. Miyabe and Y. Takemoto, Bull. Chem. Soc. Jpn., 2008, 81, 785; CrossRef h) S. J. Connon, Chem. Commun., 2008, 2499; CrossRef i) S. J. Connon, Synlett, 2009, 354; CrossRef j) K. Etzenbach-Effers and A. Berkessel, Top. Curr. Chem., 2010, 291, 1. CrossRef
8. B. C. Ranu, S. K. Guchhait, K. Ghosh, and A. Patra, Green Chem., 2000, 5. The relative stereochemistry of this compound is unclear. CrossRef
9. Evans pKa Table. Available online: http://www2.lsdiv.harvard.edu/labs/evans/.
10. E. Chrystiuk and A. Williams, J. Am. Chem. Soc., 1987, 109, 3040. CrossRef
11. It can not preclude the possibility that the Michael acceptors could also be activated by a thiourea catalyst.
12. For our recent work in this field, see: M. Moritaka, N. Miyamae, K. Nakano, Y. Ichikawa, and H. Kotsuki, Synlett, 2012, 23, 2554. CrossRef
13. Thiourea A: F. Z. Dorwald, J. B. Hansen, J. P. Mogensen, T. M. Tagmose, B. Pirotte, P. Lebrun, P. De Tullio, S. Boverie, and J. Delarge, US Pat 1997-55193; Thiourea B: H. Y. Kim and K. Oh, Org. Lett., 2011, 13, 1306; CrossRef Thiourea C: R. H. Shapiro, J. W. Serum, and A. M. Duffield, J. Org. Chem., 1968, 33, 243; CrossRef Thiourea D: I. J. P. De Esch, J. E. J. Mills, T. D. J. Perkins, G. Romeo, M. Hoffmann, K. Wieland, R. Leurs, W. M. P. B. Menge, P. H. J. Nederkoorn, P. M. Dean, and H. Timmerman, J. Med. Chem., 2001, 44, 1666. CrossRef
14. R. L. Willer and E. L. Eliel, J. Am. Chem. Soc., 1977, 99, 1925. CrossRef
15. T. Caruso, M. Feroci, A. Inesi, M. Orsini, A. Scettri, and L. Palombi, Adv. Synth. Catal., 2006, 348, 1942. CrossRef
16. A. Soriente, R. Arienzo, M. De Rosa, L. Palombi, A. Spinella, and A. Scettri, Green Chem., 1999, 157. CrossRef
17. P. H. Lee, D. Seomoon, K. Lee, and Y. Heo, J. Org. Chem., 2003, 68, 2510. CrossRef
18. A. Mekonnen and R. Carlson, Eur. J. Org. Chem., 2006, 2005. CrossRef
19. B. C. Ranu and S. Bhar, Tetrahedron, 1992, 48, 1327. CrossRef