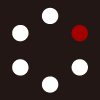
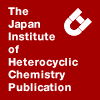
HETEROCYCLES
An International Journal for Reviews and Communications in Heterocyclic ChemistryWeb Edition ISSN: 1881-0942
Published online by The Japan Institute of Heterocyclic Chemistry
e-Journal
Full Text HTML
Received, 26th September, 2013, Accepted, 30th October, 2013, Published online, 1st November, 2013.
DOI: 10.3987/REV-13-783
■ Syntheses of Heterocycles via Alkyne Cycloadditions Catalyzed by Cyclopentadienylruthenium-Type Complexes
Yoshihiko Yamamoto*
Department of Basic Medicinal Sciences, Graduate School of Pharmaceutical Sciences, Nagoya University, Furo-cho, Chikusa-ku, Nagoya, Aichi 464-8601, Japan
Abstract
Transition-metal catalysis not only simplifies molecular assemblies by reducing the synthetic steps but also decreases waste production, thus accomplishing an efficient and environmentally benign synthesis of heterocycles. Ruthenium catalysis has made significant progress in the past decades, and its application to heterocycle synthesis has become one of the major research topics. In particular, organoruthenium complexes with a cyclopentadienyl-type ligand have been successfully utilized in various carbon-carbon and carbon-heteroatom bond formations. This review extensively surveys the synthetic methods of five- and six-membered heterocycles via the ruthenium-catalyzed cycloadditions of alkynes with other unsaturated compounds. The applications of the ruthenium-catalyzed heterocycle synthesis are also briefly outlined.CONTENTS
1. Introduction
2. Synthesis of Six-Membered Heterocycles
2-1. [2 + 2 + 2] Cycloadditions with Nitriles
2-2. [2 + 2 + 2] Cycloadditions with Heterocumulenes
2-3. Miscellaneous Cycloadditions with Ketones
3. Synthesis of Five-Membered Heterocycles
3-1. [2 + 2 + 1] Cycloadditions
3-2. [3 + 2] Cycloadditions with Azides
4. Applications of Ruthenium-Catalyzed Synthesis of Heterocycles
5. Summary
INTRODUCTION
Heterocyclic compounds are ubiquitous in natural products, pharmaceuticals, and advanced functional materials. Therefore, their efficient synthesis is one of the main research topics of organic chemistry. The conventional methods for heterocycle synthesis have relied on traditional condensation reactions under acidic or basic conditions; however, these methods lead to excessive waste production.1 In order to make heterocycle synthesis an environmentally benign process, transition-metal (TM)-catalyzed reactions have been developed because TM catalysts, in general, work under mild and/or neutral conditions, thus decreasing the waste production.2 Furthermore, TM catalysts enable the multicomponent assembly of heterocyclic structures from simple unsaturated precursors, thus simplifying the synthetic procedures. In this context, ruthenium catalysts have proved to be particularly versatile;3 e.g., ruthenium-based metathesis catalysts have become extremely popular in heterocycle synthesis via carbon-carbon bond formations.4 Apart from the metathesis chemistry, various types of ruthenium-catalyzed reactions can be employed for the fabrication of heterocyclic compounds via carbon-carbon and carbon-heteroatom bond formations.5 This review surveys the syntheses of heterocycles via the ruthenium-catalyzed cycloadditions of alkynes because their reactive carbon-carbon triple bonds enable multiple carbon-carbon and carbon-heteroatom bond formations,6 with particular attention to the ruthenium catalysts containing cyclopentadienyl (Cp)-type ligands. This is because the steric shielding by the Cp-type ligands plays a critical role not only in stabilizing the active ruthenium catalysts but also in controlling the product selectivity.3,7 This review reports the examples involving carbon-heteroatom bond formations, and therefore, heterocycle syntheses via only carbon-carbon bond formations have not been discussed here. The applications of the ruthenium-catalyzed heterocycle synthesis will also be briefly outlined.
2. SYNTHESIS OF SIX-MEMBERED HETEROCYCLES
2-1. [2 + 2 + 2] CYCLOADDITIONS WITH NITRILES
Since the pioneering studies of stoichiometric reactions of cobaltacyclopentadienes by Wakatsuki and Yamazaki,8 the TM-catalyzed [2 + 2 + 2] cycloadditions of two molecules of alkynes with nitriles or heterocumulenes have emerged as powerful tools for the synthesis of six-membered heterocycles such as pyridines and pyridones. Later, various TM complexes have been investigated to identify efficient catalysts for these useful synthetic processes.9 The earliest examples of cobalt-catalyzed intermolecular [2 + 2 + 2] cycloadditions of alkynes with nitriles affording pyridines were independently reported by the Yamazaki and Bönneman groups.10 The Vollhardt group reported the intramolecular variants using the cobalt catalyst.11 In contrast, ruthenium catalysts had not been much explored until recently. Early attempts of the ruthenium-catalyzed intermolecular [2 + 2 + 2] cycloaddition of alkynes with nitriles were not successful; the desired pyridines were not obtained with acceptable yield and selectivity.12 In 2001, Itoh and coworkers reported that electron-deficient nitriles underwent partially intramolecular [2 + 2 + 2] cycloaddition with 1,6-diynes to selectively afford bicyclic pyridines.13 The cycloaddition of malonate-derived 1,6-diyne 2 with nitriles 3, which have an acyl group such as R = CO2Et and COPh, in the presence of 2 mol% ruthenium catalyst 1, which has a pentamethylcyclopentadienyl (Cp*) ligand, proceeded smoothly at 60 ˚C for 0.5 h to afford bicyclic pyridines 4 in high yields (Scheme 1). In contrast, simple nitriles such as acetonitrile and benzonitrile failed to undergo cycloddition with 2 under the same conditions.
Similarly, nitriles with 2-furoyl or acetyl groups were employed to afford the corresponding pyridines in good yields, although increased catalyst loadings and a higher reaction temperature of 80 ˚C were required. In contrast, tosyl cyanide (R = Ts), trichloroacetonitrile (R = CCl3), and pentafluorobenzonitrile (R = C6F5) proved to be less efficient, affording moderate yields of pyridine derivatives (31%, 44%, and 67%, respectively). Increased loadings of these nitriles (3 equiv.) improved the product yields to 53%, 50%, and 80%, respectively. Various 1,6-diynes have been explored in this study, as shown in Figure 1, although lower yields were observed for diynes with a heteroatom tether.
The cycloaddition of unsymmetrical ether-tethered diyne 5 with cyanoformate 3 furnished pyridine regioisomers 6 and 7 in 83% combined yield with the ratio of 88:12 (Scheme 2a);14 thus, the cyanide was inserted in such a way that the steric repulsion between the terminal methyl substituent and the ethoxycarbonyl group was avoided. Interestingly, ester-tethered diyne 8 also exhibited a similar regioselectivity, affording pyridine regioisomers 9 and 10 in 83% combined yield with the ratio of 82:18 (Scheme 2b). In this case, the regioselectivity was electronically controlled by the internal carbonyl group, and the ethoxycarbonyl group was oriented para to the carbonyl group in the major regioisomer. Moreover, the steric and electronic effects cooperatively improved the regioselectivity for the cycloaddition of diyne 11, resulting in the formation of 12 and 13 in 84% combined yield with the ratio of 98:2 (Scheme 2c).
