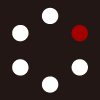
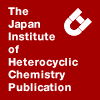
HETEROCYCLES
An International Journal for Reviews and Communications in Heterocyclic ChemistryWeb Edition ISSN: 1881-0942
Published online by The Japan Institute of Heterocyclic Chemistry
e-Journal
Full Text HTML
Received, 14th September, 2013, Accepted, 15th October, 2013, Published online, 24th October, 2013.
DOI: 10.3987/COM-13-S(S)116
■ Bi(OTf)3 as a Dual Role Catalyst. Synthesis of Substituted Morpholine Derivatives via Catalytic O-Allylation
Ryuji Hayashi, Jin-A Park, and Gregory R. Cook*
Department of Chemistry and Biochemistry, North Dakota State University, Fargo, North Dakota 58108, U.S.A.
Abstract
The diastereoselective synthesis of cis-1,4-disubstituted morpholines has been accomplished in good to excellent yields via Bi(OTf)3 catalyzed ring-closing O-allylation under mild conditions without the need for added base. The bismuth Lewis acid catalyst appeared to play two roles; the mild deprotection of a silyl ether and the Lewis acid activation of an allylic halide for mild nucelophilic cyclization. Substituted morpholines were obtained with diastereoselectivity ranging from 2:1 to >99:1.INTRODUCTION
Substituted morpholine derivatives are found in many natural and unnatural products and their activity in pharmacology is very attractive.1 To name a few, they are known to be potentially useful as antidepressants,2 appetite suppressants,3 antitumor agents,4 antibacterials,5 and antioxidants.6 Therefore, the efficient synthesis of novel substituted morpholine derivatives opens the door to explore new drug targets. There have been a substantial amount of examples of the synthesis of morpholine derivatives, typically prepared from amino alcohols7 and epoxides.8 In order to generate a new carbon-oxygen bond, O-alkylation of alcohols with alkyl halides is one of the most useful strategies. O-Alkylation generally requires at least a stoichiometric amount of base for both deprotonation and neutralization of acid byproducts (HCl, HBr, HI).9 Thus, catalytic conditions that require no base are highly desirable.
During our studies of mild Lewis acid catalyzed halide activation, we developed an atom transfer cyclization10 catalyzed by In(III). The reaction was driven by catalytic allylic halide activation under mild conditions. We have also discovered other C-C bonds formations via catalytic halide activation using Lewis acids including ring-closing Friedel-Crafts reactions,11 intermolecular Friedel-Crafts reactions,12 and 5-exo-trig cyclization reactions.13 However, nucleophilic addition of heteroatoms in this type of activation has not been demonstrated. Herein, we present a catalytic O-alkylation via halide activation with Lewis acids in the absence of base14 for the preparation of substituted morpholine derivatives.
RESULTS AND DISCUSSION
Our initial investigation examined the preparation of 2-vinyl morpholine 2 from compound 1a, which was prepared in three steps from ethanolamine (Table 1). The first question we addressed was whether a simple cleavage of the TBS group in 1a would automatically cyclize to yield 2 (Table 1). Unexpectedly, when treated with 1.2 equivalents of CsF in MeCN (entry 1) no reaction was observed. When the reaction was carried out in DMF (entry 2), the starting material decomposed. Using 2 equivalents of TBAF in THF15 (entry 3) resulted in only 6% yield of the desired morpholine 2. On the other hand, acidic conditions appeared to be slightly better. Application of a stoichiometric amount of triflic acid16 (entry 4) resulted in 39% yield of 2. Clearly simple deprotection of TBS with basic fluoride sources to reveal a nucleophilic alkoxide was not efficient. Acidic conditions afforded an increased yield of 2 but not in a useful range presumably due to the low nucleophilicity of the alcohol. Therefore, we considered that activation of allylic halide 1a with Lewis acid would enhance the cyclization to yield 2. In the presence of 10 mol% of Bi(OTf)317 in CH2Cl2 at room temperature for 16 hours, the target morpholine 2 was produced in an encouraging 56% yield. Interestingly, when 4Å MS were added the reaction did not proceed and 1a was recovered nearly quantitatively (entry 6). This suggested that trace amounts of water may be required for successful reaction.
Having discovered that Bi(OTf)3 could catalyze the cyclization of 1a, we turned our attention to optimizing conditions using Lewis acid. The results of this investigation are summarized in Table 2. The use of catalytic In(III) or In(I) salts in dichloromethane resulted in the formation of morpholine 2, albeit in low to moderate yields (entries 1-4). In all cases the major product obtained was the deprotected alcohol 1b. As Bi(OTf)3 appeared to be a better catalyst for the reaction we examined the role of catalyst amount, solvent and temperature using this promoter. Using 5 mol% of Bi(OTf)3 at room temperature morpholine 2 was obtained in 31% yield along with 66% of the alcohol 1b (entry 5). Increasing the catalyst loading to 10 mol% resulted in increased cyclization (56% yield) (entry 6). Other solvents demonstrated variable results. When the reaction was run in THF, DMF and water (entries 7, 12, 13), the starting material was recovered intact. Presumably the overwhelming amount of water in the latter case interfered with the Lewis acid’s ability to deprotect the silyl ether. In MeCN and EtOH (entries 8 and 14) at room temperature, deprotection ensued to form about 80% of the free alcohol18 1b along with very low yields of 2. The use of EtOH at 70 °C resulted in decomposition of the starting material (entry 15). In
contrast, MeNO2, toluene, dibromomethane, and dichloroethane yielded 2 in moderate to good yield (entries 9, 11, 16 and 17). As dichloroethane seemed to be the optimal solvent for this reaction, we investigated the role of catalytic loading and temperature in this solvent. Utilizing only 2 mol% Bi(OTf)3 in dichloroethane for 2 hours gave the morpholine 2 in only 34% along with 62% of the free alcohol 1b. Interestingly, simply increasing the reaction time to 16 hours under the same conditions afforded 88% of the pure morpholine 2. This suggested that the Lewis acid initially catalyzed the deprotection of the silyl ether and cyclization proceeded after formation of the alcohol. The Bi(OTf)3 presumably played a dual role to activate the allylic halide to yield 2.
Having established the conditions for optimal cyclization using Bi(OTf)3 catalysis, we invested the scope and limitation of the substrate with regards to O-protecting group and diastereoselectivity (Table 3). First, we investigated the role of the O-protecting group. The optimal conditions with the TBS-protected substrate 1a afforded 88% yield (entry 1). Using the free alcohol 1b directly (entry 2) provided the cyclized product 2 in quantitative yield supporting our hypothesis that deprotection occured first followed by cyclization of the alcohol. Interestingly, without catalyst, 1b did provide some morpholine 2 in low
yield (entry 3) but the reaction was not nearly as efficient as that with the catalyst present. Allyl or benzyl protected substrates 1c and 1d decomposed under the standard reaction conditions (entries 4 and 5). The TES-protected alcohol 1e underwent cyclization in good yield (entry 6). Allylic chloride substrates also performed well under the reaction conditions however, the yield was slightly lower (77%, entry 7). Increasing the catalyst loading from 2 to 5 mol% resulted in an increased yield of 88% (entry 8).
Placing substituents α- to the nitrogen resulted in diastereoselective cyclization favoring the cis isomer in all cases. A bulky t-butyl group was optimal giving rise to a single diastereomer (entry 9). Diastereoselectivity was dependent on the size of the substituent. An isopropyl group afforded a cis:trans ratio of 15:1 (entries 10 and 11) while a smaller methyl group only afforded 2:1 selectivity (entries 12 and 13). Benzyl gave 3:1 selectivity (entries 14 and 15). Optical activity was completely preserved using chiral substrates as shown in Scheme 1.
In order to gain more understanding about the role of the catalyst in the Bi(OTf)3-catalyzed cyclization, we examined the diastereoselectivity of the reaction under different conditions. The results are summarized in Table 4. Interestingly, the cyclization of alcohol 1h under the reaction conditions without the catalyst resulted in the formation of 4 in 60% yield but with lower diastereoselectivity (5:1, entry 1). Under base conditions at room temperature using NaH a similar 6:1 ratio of isomers was obtained (entry 2). Comparing the Bi-catalyzed reaction (entry 3) to these suggests that the catalyst was intimately involved in the cyclization step and probably organized the transition state in a controlled fashion. Based on these observations we hypothesize a reaction with Bi(OTf)3 playing a dual role as described in Scheme 2. Initially the catalyst, most likely with the assistance of a trace of water,15 catalyzed the deprotection of the silyl ether to form the free alcohol. The Lewis acid could subsequently activate the allylic halide for allylic substitution by the alkoxide. As higher diastereoselectivity was observed in the presence of the Lewis acid, a transition state such as 8 is plausible. The Bi metal could simultaneously coordinate the alcohol (or alkoxide) while activating the halide. A trans-decalin-like transition state would explain the preference for the cis stereochemistry observed in the product. The R-substituent would prefer an axial orientation due to the bulky equatorial N-tosylate group that would disfavor the R-group from adopting an equatorial position (7).19 The axial substituent is further accommodated by the lack of a 1,3-diaxial interaction due to the oxygen in the ring.
In conclusion, we have demonstrated that Bi(OTf)3 can catalyze the ring-closing O-allylation under mild conditions in the absence of base. A variety of substituted morpholine derivatives were prepared from amino alcohols using only 2 mol% of catalyst. When the substituent on the amino alcohol was bulky, good cis-diastereoselectivity was obtained Importantly, the Bi(OTf)3 catalyst appeared to play a dual role in this reaction, catalyzing the deprotection of a silyl ether and activating the allylic halide while organizing a tightly controlled transition state.
EXPERIMENTAL
Thin layer chromatography (TLC) was performed on silica gel Whatman-60F glass plates, and components were visualized by illumination with UV light or by staining with potassium permanganate solution. Chromatography was performed using E. Merck silica gel 60 (230-400 mesh). NMR spectra were recorded in DMSO-d6 or CDCl3 on a Varian Inova 500 MHz or 400 MHz spectrometer. 13C NMR was recorded using broadband proton decoupling. Chemical shifts are reported relative to TMS and coupling constants in Hz. Optical rotations were recorded on a JASCO-CIP-370 instrument. High-resolution mass spectra (HRMS) [Positive Ion FAB (FAB+) or Electrospray (ES)] were obtained in the Department of Chemistry and Biochemistry at North Dakota State University. Melting points were determined without correction. Reactions were carried out under an inert atmosphere of nitrogen or argon. Solvents were dried using a nitrogen-pressurized alumina column system from Solvtek.