Moreover, Itoh and coworkers also reported exceptional reactivity of dicyanides toward the ruthenium-catalyzed [2 + 2 + 2] cycloaddition with 1,6-diynes.16 Diyne 2 and malononitrile (14, n = 1) underwent cycloaddition in the presence of 2 mol% catalyst 1 at 60 ˚C for 7 h to afford bicyclic pyridine 15 (n = 1) in 91% yield (Scheme 3). Furthermore, the above reaction proceeded even at room temperature; however, a prolonged reaction time (22 h) was required to afford 15 (n = 1) in 92% yield. Notably, one of the two cyano groups remained intact after the reaction, indicating that one cyano group coordinates to the catalyst, thus facilitating the incorporation of the other cyano group in the pyridine product. In good agreement with this observation, the distance between the two cyano groups was found to be critical. In fact, the reaction of homologous succinonitrile (14, n = 2) was found to be sluggish under similar conditions, affording the corresponding pyridine 15 (n = 2) in low yield (29%) because of the competitive [2 + 2 + 2] dimerization of the diyne. A similar effect of the distance between the cyano groups was observed for dicyanobenzenes 16; the reactivity decreased in the following order: o- > m- > p-isomers. In contrast, the reactivity of fumaronitrile (17) was comparable to that of malononitrile, even though 17 has a two-carbon tether similar to succinonitrile. This may be attributed to the rigidity of the ethylene tether.
Similar to cyano groups, halogens were also found to act as a coordinating group in the ruthenium-catalyzed [2 + 2 + 2] cycloaddition of 1,6-diynes and nitriles.17 Acetonitrile derivatives 18 with a halogen atom α to the cyano group were allowed to react with diyne 2 in the presence of 2 mol% 1 at room temperature to afford bicyclic pyridines 19 (Scheme 4). The chloro- and fluoro-substituted nitriles furnished 19 (X = Cl or F) in excellent isolated yields (90–93%). However, for bromoacetonitrile, 19 (X = Br) was obtained in a low yield (42%), probably because of the instability of the bromomethyl-substituted product 19. Various α-chloro nitriles furnished the corresponding pyridines in good yields (71–91%) as shown in Scheme 4. The functional moieties such as an aniline, alkenes, and even an alkyne, which is generally reactive toward the ruthenium-catalyzed cyclotrimerization, were tolerated in these reactions. When a dichlorodicyanide was used, the cyano group in the close proximity of the chlorine atoms selectively underwent cycloaddition.
The steric and electronic effects on regioselectivity were investigated (Scheme 5).18 The cycloaddition of unsymmetrical diyne 20, which has a terminal methyl group, with chloroacetonitrile (18) proceeded at room temperature for 3 h to afford bicyclic pyridine 21 as the exclusive product in 88% yield (Scheme 5a). The introduction of a terminal methyl substituent on 20 demonstrated a favorable effect not only on the regioselectivity but also on the chemoselectivity for the desired pyridine by preventing the dimerization of 20. In fact, the cycloaddition of 20 with less efficient dicyanides such as succinonitrile or glutaronitrile furnished the corresponding pyridines 22 in moderate-to-high yields (46–83%), and the use of phthalonitrile and o-chlorobenzonitrile furnished the desired biaryl products 23 in high yields (71–89%). In contrast, the cycloaddition of amide-tethered diyne 24 with 18 under the same conditions furnished regioisomeric pyridines 25 and 26 in an approximately 1:1 ratio, with almost complete loss of regioselectivity (Scheme 5b). Similar results were also obtained when malononitrile was used as the nitrile component. Thus, almost complete loss of the electronic directing effect in the halogen or cyano group-assisted [2 + 2 + 2] cycloadditions indicates that the reactions proceed by a different mechanism compared to the similar [2 + 2 + 2] cycloadditions using electron-deficient nitriles.
Other coordinating groups that assist [2 + 2 + 2] cycloaddition were also explored to expand the scope of nitrile components;18 e.g., methoxy- or methylthio-substituted acetonitrile derivatives 27 underwent cycloaddition with diyne 20 to afford bicyclic pyridines 28 in ca. 80% yields (Scheme 6a). Moreover, the cycloaddition of diyne 20 with propargylnitrile 29 afforded the corresponding pyridine 30 in 75% yield, indicating that the alkyne can work as a coordinating group (Scheme 6b). In contrast, dimethylaminoacetonitrile, acrylonitrile, and 3-pentenenitrile failed to undergo [2 + 2 + 2] cycloaddition. From these results, it was concluded that at least two lone pairs or π-bonds are necessary for the coordinating groups to be effective.
Besides the above partially intramolecular [2 + 2 + 2] cycloadditions, a fully intramolecular cycloaddition was achieved using cyanodiynes 31 as shown in Scheme 7.18 The desired tricyclic pyridines 32 were obtained in good yields (73–86%), although syringe pump addition was required to avoid intermolecular side reactions in the case of cyanodiynes with a terminal alkyne moiety (31, R = H).
A plausible mechanism for the ruthenium-catalyzed [2 + 2 + 2] cycloaddition of 1,6-diynes with electron-deficient nitriles has been suggested based on the density functional theory (DFT) calculations (Scheme 8).14,19 According to this mechanism, the cycloaddition starts with the oxidative cyclization of diyne complex 33 to produce ruthenacycle 34, which, because of the metal-carbon double bonds, undergoes the [2 + 2] cycloaddition with an electron-deficient nitrile to produce azatricycle complex 35. The DFT analysis showed that the electron-withdrawing group facilitates the [2 + 2] cycloaddition. The isomerization of 35 to 36 followed by reductive elimination produces η1-pyridine complex 37. Finally, the ligand exchange regenerates the initial diyne complex 33 and affords the cycloadduct, thus completing the catalytic cycle. On the other hand, the mechanism for the coordination-assisted [2 + 2 + 2] cycloaddition is unclear, even though a dinuclear pathway has been proposed to explain the role of the coordinating group.18
The ruthenium-catalyzed [2 + 2 + 2] cycloadditions of alkynes with nitriles affording pyridines have also been achieved using different catalyst systems. Saá and coworkers used cationic complex 38 in the presence of a chloride anion source, Et4NCl, in DMF.20 The cycloaddition of diyne 2 with malononitrile (14) or chloroacetonitrile (18) in the presence of 10 mol% catalyst proceeded at room temperature to successfully afford bicyclic pyridines 15 and 19 in 84% and 56% yields, respectively (Scheme 9). This catalyst system exhibited a similar reactivity profile as that of catalyst 1 for the cycloaddition of alkynes with nitriles with a coordinating group. When dicyanides were used as the nitrile component, one of the two cyano groups remained intact. Interestingly, ethylthiocyanide was allowed to react with diyne 2 at 80 ˚C to afford the corresponding pyridine in a moderate yield (53%). On the other hand, acrylonitrile was shown to be an incompetent nitrile component, affording cyclohexadiene 39 in a low yield (14%) because of the competing cycloaddition of the activated alkene moiety of acrylonitrile. Cationic catalyst 38 in the presence of Et4NCl was also effective for the intermolecular cyclocotrimerization of methyl propiolate (40) with electron-deficient nitriles 3 to exclusively afford 2,3,6-trisubstituted pyridines 41 in high yields of 82–89% (Scheme 10). In contrast, the same group reported that the attempted cycloaddition of malononitrile (14) with propiolate 40 failed to afford the corresponding pyridine, furnishing a regioisomer mixture of benzene triesters because of competitive cyclotrimerization of propiolate 40.
The Teplý group demonstrated the robustness of ruthenium complex 1 in the presence of air for a series of catalytic reactions.21 Thus, the [2 + 2 + 2] cycloaddition of diyne 42 with malononitrile (14) in the presence of air in 1,2-dichloroethane for 17 h furnished the desired bicyclic pyridine 43 in 80% yield, which was only slightly lower than that originally reported for the reaction under the argon atmosphere (Scheme 11a). The same reaction carried out in fetal bovine serum as the solvent with an increased catalyst loading furnished 43 in a moderate yield (57%), indicating the tolerance of catalyst 1 under biological conditions.22 When a water-soluble phosphine ligand, trisodium tris(3-sulfonatophenyl)phosphine (tppts, 44) was employed, the [2 + 2 + 2] cycloaddition of tosylamide-tethered diyne 45 with chloroacetonitrile (18) in water at 50 ˚C for 48 h furnished bicyclic pyridine 46 in 69% yield (Scheme 11b).23 However, the use of ether or malonate derivatives as the diyne substrates lowered the yields (44–59%). The coordination-assisted [2 + 2 + 2] cycloaddition of 1,6- and 1,7-diynes with dicyanides and halo nitriles were also carried out using Hoveyda-Grubbs catalyst.24
2-2. [2 + 2 + 2] CYCLOADDITIONS WITH HETEROCUMULENES
The TM-catalyzed [2 + 2 + 2] cycloaddition of two molecules of alkynes with isocyanates has been studied as a powerful method for synthesizing pyridones. Hong and Yamazaki, and Hoberg and Oster independently reported an intermolecular reaction, i.e, cyclocotrimerization of alkynes with isocyanates.25 Later, Vollhardt and Earl developed partially intramolecular versions using diynes and isocyanatoalkynes.26 In particular, the cycloaddition of isocyanatoalkynes with alkynes affording 2,3-dihydro-5(1H)-indolizinones was successfully applied to the total synthesis of antitumor agent camptothecin.26 Although the [2 + 2 + 2] cycloadditions of heterocumulenes have been less investigated than those of nitriles, several TM complexes have been identified as the catalysts for this reaction.9
In 2001, Itoh and coworkers reported the ruthenium-catalyzed [2 + 2 + 2] cycloaddition of 1,6-diynes with isocyanates to afford bicyclic pyridones.27 Because of the electron-deficient carbon-nitrogen double bonds in isocyanates, they exhibit similar reactivity as electron-deficient nitriles. Diyne 2 and N-aryl and N-alkyl isocyanates 47 underwent cycloaddition in the presence of 5 mol% of 1 in refluxing 1,2-dichloroethane for 1–2 h to afford the expected bicyclic pyridones 48 in 79–93% yields (Scheme 12). Four equivalents of isocyanates 47 were required to ensure complete conversion of 2 because they tend to decompose under the reaction conditions. Besides the malonate derivative 2, various 1,6-diynes were used for the pyridone synthesis except for the malononitrile-derived diyne, which furnished the product in a low yield (18%).
Isothiocyanates were also investigated as heterocumulene components instead of isocyanates by Itoh and coworkers.28 They reported that the carbon-sulfur double bond of isothiocyanates could be successfully incorporated in the [2 + 2 + 2] cycloaddition. Although 10 mol% of the catalyst was required, the reaction of diyne 2 with 1.2 equiv of isothiocyanates 49 in refluxing 1,2-dichloroethane furnished bicyclic thiopyranimines 50 in 50–88% yields (Scheme 13a). Isothiocyanates with N-substituents such as ester, ketone, phenyl, and cyclohexyl groups have also been used. The quaternary carbon center on the tether of the diyne substrates is essential for the cycloaddition probably because isothiocyanates strongly coordinate to the ruthenium center as soft ligands. It is assumed that the Thorpe-Ingold effect of the quaternary centers plays an important role in facilitating the coordination of the diyne substrates and their oxidative cyclization leading to the ruthenacycle key intermediates.29 In good agreement with this assumption, the increased loading of N-ethoxycarbonyl isothiocyanate lowered the yield of the cycloadduct. Besides isothiocyanates, carbon disulfide also participated in the [2 + 2 + 2] cycloaddition with a 1,6-diyne.28 Diyne 2 was heated in a mixture of carbon disulfide/1,2-dichloroethane (2:3 v/v) in the presence of 10 mol% 1 at 90 ˚C for 6 h to afford the desired bicyclic dithiopyran 51 in 50% yield along with 24% recovery of diyne 2 (Scheme 13b).
The regioselectivity of the [2 + 2 + 2] cycloadditions of N-propyl isocyanate (52) and N-phenyl isothiocyanate (54) has been investigated (Scheme 14).14 The reaction of unsymmetrical diyne 20 and 52 in the presence of 5 mol% 1 exclusively furnished pyridone 53 in 85% yield (Scheme 14a). Similarly, the reaction of 20 with isothiocyanate 54 in the presence of 10 mol% 1 furnished a 9:1 regioisomer mixture of 55 and 56 in a high combined yield of 82% (Scheme 14b). Besides the steric effect, the electronic effect was also investigated by carrying out the reaction of amide 24 with isocyanate 52 for 18 h to afford 57 and 58 with a lower yield (67%) and a lower regioselectivity of 82:18 (Scheme 14c).
The cyclocotrimerization of ethyl propiolate (40) and isocyanate 52 furnished regioisomeric pyridones 59 and 60 in 37% and 27% yields, respectively (Scheme 15).14 However, this fully intermolecular [2 + 2 + 2] cycloaddition was found to be less selective. Although a large excess of 52 (50 equiv) was used, the pyridone formation was accompanied by the cyclotrimerization of 40, leading to regioisomeric benzene triesters. Notably, two of the four possible regioisomers of the expected pyridones were selectively formed in the cyclocotrimerization of 40 with 52.