General procedure for the synthesis of 1a-1m. Beginning with amino alcohols, the nitrogen was protected by tosylation and the alcohol was protected by silylation according to literature methods.20 The N-tosly-O-silyl amino alcohol (1.0 eq) and NaH (1.5 eq.) were stirred in THF (0.5M) at 0 ºC for 30 min under argon. 1,4-Dibromobutene (2.5 equiv) in THF (1 M) was added dropwise into the mixture and stirred for 16 h (if R was not H, reactions were stirred at 60 ºC). Saturated aqueous NH4Cl was added and the organic layer separated. The remaining aqueous layer was then extracted with CH2Cl2 twice, the combined organic fractions dried with Na2SO4, and concentrated in vacuo. The crude product was then purified by chromatography over silica gel (10% EtOAc/Hexanes) to afford the O-TBS-protected substrates.
General procedure for the synthesis of free alcohols: The O-TBS-protected allylic halides 1 and EtOH (0.5M) were placed in a small round bottom flask equipped with a magnetic stirring bar. Bi(OTf)3 (5 mol%) was added and the reaction was allowed to stir at rt for 16 h. Saturated aqueous NH4Cl and CH2Cl2 were added and the organic layer separated. The remaining aqueous layer was then extracted with CH2Cl2 twice, the combined organic fractions dried with Na2SO4, and concentrated in vacuo. The crude product was purified by chromatography over silica gel (30% EtOAc/Hexanes) to afford the free alcohol.
(E)-N-(4-Bromobut-2-enyl)-N-[2-(tert-butyldimethylsilanyloxy)ethyl]-4-methylbenzenesulfonamide (1a). IR (neat) cm-1; 2928, 2361, 1598, 1471, 1344, 1256, 1205, 1160, 1091, 1006, 970, 925, 838, 779, 724, 667; 1H NMR (400 MHz, CDCl3) δ 7.68 (d, J = 8.4 Hz, 2H), 7.27 (d, J = 8.4 Hz, 2H), 5.75 (ddd, J = 7.6, 15.2, 22.8 Hz, 1H), 5.61 (ddd, J = 6.4, 15.2, 21.6 Hz, 1H), 3.85 (dd, J = 6.4, 14.0 Hz, 4H), 3.69 (t, J = 6.4 Hz, 2H), 3.20 (t, J = 6.4 Hz, 2H), 2.39 (s, 3H), 0.83 (s, 9H), 0.00 (s, 6H); 13C NMR (100 MHz, CDCl3) δ 148.7, 142.6, 135.8, 135.5, 135.1, 67.8, 55.8, 54.7, 36.8, 31.3, 27.0; HRMS Calcd for C19H32BrNO3SSi (M+Na+): 484.0953, found 484.0947.
(E)-N-(4-Bromobut-2-enyl)-N-(2-hydroxyethyl)-4-methylbenzenesulfonamide (1b). IR (neat) cm-1; 2923, 2291, 1598, 1437, 1336, 1207, 1157, 1089, 817, 722; 1H NMR (400 MHz, CDCl3) δ 7.69 (d, J = 8.0 Hz, 2H), 7.31 (d, J = 8.0 Hz, 2H), 5.80 (ddd, J = 7.6, 15.2, 22.8 Hz, 1H), 5.64 (ddd, J = 6.4, 15.2, 21.6 Hz, 1H), 3.86 (d, J = 7.2 Hz, 4H), 3.71 (t, J = 5.6 Hz, 2H), 3.22 (t, J = 5.6 Hz, 2H), 2.41 (s, 3H); 13C NMR (100 MHz, CDCl3) δ 143.9, 136.4, 130.8, 130.2, 130.1, 127.5, 61.4, 50.7, 50.2, 31.4, 21.7; HRMS Calcd for C13H18BrNO3S (M+Na+): 370.0088, found 370.0080.
(E)-N-(2-Allyloxyethyl)-N-(4-bromobut-2-enyl)-4-methylbenzenesulfonamide (1c). IR (neat) cm-1; 2922, 1723, 1597, 1494, 1449, 1341, 1159, 1090, 1020, 927, 888, 816, 741, 663; 1H NMR (400 MHz, CDCl3) δ 7.69 (d, J = 8.4 Hz, 2H), 7.28 (d, J = 7.6 Hz, 2H), 5.91 (m, 1H), 5.79 (m, 1H), 5.68 (ddd, J = 6.4, 10.4, 12.8 Hz, 1H), 5.17 (m, 2H), 3.93 (m, 4H), 3.85 (d, J = 6.4 Hz, 2H), 3.53 (t, J = 6.4 Hz, 2H), 3.30 (t, J = 6.0 Hz, 2H), 2.40 (s, 3H); 13C NMR (100 MHz, CDCl3) δ 143.5, 133.4, 131.6, 129.9, 128.8, 127.4, 119.1, 70.4, 69.4, 52.0, 46.7, 32.1, 21.7; HRMS Calcd for C16H22BrNO3S (M+Na+): 410.0401, found 410.1460.
(E)-N-(2-Benzyloxyethyl)-N-(4-bromobut-2-enyl)-4-methylbenzenesulfonamide (1d). IR (neat) cm-1; 2920, 2653, 2245, 1495, 1343, 1163, 1117, 874, 600, 550; 1H NMR (400 MHz, CDCl3) δ 7.73 (d, J = 8.4 Hz, 2H), 7.30-7.24 (m, 7H), 5.80-5.72 (m, 1H), 5.65-5.58 (m, 1H), 4.37 (s, 2H), 3.89 (d, J = 7.6, 2H), 3.73 (d, J = 5.2, 2H), 3.34-3.26 (m, 4H), 2.42 (s, 3H); 13C NMR (100 MHz, CDCl3) δ 143.5, 137.3, 136.7, 131.5, 129.9, 128.8, 128.7, 128.6, 128.0, 127.4, 70.3, 68.9, 53.0, 47.2, 32.1, 21.7; HRMS Calcd for C20H24BrNO3S (M+Na+): 460.0558, found 460.0558.