The mechanism of [2 + 2 + 2] cycloadditions of heterocumulenes was proposed based on the DFT calculations by Kirchner and coworkers.30 A model reaction of acetylene with isocyanic acid (HN=C=O) proceeds via ruthenacycle 61, which undergoes [2 + 2] cycloaddition with the carbon-nitrogen double bond of isocyanic acid to produce ruthenabicycle 62 followed by the isomerization of 62 to 63 via a 1,2-nitrogen shift from the ruthenium center to the neighboring carbene carbon (Scheme 16). Finally, the reductive elimination of pyridone from 63 produces 64. The transformation from 62 to 63 has the highest activation barrier. The reaction of isothiocyanic acid (HN=C=S) was also investigated to elucidate a similar mechanism.
The [2 + 2 + 2] cycloadditions of 1,6-diynes with isocyanates, isothiocyanate, and carbon disulfide were also carried out using Hoveyda-Grubbs catalyst.31 Regioselectivities were similar to those observed for the above examples using catalyst 1.
2-3. MISCELLANEOUS CYCLOADDITIONS WITH KETONES
As shown in the preceding sections, the ruthenium-catalyzed [2 + 2 + 2] cycloadditions involving the carbon-nitrogen triple bonds of nitriles and carbon-nitrogen and carbon-sulfur double bonds of heterocumulenes have been extensively explored. However, the catalytic cycloadditions involving the carbon-oxygen double bonds of ketones have attracted much less attention, probably because of the low coordinating ability of carbonyl groups to TM complexes. Stoichiometric and catalytic [2 + 2 + 2] cycloadditions of alkynes and ketones have been achieved using cobalt, zirconium, nickel, and rhodium complexes.32–35 Itoh and coworkers also reported the ruthenium-catalyzed [2 + 2 + 2] cycloaddition of 1,6-diynes with tricarbonyl compounds.36 The reaction of 1,6-diyne 2 with a highly electron-deficient ketone, ketomalonate 65, in the presence of 5 mol% 1 at 90 ˚C did not afford the desired bicyclic pyran 66 (R = H); however, the corresponding elecrocyclic ring-opening product 67 was obtained in a moderate yield of 46% (Scheme 17a). When unsymmetrical diyne 20 with a terminal methyl group was used as the substrate, dienylketone 68 was obtained as a ring-opened product with an improved yield of 75%. The substrate scope of the diyne is shown in Figure 2. Similar dienyl ketones were obtained as single products in 42–71% yields from the reactions of various unsymmetrical 1,6-diynes with ketomalonate 65. On the other hand, the reaction of diyne 69 with indanetrione 70 furnished two regioisomers 71 and 72 in 24% and 28% yields, respectively (Scheme 17b). Different carbonyl compounds such as ethyl pyruvate, diacetyl, and decafluorobenzophenone were also tested; however, none of them furnished the desired cycloaddition product. The mechanism of [2 + 2 + 2] cycloaddition of dipropargyl ether with ketomalonodialdehyde has been studied using the DFT calculations.37
The bicyclic 2H-pyrans obtained by [2 + 2 + 2] cycloaddition readily underwent electrocyclic ring opening to afford dienyl carbonyl compounds as shown above.34 In contrast, 4H-pyrans are stable at room temperature; thus, the ring opening reactions does not occur. Trost and co-workers reported interesting transformations of ω-alkynylenones using cationic ruthenium complex 73 as the catalyst.38 ω-Alkynylenone 74 (n = 1) underwent intramolecular oxa Diles-Alder cycloaddition in the presence of 5 mol% 73 at room temperature to afford bicyclic 4H-pyran 75 (n = 1) in 70% yield (Scheme 18). This fascinating [4 + 2] cycloaddition also proceeded with homologous substrate 74 (n = 2), although the yield of cyclohexane-fused product 75 (n = 2) decreased to 60%. This method tolerates sulfonamide, sulfone, and isopropyl ketones. However, a substrate with terminal alkyne moiety failed to afford the desired pyran. A plausible mechanism for this transformation was explained by the oxidative cyclization of ω-alkynylenone 78 leading to oxaruthenacycle 79 followed by reductive elimination to afford 80 (Scheme 19).
A formal [3 + 3] cycloaddition route to bicyclic 4H-pyrans was also developed by Nishibayashi and coworkers.39 They reported the ruthenium-catalyzed propargylic substitution of propargylic alcohols 82 with 1,3-cyclohexanedione 83 using a thiolate-bridged dinuclear complex 81 as the catalyst precursor (Scheme 20). The cycloaddition of 82 and 5 equiv of 83 in the presence of 5 mol% 81 and 10 mol% NH4BF4 at 60 ˚C for 1 h furnished cyclohexenone-fused pyrans 84 in high yields (86–99%). Electron-rich and electron-deficient aryl groups, 2-naphthyl group, and 2,2-diphenylvinyl group could be incorporated as the substituent R on the propargylic alcohol substrates 82. Besides 1,3-diketones such as 83 and 1,3-cyclopentanedione, tetronic acid and 4-hydroxycoumarin also worked well as the 1,3-dicarbonyl components. However, 1,3-cycloheptanedione, acetylacetone, and methyl acetoacetate furnished simple propargylic substitution products rather than the corresponding pyrans. A cationic dinuclear ruthenium complex, which was produced from the reaction of 81 and NH4BF4, was proposed as the catalyst. This cationic catalyst is presumed to convert propargylic alcohol 82 to allenylidene complex 85, which would then react with 1,3-cyclohexanedione at the γ carbon to produce vinylidene complex 86 (Scheme 21). Because of the keto-enol equilibrium of the 1,3-diketone moiety of 86, the hydroxyl group would attack the vinylidene α carbon to afford the final bicyclic pyrans via protonolysis of vinylruthenium 87.
3. SYNTHESIS OF FIVE-MEMBERED HETEROCYCLES
3-1. [2 + 2 + 1] CYCLOADDITIONS
Furans are important oxygen heterocycles found in natural products, pharmaceuticals, and advanced functional materials.1 Beller and co-workers have developed an efficient synthetic methodology to construct 2,5-diarylfurans form arylalkynes involving two TM-catalyzed reactions: (i) the ruthenium-catalyzed hydroalkoxylative dimerization of arylalkynes with alcohols, and (ii) the copper-catalyzed oxidative cyclization of the resulting dienyl ethers.40 Although this two-step protocol provides an efficient route to monocyclic furans, an alternative method involving the transformation of α,ω-diynes into bicyclic furans is also attractive. However, such a method has been confined to the stoichiometric oxidation via rhodacyclopentadienes and a single example of the palladium-catalyzed oxidative cyclization of 1,7-diphenyl-1,6-heptadiyne resulted in a low yield of the product.41,42 Therefore, no general catalytic protocol for the synthesis of bicyclic furans from α,ω-diynes existed previously.