(E)-N-(4-Bromobut-2-enyl)-4-methyl-N-2-(triethylsilyloxy)benzenesulfonamide (1e). IR (neat) cm-1; 2956, 1458, 1342, 1158, 1090, 1005, 921, 815, 756, 720, 653, 550; 1H NMR (400 MHz, CDCl3) δ 7.68 (d, J = 8.4 Hz, 2H), 7.28 (d, J = 8.0 Hz, 2H), 5.76 (ddd, J = 7.6, 15.2, 22.8 Hz, 1H), 5.63 (ddd, J = 6.4, 15.2, 21.6 Hz, 1H), 3.86 (dd, J = 5.6, 12.0 Hz, 4H), 3.69 (t, J = 6.4 Hz, 2H), 3.21 (t, J = 6.0 Hz, 2H), 2.40 (s, 3H) 0.91 (t, J = 8.0 Hz, 9H), 0.58 (q, J = 9.5 Hz, 6H); 13C NMR (100 MHz, CDCl3) δ 143.5, 137.3, 130.5, 130.4, 130.0, 127.4, 62.2, 50.5, 49.5, 31.5, 29.9, 21.7, 6.9, 4.5; HRMS Calcd for C19H32BrNO3SSi (M+Na+): 484.0953, found 484.0959.
(E)-N-[2-(tert-Butyldimethylsilanyloxy)-ethyl]-N-(4-chlorobut-2-enyl)-4-methylbenzenesulfonamide (1f). IR (neat) cm-1; 2929, 2857, 1598, 1471, 1444, 1343, 1305, 1256, 1160, 1091, 1006, 971, 927, 837, 779, 727, 651; 1H NMR (400 MHz, CDCl3) δ 7.68 (d, J = 8.4 Hz, 2H), 7.28 (d, J = 8.0 Hz, 2H), 5.69 (ddd, J = 6.4, 15.2, 21.6 Hz, 1H), 5.63 (ddd, J = 6.0, 15.2, 21.2 Hz, 1H), 3.96 (d, J = 6.8 Hz, 2H), 3.88 (d, J = 7.2 Hz, 2H), 3.70 (t, J = 6.0 Hz, 2H), 3.21 (t, J = 6.4 Hz, 2H), 2.4 (s, 3H), 0.84 (s, 9H), 0.01 (s, 6H); 13C NMR (100 MHz, CDCl3) δ 143.5, 137.4, 130.1, 130.0, 129.9, 127.4, 62.6, 50.6, 49.4, 44.1, 26.1, 21.7, 18.4; HRMS Calcd for C19H32ClNO3SSi (M+Na+): 440.1458, found 440.1460.
(E)-N-(4-Bromobut-2-enyl)-N-(1-hydroxymethyl-2,2-dimethyl-propyl)-4-methylbenzenesulfonamide (1g). IR (neat) cm-1; 3530, 2965, 2254, 1598, 1495, 1477, 1436, 1330, 1204, 1156, 1090, 1021, 911, 886, 815, 735, 660; 1H NMR (400 MHz, CDCl3) δ 7.72 (d, J = 8.4 Hz, 2H), 7.24 (d, J = 8.0 Hz, 2H), 5.81-5.71 (m, 2H), 3.86 -3.60 (m, 7H), 2.37 (s, 3H), 1.76 (s, 1H), 0.88 (s, 9H); 13C NMR (100 MHz, CDCl3) δ 171.4, 143.6, 137.8, 132.9, 129.6, 128.9, 128.2, 68.1, 60.6, 59.3, 45.8, 34.8, 32.0, 29.3, 28.5, 21.7, 21.2, 14.4; HRMS Calcd for C17H32BrNO3S (M+Na+): 426.0714, found 426.0704; [α]D25 -6.0726 (c 0.46, CH2Cl2).
(E)-N-(4-Bromobut-2-enyl)-N-(1-hydroxymethyl-2-methylpropyl)-4-methylbenzenesulfonamide (1h). IR (neat) cm-1; 3521, 2967, 1599, 1470, 1332, 1215, 1152, 1093, 1011, 890, 815, 754, 669; 1H NMR (400 MHz, CDCl3) δ 7.72 (d, J = 8.4 Hz, 2H), 7.27 (d, J = 8.0 Hz, 2H), 5.87-5.76 (m, 2H), 3.97 (dd, J = 5.2, 16.8 Hz, 1H), 3.88 (d, J = 6.4 Hz, 2H), 3.78 (m, 2H), 3.59 (m, 2H), 2.43 (s, 3H), 1.82-1.73 (m, 2H), 0.90 (d, J = 6.8 Hz, 3H), 0.68 (d, J = 6.8 Hz, 3H); 13C NMR (100 MHz, CDCl3) δ 143.6, 132.7, 129.8, 129.4, 127.6, 66.5, 62.3, 45.7, 31.8, 28.2, 21.7, 20.8, 20.3; HRMS Calcd for C16H24BrNO3S (M+Na+): 412.0558, found 412.0567.
(E)-N-(4-Bromobut-2-enyl)-N-[1-(tert-butyldimethylsilanyloxymethyl)-2-methylpropyl]-4-methylbenzenesulfonamide (1i). IR (neat) cm-1; 2929, 2360, 1464, 1338, 1154, 1090, 1009, 979, 895, 866, 838, 755, 686, 655; 1H NMR (400 MHz, CDCl3) δ 7.68 (d, J = 8.4 Hz, 2H), 7.24 (d, J = 8.0 Hz, 2H), 5.80-5.70 (m, 2H), 4.04-3.98 (m, 1H), 3.87-3.83 (m, 3H), 3.64 (dd, J = 4.8, 10.8 Hz 1H), 3.52-3.41 (m, 2H), 2.40 (s, 3H), 2.03-1.90 (m, 1H), 0.92 (d, J = 6.8 Hz, 3H), 0.87 (d, J = 6.4 Hz, 3H), 0.80 (s, 9H), -0.07 (d, J = 4.8, 6H); 13C NMR (100 MHz, CDCl3) δ 143.1, 138.8, 133.6, 129.6, 128.3, 127.6, 65.8, 63.5, 46.3, 32.1, 27.6, 26.0, 21.6, 20.8, 20.1, 18.4; HRMS Calcd for C22H38BrNO3SSi (M+Na+): 526.1423, found 526.1415; [α]D25 -72.149 (c 0.91, CH2Cl2); the enantiomeric purity was determined by HPLC (254 nm, 25 ºC) tR = 47.3 min (minor); tR = 49.6 min (major) [Chiralcel AD-H + AD-H (0.46 cm x 25 cm) (from Daicel Chemical Ind., Ltd.) hexane/i-PrOH, 99/1, 1.0 ml/min] as 99% ee.
(E)-N-(4-Bromobut-2-enyl)-N-(1-hydroxypropan-2-yl)-4-methylbenzenesulfonamide (1j). IR (neat) cm-1; 3527, 2977, 2361, 1598, 1494, 1437, 1333, 1206, 1152, 1091, 1050, 1008, 969, 911, 870, 815, 728, 657; 1H NMR (500 MHz, CDCl3) δ 7.74 (d, J = 8.0 Hz, 2H), 7.32 (d, J = 8.0 Hz, 2H), 5.89 (ddd, J = 7.5, 15.5, 22.0 Hz, 1H), 5.83 (ddd, J = 6.5, 15.5, 22.0 Hz, 1H), 4.04-3.92 (m, 4H), 3.79 (dd, J = 7.0, 16.5 Hz, 1H), 3.59-3.51 (m, 2H), 2.44 (s, 3H), 2.00 (s, 1H), 0.98 (d, J = 7.0 Hz, 3H); 13C NMR (100 MHz, CDCl3) δ 143.7, 138.0, 132.8, 130.0, 129.4, 127.4, 64.8, 55.7, 44.8, 31.8, 21.8, 14.9, 14.4; HRMS Calcd for C14H20BrNO3S (M+Na+): 384.0245, found 384.0245; [α]D25 46.491 (c 0.33, CH2Cl2).
(E)-N-(4-Bromobut-2-enyl)-N-[2-(tert-butyldimethylsilanyloxy)-1-methylethyl]-4-methylbenzenesulfonamide (1k). IR (neat) cm-1; 3031, 2929, 2857, 2359, 1599, 1495, 1471, 1336, 1258, 1215, 1154, 1092, 1006, 968, 914, 838, 757, 654, 551; 1H NMR (500 MHz, CDCl3) δ 7.73 (d, J = 8.5 Hz, 2H), 7.30 (d, J = 6.0 Hz, 2H), 5.88-5.75 (m, 2H), 4.02-3.82 (m, 5H), 3.60 (dd, J = 5.5, 10.0 Hz, 1H), 3.52 (dd, J = 6.0, 10.0 Hz, 1H), 2.43 (s, 3H), 1.10 (d, J = 7.0 Hz, 3H), 0.87 (s, 9H), 0.02 (d, J = 2.5 Hz, 6H); 13C NMR (125 MHz, CDCl3) δ 214.8, 143.3, 133.6, 129.8, 128.6, 127.4, 66.1, 55.3, 45.3, 32.1, 26.1, 21.7, 15.9; HRMS Calcd for C20H34BrNO3SSi (M+Na+): 498.1110, found 498.1116; [α]D25 -2.454 (c 1.82, CH2Cl2).
(E)-N-(4-Bromobut-2-enyl)-N-(1-hydroxy-3-phenylpropan-2-yl)-4-methylbenzenesulfonamide (1l). IR (neat) cm-1; 3527, 3028, 2924, 2252, 1598, 1495, 1454, 1333, 1205, 1157, 1094, 911, 815, 732, 701, 659; 1H NMR (400 MHz, CDCl3) δ 7.61 (d, J = 8.0 Hz, 2H), 7.24-7.17 (m, 5H), 7.04 (d, J = 6.4 Hz, 2H), 5.86 (ddd, J = 4.6, 15.2, 22.8 Hz, 1H), 5.76 (ddd, J = 6.0, 15.2, 21.2 Hz, 1H), 4.06-3.94 (m, 2H), 3.89-3.83 (m, 3H), 3.66-3.56 (m, 2H), 2.75 (dd, J = 4.8, 13.6 Hz, 1H), 2.67 (dd. J = 7.6, 13.6 Hz, 1H), 2.39 (s, 3H), 1.87 (s, 1H); 13C NMR (100 MHz, CDCl3) δ 143.7, 137.8, 137.7, 132.6, 129.9, 129.5, 129.3, 128.8, 127.5, 126.9, 62.7, 62.0, 46.0, 36.7, 31.8, 21.7; HRMS Calcd for C20H24BrNO3S (M+Na+): 460.0558, found 460.0558; [α]D25 -14.090 (c 0.15, CH2Cl2).