Yamamoto and coworkers developed a new method for the preparation of diverse furans via [2 + 2 + 1] cycloaddition.43 The reaction of ether-tethered 1,6-diyne 85 and 5 equiv of dimethylsulfoxide (DMSO) in the presence of 3 mol% cationic ruthenium complex 73 in dimethylformamide (DMF) at 140 ˚C for 4 h furnished bicyclic furan 86 in 90% yield (Scheme 22a). In this novel [2 + 2 + 1] cycloaddition, the oxygen atom of the furan was derived from DMSO, as shown by the reaction of isolated ruthenacycle 87 with DMSO in the presence of AgPF6 to afford 86 in 65% yield as estimated from the NMR spectrum (Scheme 22b). The wide scope of this novel transfer-oxygenative cyclization is shown in Figure 3. Various functional groups such as esters, ketones, nitriles, and a sulfonamide on the tether were shown to be well tolerated in the [2 + 2 + 1] cycloaddition. Interestingly, the oxygen-atom transfer did not take place from a sulfone. As a terminal aryl group, both electron-deficient and electron-rich phenyl groups, 2-thienyl, and ferrocenyl groups could be used. Besides 1,6-diynes, 1,7-diynes could be also used, even though increased catalyst loadings were required because of the slower reactions. Furthermore, the double transfer-oxygenative cyclization of p-phenylene-tethered tetrayne 88 furnished a fascinating pentaryl product 89 in 87% yield, even though 10 mol% loading of catalyst 73 was required (Scheme 22c).
Besides diynes with terminal aryl groups, 1,6-diynes with terminal alkyl groups proved to be good substrates for the transfer-oxygenative cyclization.43 However, catalyst 73 did not work for these substrates. Instead, pentamethylcyclopentadienyl ruthenium complex 38 was found to be the optimal catalyst. Thus, malonate- or tosylamide-derived 1,6-diynes 90 with a terminal methyl group were subjected to transfer-oxygenative cyclization using 3 or 5 mol% 38 in THF at 70 °C to afford the desired bicyclic furans 91 in 90% and 74% yields, respectively (Scheme 23). Similarly, an ether derivative with terminal cyclopentyl groups furnished the corresponding furan in 61%, even though 15 mol% catalyst loading was necessary.
Yamamoto and coworkers proposed a mechanism as outlined in Scheme 24 based on the DFT calculations of the model complexes.43 The catalytic cycle involves the oxidative cyclization of DMSO-ligated diyne complex 92 to cationic ruthenacycle 93 followed by the concerted oxygen atom transfer from the DMSO ligand to one of the carbene carbons in 93 to generate ketocarbene complex 94, which subsequently cyclized to afford η4-furan complex 95. Finally, ligand exchange regenerates the starting diyne complex 92 and affords the cycloadduct, thus completing the catalytic cycle.
Wang and coworkers reported a similar [2 + 2 + 1] cycloaddition of α,ω-diynes affording bicyclic pyrroles using a palladium catalyst.44 However, the diyne substrates were limited to 1,8-di(arylethynyl)naphthalenes. Thus, the substrate scope of the [2 + 2 + 1] pyrrole synthesis remained to be investigated. Yamamoto and coworkers reported an example of formal [2 + 2 + 1] cycloaddition catalyzed by a ruthenium complex, although the described method lacks generality.14 They reported that the reaction of malonate-derived 1,6-diyne 2 with N-thionylaniline 96 might have formed [2 + 2 + 2] cycloadduct 97 (Scheme 25). However, the reaction of diyne 2 and large excess of 96 in the presence of 10 mol% 1 in 1,2-dichloroethane at 90 °C for 2 days furnished bicyclic pyrrole 98, which was presumably formed by the extrusion of SO from 97, albeit in a low yield (35%). The structure of 98 was unambiguously confirmed by X-ray crystallography.
3-2. [3 + 2] CYCLOADDITIONS WITH AZIDES
1,3-Dipolar cycloaddition of organic azides with alkynes, known as Huisgen cycloaddition, is a simple route to 1,2,3-triazoles.45 However, relatively high reaction temperatures (80–120 °C) and the regioselectivity issue limit the immense synthetic potential of this fascinating [3 + 2] cycloaddition over three decades. In 2002, Sharpless and coworkers reported the copper-catalyzed azido-alkyne cycloaddition (CuAAC) that dramatically changed the course of the Huisgen cycloaddition, exclusively affording 1,4-disubstituted regioisomers of 1,2,3-triazoles.46 Since 2002, the CuAAC have found diverse applications because the copper catalysis provides many synthetic advantages such as an impressive rate enhancement, a strict regiochemistry, diverse functional group compatibility, and a tolerance toward aqueous conditions.47 Thus, an alternative catalyst that enables the synthesis of 1,5-disubstituted 1,2,3-triazoles was elusive. To address this issue, Fokin and coworkers investigated the cycloaddition of benzyl azide 99 with phenylacetylene 100 in the presence of Ru(OAc)2(PPh3)2 in benzene at 80 °C for 4 h; the reaction exclusively furnished 1,4-disubstitued product 102 at 100% conversion of 99 (Scheme 26).48 In contrast, CpRuCl(PPh3)2 favored 1,5-disubstituted regioisomer 101 over 1,4-disubstituted regioisomer 102 with a ratio of 85:15, albeit in a lower conversion. Further, a similar ruthenium complex, Cp*RuCl(PPh3)2 (103), improved both the regioselectivity and conversion of 99, resulting in the exclusive formation of 1,5-disubstituted product 101. Other ruthenium complexes such as Cp*RuCl(cod) (1), Cp*RuCl(nbd) (nbd = norbornadiene), and [Cp*RuCl2]2 gave similar results.
Among the ruthenium complexes explored, Cp*RuCl(PPh3)2 (103) and Cp*RuCl(cod) (1) are particularly useful for the exclusive formation of 1,5-disubstituted 1,2,3-triazole regioisomers. Catalyst 103 is stable under air whereas catalyst 1 is more efficient because of the 1,5-cyclooctadiene ligand, which is more easily displaced than the triphenylphosphine ligand. The scope of the ruthenium-catalyzed azide-alkyne cycloaddition (RuAAC) using these representative catalysts is shown in Figure 4.49 The 1,5-disubstituted 1,2,3-triazole derivatives containing amino acids, nucleosides, nitrogen heterocycles, terpenes, steroids, and even phenylboronate moieties were obtained in good yields.
The main advantage of RuAAC over CuAAc is that internal alkynes can be used to obtain 1,4,5-trisubstituted triazoles. As shown in Figure 4, propargylic alcohols, an ynamide, and an alkynyl ketone were used as the internal alkyne components to afford highly functionalized triazoles in good yields.49 Interestingly, in each case, one of the two possible regioisomers was selectively produced in such a way that hydroxymethyl or electron-withdrawing substituents are placed at the C5 position of the 1,2,3-triazole ring.