(E)-N-[1-Benzyl-2-(tert-butyldimethylsilanyloxy)-ethyl]-N-(4-bromobut-2-enyl)-4-methylbenzenesulfonamide (1m). IR (neat) cm-1; 2930, 1736, 1334, 1155, 838, 699, 550; 1H NMR (400 MHz, CDCl3) δ 7.71 (d, J = 8.0 Hz, 2H), 7.26-7.21 (m, 5H), 7.18-7.16 (m, 2H), 5.62-5.46 (m, 2H), 5.04 (t, J = 6.8 Hz, 1H), 4.11 (m, 1H), 4.00 (m, 1H), 3.77 (d, J = 7.2, 2H), 3.63 (m, 1H), 2.4 (s, 3H), 0.80 (s, 9H), 0.01 (s, 6H); 13C NMR (100 MHz, CDCl3) δ 143.4, 138.5, 137.1, 132.9, 129.8, 128.8, 128.7, 128.5, 128.0, 127.6, 63.5, 62.0, 46.3, 32.0, 26.0, 21.7, 18.4; HRMS Calcd for C26H38BrNO3SSi (M+Na+): 574.1423, found 574.1419; [α]D25 -20.548 (c 0.34, CH2Cl2).
General procedure for the Bi(OTf)3-catalyzed cyclization: Substrates 1 (0.1 – 0.3 mmol) and CH2Cl2 (0.05M) were placed in a small round bottom flask equipped with a condenser and a magnetic stir bar. Bi(OTf)3 (2 to 5 mol%) was added and the reaction was allowed to stir at 70 ºC. Upon completion of the reaction (16 h), the mixture was loaded on to a silica gel column directly and isolated using 10:1 hexane-EtOAc solution to afford morpholine product.
Cis-5-tert-butyl-4-(toluene-4-sulfonyl)-2-vinylmorpholine (3). IR (neat) cm-1; 2965, 2256, 1736, 1598, 1477, 1401, 1342, 1290, 1110, 1030, 966, 939, 813, 739, 672; 1H NMR (400 MHz, CDCl3) δ 7.71 (d, J = 8.0 Hz, 2H), 7.30 (d, J = 8.4 Hz, 2H), 5.65 (ddd, J = 5.6, 10.8, 16.4 Hz, 1H), 5.19-5.11 (m, 2H), 4.05 (d, J = 12.4 Hz, 1H), 3.68 (dd, J = 2.8, 18.0 Hz, 1H), 3.43 (d, J = 4.4 Hz, 1H), 3.35-3.25 (m, 2H), 3.20 (dd, J = 11.6, 14.8 Hz, 1H), 2.41 (s. 3H), 1.06 (s, 9H); 13C NMR (100 MHz, CDCl3) δ 143.7, 138.8, 135.2, 130.1, 127.3, 117.5, 65.1, 59.1, 47.2, 36.1, 29.3, 21.7; HRMS Calcd for C17H25NO3S (M+Na+): 346.1453, found 346.1457; [α]D25 5.294 (c 0.39, CH2Cl2).
Cis-5-isopropyl-4-(toluene-4-sulfonyl)-2-vinylmorpholine (4). IR (neat) cm-1; 2967, 1464, 1342, 1280, 1216, 1159, 1092, 1019, 971, 940, 909, 815, 756, 680, 557; 1H NMR (400 MHz, CDCl3) δ 7.71 (d, J = 8.4 Hz, 2H), 7.29 (d, J = 8.4 Hz, 2H), 5.69 (ddd, J = 5.6, 10.8, 16.4 Hz, 1H), 5.25-5.14 (m, 2H), 3.89 (dd, J = 2.4, 13.2 Hz, 1H), 3.68 (dd, J = 3.2, 14.8 Hz, 1H), 3.58-3.53 (m, 1H), 3.30 (dd, J = 2.8, 12.8 Hz, 2H), 2.94 (dd, J = 11.2, 14.4 Hz, 1H), 2.41 (s, 3H), 2.23-2.20 (m, 1H), 0.96 (d, J = 6.4 Hz, 3H), 0.90 (d, J = 6.8 Hz, 3H); 13C NMR (100 MHz, CDCl3) δ 143.5, 138.9, 135.1, 130.1, 127.2, 117.8, 66.3, 59.2, 45.6, 25.5, 21.7, 20.2, 20.0; HRMS Calcd for C16H23NO3S (M+Na+): 332.1294, found 332.1294; [α]D25 7.692 (c 0.25, CH2Cl2); the enantiomeric purity was determined by HPLC (254 nm, 25 ºC) tR = 81.8 min (major); tR = 84.6 min (minor) [Chiralcel AD-H + AD-H (0.46 cm x 25 cm) (from Daicel Chemical Ind., Ltd.) hexane/i-PrOH, 99/1, 1.0 ml/min] as 99% ee.