Other research groups have also explored the scope of RuAAC, e.g., the cycloaddition of pyranosyl azide 104 with ynamide 105 catalyzed by 103 exclusively furnished 107 in 75% yield (Scheme 27a).50 Trifluoromethyl-substituted internal propargylic alcohol 108 also underwent cycloaddition with benzyl azide 99 to afford 109 in a high yield with 100% regioselectivity, thus orienting the hydroxymethyl group on the C5 of the triazole ring (Scheme 27b).51 In contrast, Majireck and Weinreb reported the complete loss of regioselectivity in the formation of triazole 111 when alkynyl acetal 110 was used, indicating that the electron-withdrawing or coordinating groups on the alkyne substrates are essential for the regioselective formation of triazoles (Scheme 27c).52 Hou and coworkers demonstrated the electronic effect on the regiochemistry of RuAAC; they studied the cycloaddition of azide 112 with diarylacetylene 113, which has both electron-rich and electron-deficient aryl groups (Scheme 27d).53 A significant regioselectivity of 4.1:1 in favor of regioisomer 114 was observed, and this selectivity was attributed to a favorable transition state, in which a carbon-nitrogen bond was formed between the azide terminal nitrogen and the more electrophilic alkyne carbon.
DFT calculations have been carried out by several research groups to better understand the mechanism of the regioselective formation of 1,5-disubstituted 1,2,3-triazoles.49,54,55 A simplified catalytic cycle proposed by Lin and coworkers is shown in Scheme 28.49 The spectator ligands L are expected to be displaced by a terminal alkyne and an azide to produce intermediate 116, where the alkyne ligates in a η2 fashion and the azide coordinates at its nitrogen atom substituted by the R2 group. The rate-determining oxidative coupling step in 116 produces bicyclic complex 117 via the carbon-nitrogen bond formation between the alkyne terminal carbon and the azide terminal nitrogen followed by reductive elimination to afford 16e triazole complex 118. Finally, the triazole ligand is replaced by the spectator ligands to regenerate 115 and affords the cycloadduct, thus completing the catalytic cycle.
In the above mechanism, the spectator ligands L are not required for the [3 + 2] cycloaddition. Therefore, the more facile the displacement of the neutral ligand L, the more efficient the catalyst Cp*RuClLn is, indicating that the vacant coordination sites on the ruthenium center are important for RuAAC. In good agreement with this assumption, Fokin and coworkers confirmed that a tetranuclear complex without any spectator ligand, [Cp*RuCl]4 (119), showed a better performance than the standard catalyst 103 in the diagnostic cycloaddition of benzyl azide 99 with phenylacetylene 100 (Scheme 29a).56 On the other hand, Nolan and coworkers screened a series of bulky phosphines and N-heterocyclic carbenes to identify a superior coordinatively unsaturated 16-electron complex as the catalyst.54 Thus, they reported that a monophosphine complex, Cp*RuCl(PiPr3) (120), exhibited a very high catalytic efficiency (Scheme 29a). The cycloaddition of 99 with propargylic alcohol 121 in the presence of 1 mol% 120 proceeded at room temperature within 1 min to quantitatively afford the desired triazole 122 (Scheme 29b). The cycloaddition of aryl azides is more challenging than that of alkyl azides such as 99. The Fokin group reported that microwave (MW) irradiation facilitated the cycloaddition of aryl azides 123 with phenylacetylene 100 (Scheme 29c).56 In fact, the reaction of phenyl azide 123 (Ar = Ph) and alkyne 100 under MW heating conditions (10 mol% 119, DMF, 110 °C, 20 min) furnished 124 in 87% yield, while the reaction conducted using a conventional heating source under the same conditions resulted in a lower yield of 52%. In the same manner, p-anisyl and p-chlorophenyl derivatives were obtained in 71% yields, while p-ethoxycarbonylphenyl and o-tolyl derivatives gave lower yields. The MW heating conditions were also successfully applied to the one-pot sequential process assembling 1,2,3-triazoles from sodium azide, primary alkyl halides, and alkynes.57 In this example, both the formation of alkyl azides and subsequent RuAAC were performed under MW irradiation.
The catalyst 119 was also applied to the intramolecular RuAAC of ω-alkynyl azides, leading to macrocyclic triazoles (Scheme 30).58 Although both higher dilution and temperature were required, the intramolecular RuAAC of 125 smoothly proceeded within 10 min to selectively afford 11- and 12-membered macrocycles 126 containing the 1,5-disubstituted triazoles in 58% and 67% yields, respectively. On the other hand, the intramolecular CuAAC of 125 furnished 12- and 13-membered macrocycles 127 in comparable yields owing to 1,4-disubstitued-triazole formation.
Fokin and coworkers further reported the implication of acetylide complexes in the RuAAC of terminal alkynes with azides.59 Moreover, a recyclable ruthenium catalyst was developed by the Astruc group.60 They prepared a magnetically separable ruthenium complex 131, which was immobilized on iron oxide nanoparticles using a phosphine ligand (Scheme 31). Therefore, they successfully repeated the standard cycloaddition of benzyl azide 99 with phenylacetylene 100 using 2 mol% 131 in THF at 65 °C to afford the desired triazole product 101 in good yields. The catalyst was recycled and found to be selective for at least five cycles.
4. APPLICATIONS OF RUTHENIUM-CATALYZED SYNTHESIS OF HETEROCYCLES
This section deals with the synthesis of diverse heterocycles as well as natural products whose syntheses include some steps closely related to ruthenium-catalyzed alkyne cycloadditions. Several illustrative compounds prepared via ruthenium-catalyzed alkyne cycloadditions will be presented.
As discussed in the Sections 2-1 and 2-2, the ruthenium-catalyzed [2 + 2 + 2] cycloadditions of α,ω-diynes with nitriles and isocyanates are powerful methods to assemble diverse bicyclic heterocycles. Yamamoto and coworkers utilized their ruthenium-catalyzed alkyne [2 + 2 + 2] cycloaddition to the synthesis of the spirocyclic C-arylglycoside core motif of naturally occurring antibiotic papulacandins.61 The synthesis of the heterocyclic analogs of this fascinating molecular target was attempted by the cycloaddition of glucose-derived unsymmetrical 1,6-diyne 132 with chloroacetonitrile 18 in the presence of 5 mol% 1 at room temperature to afford spirocyclic C-pyridylglycoside 133 in 83% yield as a single isomer (Scheme 32a). Moreover, C-heteroarylriboside analog 135 was also synthesized by the cycloaddition of ribose-derived unsymmetrical 1,6-diyne 134 with 18 (Scheme 32b). In this case, the expected β anomer 135 was obtained in 79% yield along with it's α anomer in 4% yield. In contrast, the reaction of 134 with isocyanate 52 exclusively furnished pyridone 136 in 84% yield (Scheme 32c).