(Mixture of 1:2 cis/trans)-5-methyl-4-(toluene-4-sulfonyl)-2-vinylmorpholine (5). IR (neat) cm-1; 3033, 2980, 2864, 1918, 1599, 1494, 1444, 1341, 1161, 966, 815, 680, 550; 1H NMR (500 MHz, CDCl3) δ 7.72 (d, J = 8.5 Hz, 2H), 7.36 (d, J = 8.0 Hz, 2H), 5.86-5.74 (m, 1H), 5.39 (dd, J = 7.5, 25.0 Hz, 1H), 5.29-5.24 (d, J = 13.5 Hz, 1H), 4.00 (q, J = 6.5 Hz, 1H), 3.88-3.86 (m, 1H), 3.73-3.57 (m, 3H), 2.89 (t, J = 12.0 Hz), 2.45 (s, 3H), 1.12 (d, J = 6.5 Hz); 13C NMR (125 MHz, CDCl3) δ 144.0, 143.7, 137.8, 135.0, 134.8, 134.7, 130.2, 130.1, 130.0, 127.9, 127.3, 118.4, 118.0, 75.2, 72.4, 71.8, 71.4, 70.5, 51.8, 49.0, 48.5, 44.5, 43.9, 21.8, 15.8, 13.9 d; HRMS Calcd for C14H19NO3S (M+Na+): 304.0983, found 304.0976; [α]D25 7.692 (c 0.25, CH2Cl2).
Cis-5-benzyl-4-(toluene-4-sulfonyl)-2-vinylmorpholine (6). IR (neat) cm-1; 3028, 2925, 2865, 2254, 1733, 1599, 1496, 1455, 1342, 1304, 1163, 1112, 1090, 1054, 959, 911, 815, 734; 1H NMR (400 MHz, CDCl3) δ 7.62 (d, J = 8.0 Hz, 2H), 7.31-7.12 (m, 7H), 5.83 (ddd, J = 5.6, 10.8, 16.4 Hz, 1H), 5.39 (d, J = 17.6 Hz, 1H), 5.26 (d, J = 10.8 Hz, 1H), 4.00 (dd, J = 3.6, 8.0 Hz, 1H), 3.88-3.85 (m, 1H), 3.71-3.55 (m, 2H), 3.47 (dd, J = 2.8, 11.6 Hz, 1H), 3.04 (q, J = 10.4 Hz, 2H), 2.69 (dd, J = 4.8, 12.8 Hz, 1H), 2.39 (s, 3H); 13C NMR (100 MHz, CDCl3) δ 143.7, 138.0, 137.8, 135.0, 130.0, 129.7, 129.6, 128.9, 127.5, 127.3, 126.8, 119.2, 118.1, 72.7, 67.3, 62.2, 55.9, 54.3, 45.1, 34.4, 34.2, 21.7 4 d ; HRMS Calcd for C20H23NO3S (M+Na+): 380.1296, found 380.1302; [α]D25 37.008 (c 0.36, CH2Cl2).
ACKNOWLEDGEMENTS
Dedicated to my very good friend Prof. Victor Snieckus on the occasion of his 77th birthday. Once a cardinal, always a cardinal. We are grateful to the National Science Foundation (NSF-CHE-0616485, NSF-CHE-1012295) and the NDSU NIH Center for Protease Research (NCRR-P20-RR15566) for financial support of this project.
References
1. R. Wijtmans, M. K. S. Vink, H. E. Schoemaker, F. L. V. Delft, R. H. Blaauw, and F. P. J. T. Rutjes, Synthesis, 2004, 641. CrossRef
2. a) P. Melloni, A. Della Torre, E. Lazzari, G. Mazzini, and M. Meroni, Tetrahedron, 1985, 41, 1393; CrossRef b) Q. K. Fang, Z. Han, P. Grover, D. Kessler, C. H. Senanayake, and S. A. Wald, Tetrahedron: Asymmetry, 2000, 11, 3659; CrossRef c) J. L. Kelley, D. L. Musso, G. E. Boswell, F. E. Soroko, and B. R. Cooper, J. Med. Chem., 1996, 39, 347. CrossRef
3. a) R. B. Rothman, M. Katsnelson, N. Vu, J. S. Partilla, C. M. Dersch, B. E. Blough, and M. H. Baumann, Eur. J. Pharmacol., 2002, 51; CrossRef b) P. D’Arrigo, M. Lattanzio, G. P. Fantoni, and S. Servi, Tetrahedron: Asymmetry, 1998, 9, 4021. CrossRef
4. a) D. Kim, D. H. Ryu, J. Y. Lee, N. Lee, Y. Kim, J. Kim, K. Chang, G. Im, T. Kim, and W. Choi, J. Med. Chem., 2001, 44, 1594. CrossRef
5. K. Araki, T. Kuroda, S. Uemori, A. Moriguchi, Y. Ikeda, F. Hirayama, Y. Yokoyama, E. Iwao, and T. Yakushiji, J. Med. Chem., 1993, 36, 1356. CrossRef
6. a) C. Guilloneau, Y. Charton, Y. Ginot, M. Fouquier-d’ Hérouël, M. Bertrand, B. Lockhart, P. Lestage, and S. Goldstein, Eur. J. Med. Chem., 2003, 38, 1; CrossRef b) M. C. Chrysselis, E. A. Rekka, I. C. Siskou, and P. N. Kourounakis, J. Med. Chem., 2002, 45, 5406; CrossRef c) M. C. Chrysselis, E. A. Rekka, and P. N. Kourounakis, J. Med. Chem., 2000, 43, 609. CrossRef