Cyclothiazomycin is a member of the family of actinomycete thiopeptide antibiotics and features an interesting 2-thiazolylpyridine moiety. The Deiters group successfully synthesized 2-thiazolylpyridine building block 140 for the synthesis of cyclothiazomycin via temporary-tethered [2 + 2 + 2] cycloaddition (Scheme 33).62 Enantiopure 1,6-diyne 137, containing a C–Si–O tether, underwent cycloaddition with electron-deficient cyanothiazole 138 at 60 °C using catalyst 1 to afford pyridine cycloadduct 139 in 82% yield. Notably, a similar cycloaddition of a model diyne substrate with nitrile 138 did not proceed using cobalt-based catalysts.
The ruthenium-catalyzed [2 + 2 + 2] cycloaddition of diynes with electron-deficient nitriles selectively affords pyridines. Because the ester substituents on the resultant pyridine ring can be removed, cyanoformates can be used as the surrogates for hydrogen cyanide. Thus, Witulski, Nissen, Detert, and coworkers investigated the cycloadditions of yne-ynamides 141, 143, or 145, which possess terminal heterocyclic moieties, with methyl cyanoformate as an “HCN” surrogate to synthesize β-carboline structures (Scheme 34).63 Although this key transformation can be catalyzed by [Rh(cod)2]BF4/BINAP, ruthenium catalyst 1 was found to be superior compared to the rhodium catalyst in terms of regioselectivity. The rhodium-catalyzed cycloaddition of 143 afforded an undesirable regioisomer as the major product along with 144, whereas 1 exclusively furnished the desired regioisomer 144 in 92% yield (Scheme 34b). Similarly, cycloadducts 142 and 146 were obtained as the exclusive regioisomeric products in greater than 90% yields (Schemes 34a and 34c). The intermediates 142, 144, and 146 were ultimately transformed into the target natural products, i.e., perlolyrine, lavendamycin methyl ester, and eudistomin U, respectively.
The copper- and ruthenium-catalyzed [3 + 2] alkyne-azide cycloadditions have found numerous applications such as the synthesis of bioactive molecules, advanced functional materials, and supramolecular structures.47,64-75 Selected examples of the functional molecules synthesized using RuAAC are shown in Figure 5.
Two biologically significant molecules can be covalently attached using [3 + 2] cycloadditions, and several conjugate molecules with a triazole tether have been synthesized.64,70,73,74 1,2,3-Triazole rings are also considered as the bioisosteres of peptide bonds, esters, and olefins. In particular, 1,5-disubstituted 1,2,3-triazoles have been employed as stable cis-peptide bond surrogates.66,68,71 Moreover, a 1,5-disubstituted 1,2,3-triazole has been successfully introduced as the surrogate of a disulfide bridge in a peptide.72 RuAAC has also been used for the late-stage modification of reactive TM complexes.73 The hybridization of a thiourea catalyst with a chiral pyrrolidine catalyst was accomplished using alkyne-azide couplings.74 Among the prepared organocatalysts, those containing a 1,5-disubstituted triazole tether exhibited better yields and enantioselectivity compared to a 1,4-disubstituted triazole tether in an asymmetric Michael addition reaction. Hyperbranched polytriazoles have also been synthesized using the RuAAC polymerization.75
SUMMARY
Ruthenium catalysis has been extensively explored during the past decades. The newly developed ruthenium-catalyzed alkyne cycloadditions have significantly expanded the scope of heterocycle synthesis, e.g., the ruthenium-catalyzed [2 + 2 + 2] cycloadditions of α,ω-diynes with nitriles and heterocumulenes furnished bicyclic six-membered nitrogen or sulfur heterocycles with unprecedented efficiency and selectivity. The synthetic potential of these methods has also been demonstrated by the synthesis of natural products and biologically active molecules. Besides these [2 + 2 + 2] cycloadditions, the ruthenium-catalyzed [4 + 2] and [3 + 3] cycloadditions involving ketone derivatives provide important synthetic routes to six-membered oxygen heterocycles, such as 4H-pyran derivatives. Although it has not been much explored, the ruthenium-catalyzed [2 + 2 + 1] cycloaddition offer a powerful methodology for the synthesis of diverse five-membered heterocycles. Furthermore, the advances in the ruthenium-catalyzed [3 + 2] cycloaddition of alkynes with azides to afford 1,5-disubstituted 1,2,3-triazoles significantly progressed the application to chemical biology studies because of the diverse applicability of 1,2,3-triazole motif as an innocent tether connecting biologically active segments, bioisosteres of amides, esters, and olefins, and as the surrogates of cis-peptide bonds and disulfide bridges. In the future, further developments in the ruthenium catalysis will become significantly important in diverse fields such as natural product chemistry, chemical biology, pharmaceutical, and material sciences.
ACKNOWLEDGEMENTS
This author express his sincere thanks to Prof. Emeritus Kenji Itoh and past coworkers.
References
1. J. A. Joule and K. Mills, 'Heterocyclic Chemistry,' 4th ed., Blackwell Publishing, Oxford, 2000.
2. For selected reviews on the transition-metal-catalyzed synthesis of heterocycles, see: I. Nakamura and Y. Yamamoto, Chem. Rev., 2004, 104, 2127; CrossRef D. M. D’Souza and T. J. J. Müller, Chem. Soc. Rev., 2007, 36, 1095; CrossRef N. T. Patil and Y. Yamamoto, Chem. Rev., 2008, 108, 3395; CrossRef K. C. Majumdar, P. Debnath, and B. Roy, Heterocycles, 2009, 78, 2661; CrossRef K. C. Majumdar, B. Chattopadhyay, P. K. Maji, S. K. Chattopadhyay, and S. Samanta, Heterocycles, 2010, 81, 795; CrossRef T. Liu and H. Fu, Synthesis, 2012, 44, 2805. CrossRef
3. For selected reviews on the ruthenium catalysis, see: T. Naota, H. Takaya, and S.-I. Murahashi, Chem. Rev., 1998, 98, 2599; CrossRef B. M. Trost, F. D. Toste, and A. B. Pinkerton, Chem. Rev., 2001, 101, 2067; CrossRef B. M. Trost, M. U. Frederiksen, and M. T. Rudd, Angew. Chem. Int. Ed., 2005, 44, 6630; CrossRef C. S. Yi, J. Organomet. Chem., 2011, 696, 76; CrossRef 'Ruthenium in Organic Synthesis,' ed. by S.-I. Murahashi, Wiley-VCH, Weinheim, 2004; CrossRef 'Ruthenium Catalysts and Fine Chemistry,' Topics in Organometallic Chemistry 11, ed. by C. Bruneau and P. H. Dixneuf, Springer, Berlin, 2004. CrossRef
4. For selected reviews on the heterocycles synthesis by metathesis reactions, see: A. Deiters and S. F. Martin, Chem. Rev., 2004, 104, 2199; CrossRef M. D. McReynolds, J. M. Dougherty, and P. R. Hanson, Chem. Rev., 2004, 104, 2239. CrossRef
5. Y. Yamamoto and K. Itoh, 'Ruthenium-Catalysts and Fine Chemistry,' Topics in Organometallic Chemistry 11, ed. by C. Bruneau and P. H. Dixneuf, Springer, Berlin, 2004, pp. 249-276. CrossRef
6. For selected reviews on the heterocycles synthesis via transition-metal-catalyzed alkyne transformations, see: S. Cacchi, J. Organomet. Chem., 1999, 576, 42; CrossRef S. F. Kirsch, Synthesis, 2008, 3183. CrossRef
7. M. Jiménez-Tenorio, M. C. Puerta, and P. Valerga, Eur. J. Inorg. Chem., 2004, 17; CrossRef Y. Yamamoto, K. Yamashita and Y. Harada, Chem. Asian J., 2010, 5, 946; CrossRef Y. Yamamoto, 'Ruthenium-Mediated [2 + 2 + 2] Cycloaddition,' Transition-Metal-Mediated Aromatic Ring Construction, ed. by K. Tanaka, Wiley, Weinheim, 2013, pp. 71-126.