7. a) M. M. Zhao, J. M. McNamara, G. Ho, K. M. Emerson, Z. J. Song, D. M. Tschaen, K. M. J. Brands, U. Dolling, E. J. J. Grabowski, and P. J. Reider, J. Org. Chem., 2002, 67, 6743; CrossRef b) K. M. J. Brands, J. F. Payack, J. D. Rosen, T. D. Nelson, A. Candelario, M. A. Huffman, M. M. Zhao, J. Li, B. Craig, Z. J. Song, D. M. Tschaen, K. Hansen, P. N. Devine, P. J. Pye, K. Rossen, P. G. Dormer, R. A. Reamer, C. J. Welch, D. J. Mathre, N. N. Tsou, J. M. McNamara, and P. J. Reider, J. Am. Chem. Soc., 2003, 125, 2129; CrossRef c) J. T. Lai, Synthesis, 1984, 122; CrossRef d) S. D. Rychnovsky, T. Beauchamp, R. Vaidyanathan, and T. Kwan, J. Org. Chem., 1998, 63, 6363; CrossRef e) G. Sun, P. S. Savle, R. D. Gandour, N. N. a’Bhaírd, R. R. Ramsay, and F. R. Fronczek, J. Org. Chem., 1995, 60, 6688; CrossRef f) Y. Uozumi, A. Tanahashi, and T. Hayashi, J. Org. Chem., 1993, 58, 6826; CrossRef g) J. Vernier, L. S. Hegedus, and D. B. Miller, J. Org. Chem., 1992, 57, 6914; CrossRef h) F. Berrée, A. Debache, Y. Marsac, and B. Carboni, Tetrahedron Lett., 2001, 42, 3591; CrossRef i) B. Jiang and M. Xu, Org. Lett., 2002, 4, 4077; CrossRef j) J. Lai, X. Shi, Y. Gong, and L. Dai, J. Org. Chem., 1993, 58, 4775; CrossRef k) T. Takemoto, Y. Iio, and T. Nishi, Tetrahedron Lett., 2000, 41, 1785. CrossRef
8. a) B. A. Lanman and A. G. Myers, Org. Lett., 2004, 6, 1045; CrossRef b) C. Corral, J. Lissavetzky, I. Manzanares, V. Darias, M. A. Expósito-Orta, J. A. Martín Conde, and C. C. Sánchez-Mateo, Bioorg. Med. Chem., 1999, 7, 1349; CrossRef c) E. Bouron, G. Goussard, C. Marchand, M. Bonin, X. Pannecoucke, J. Quirion, and H. Husson, Tetrahedron Lett., 1999, 40, 7227; CrossRef d) M. Somei, K. Aoki, Y. Nagaham, and K. Nakagawa, Heterocycles, 1995, 41, 5; CrossRef e) J. Nozulak, J. M. Vigouret, A. L. Jaton, A. Hofmann, A. R. Dravid, H. P. Weber, H. O. Kalkman, and M. D. Walkinshaw, J. Med. Chem., 1992, 35, 480; CrossRef f) B. M. Trost, T. L. Calkins, C. Oertelt, and J. Zambrano, Tetrahedron Lett., 1998, 39, 1713. CrossRef
9. F. A. Carey and R. J. Sundberg, Advanced Organic Chemistry Part B. 4th Ed., 2000, Kluwer Academic/ Plenum Publishers, New York.
10. G. R. Cook and R. Hayashi, Org. Lett., 2006, 8, 1045. CrossRef
11. R. Hayashi and G. R. Cook, Org. Lett., 2007, 9, 1311. CrossRef
12. M. Kaneko, R. Hayashi, and G. R. Cook, Tetrahedron. Lett., 2007, 48, 7085. CrossRef
13. R. Hayashi and G. R. Cook, Tetrahedron Lett., 2008, 49, 3888. CrossRef
14. For an example of a Bi(OTf)3-catalyzed substitution of allylic alcohols without base, see: H. Qin, N. Yamagiwa, S. Matsunaga, and M. Shibasaki, Angew. Chem. Int. Ed., 2007, 46, 409. CrossRef
15. E. W. Collington, H. Finch, and I. J. Smith, Tetrahedron Lett., 1985, 26, 681. CrossRef
16. L. A. Carpino and F. Nowshad, Tetrahedron Lett., 1993, 34, 7009. CrossRef
17. For a recent review of the utility of Bi(III) compounds in organic synthesis, see: J. M. Bothwell, S. W. Krabbe, and R. S. Mohan, Chem. Soc. Rev., 2011, 40, 4649. CrossRef
18. J. S. Bajwa, J. Vivelo, J. Slade, O. Repic, and T. Blacklock, Tetrahedron Lett., 2000, 41, 6021. CrossRef
19. N-Acyl substituents on piperidine rings are well known to favor a conformation where α-substituents adopt an axial orientation to avoid A-strain. See: (a) J. D. Brown, M. A. Foley, and D. L. Comins, J. Am. Chem. Soc., 1988, 110, 7445; CrossRef (b) P. Beak and W. J. Zajdel, J. Am. Chem. Soc., 1984, 106, 1010; CrossRef (c) F. Johnson, Chem. Rev., 1968, 68, 375. CrossRef
20. L. A. Gandon, A. G. Russell, T. Gueveli, A. E. Brodwolf, B. M. Kariuki, N. Spencer, and J. S. Snaith, J. Org. Chem., 2006, 71, 5198. CrossRef