8. Y. Wakatsuki and H. Yamazaki, J. Chem. Soc., Chem. Commun., 1973, 280. CrossRef
9. For selected reviews on [2 + 2 + 2] cycloadditions leading to heterocycles, see: J. A. Varela and C. Saá, Chem. Rev., 2003, 103, 3787; CrossRef B. Heller and M. Hapke, Chem. Soc. Rev., 2007, 36, 1085; CrossRef Y. Yamamoto, Chim. Oggi, 2007, 25, 108; J. A. Varela and C. Saá, Synlett, 2008, 2571; CrossRef M. R. Shaaban, R. El-Sayed, and A. H. M. Elwahy, Tetrahedron, 2011, 67, 6095; CrossRef K. Tanaka, Heterocycles, 2012, 85, 1017. CrossRef
10. Y. Wakatsuki and H. Yamazaki, Tetrahedron Lett., 1973, 3383; CrossRef Y. Wakatsuki and H. Yamazaki, Synthesis, 1976, 26; CrossRef Bönnemann, R. Brinkmann, and H. Schenkluhn, Synthesis, 1974, 575; CrossRef H. Bönnemann, Angew. Chem., Int. Ed. Engl., 1978, 17, 505; CrossRef H. Bonnemann, Angew. Chem., Int. Ed. Engl., 1985, 24, 248. CrossRef
11. A. Naiman and K. P. C. Vollhardt, Angew. Chem., Int. Ed. Engl., 1977, 16, 708; CrossRef D. J. Brien, A. Naiman, and K. P. C. Vollhardt, J. Chem. Soc., Chem. Commun., 1982, 133. CrossRef
12. C. Y. Ren, W. C. Cheng, W. C. Chan, C. H. Yeung, and C. P. Lau, J. Mol. Cat., 1990, 59, L1; CrossRef P. Pertici, A. Verrazzani, G. Vitulli, R. Baldwin, and M. A. Bennett, J. Organomet. Chem., 1998, 551, 37. CrossRef
13. Y. Yamamoto, S. Okuda, and K. Itoh, Chem. Commun., 2001, 1102. CrossRef
14. Y. Yamamoto, K. Kinpara, T. Saigoku, H. Takagishi, S. Okuda, H. Nishiyama, and K. Itoh, J. Am. Chem. Soc., 2005, 127, 605. CrossRef
15. Y. Yamamoto, K. Kinpara, T. Saigoku, H. Nishiyama, and K. Itoh, Org. Biomol. Chem., 2004, 2, 1287. CrossRef
16. Y. Yamamoto, R. Ogawa, and K. Itoh, J. Am. Chem. Soc., 2001, 123, 6189. CrossRef
17. Y. Yamamoto, K. Kinpara, H. Nishiyama, and K. Itoh, Adv. Synth. Catal., 2005, 347, 1913. CrossRef
18. Y. Yamamoto, K. Kinpara, R. Ogawa, H. Nishiyama, and K. Itoh, Chem. Eur. J., 2006, 12, 5618. CrossRef
19. G. Dazinger, M. Torres-Rodrigues, K. Kirchner, M. J. Calhorda, and P. J. Costa, J. Organomet. Chem., 2006, 691, 4434. CrossRef
20. J. A. Varela, L. Castedo, and C. Saá, J. Org. Chem., 2003, 68, 8595. CrossRef
21. L. Severa, J. Vávra, A. Kohoutová, M. Čížková, T. Šálová, J. Hývl, D. Šaman, R. Pohl, L. Adriaenssens, and F. Teplý, Tetrahedron Lett., 2009, 50, 4526. CrossRef
22. L. Adriaenssens, L. Severa, J. Vávra, T. Šálová, J. Hývl, M. Čížková, R. Pohl, D. Šaman, and F. Teplý, Collect. Czech. Chem. Commun., 2009, 74, 1023. CrossRef
23. F. Xu, C. Wang, X. Li, and B. Wan, ChemSusChem, 2012, 5, 854. CrossRef
24. S. Medina, G. Domínguez, and J. Pérez-Castells, Org. Lett., 2012, 14, 4982. CrossRef
25. P. Hong and H. Yamazaki, Tetrahedron Lett., 1977, 1333; CrossRef P. Hong and H. Yamazaki, Synthesis, 1977, 50; CrossRef H. Hoberg and B. W. Oster, Synthesis, 1982, 324; CrossRef H. Hoberg and B. W. Oster, J. Organomet. Chem., 1982, 234, C35; CrossRef H. Hoberg and B. W. Oster, J. Organomet. Chem., 1983, 252, 359. CrossRef
26. R. A. Earl and K. P. C. Vollhardt, J. Am. Chem. Soc., 1983, 105, 6991; CrossRef R. A. Earl and K. P. C. Vollhardt, J. Org. Chem., 1984, 49, 4786. CrossRef
27. Y. Yamamoto, H. Takagishi, and K. Itoh, Org. Lett., 2001, 3, 2117. CrossRef
28. Y. Yamamoto, H. Takagishi, and K. Itoh, J. Am. Chem. Soc., 2002, 124, 28. CrossRef
29. R. M. Beesley, C. K. Ingold, and J. F. Thorpe, J. Chem. Soc., 1915, 107, 1080; CrossRef M. E. Jung and G. Piizzi, Chem. Rev., 2005, 105, 1735. CrossRef
30. R. Schmid and K. Kirchner, J. Org. Chem., 2003, 68, 8339; CrossRef G. Dazinger, R. Schmid, and K. Kirchner, New J. Chem., 2004, 28, 153. CrossRef
31. S. Alvarez, S. Medina, G. Domínguez, and J. Pérez-Castells, J. Org. Chem., 2013, 78, 9995. CrossRef
32. D. F. Harvey, B. M. Johnson, C. S. Ung, and K. P. C. Vollhardt, Synlett, 1989, 15; CrossRef R. Gleiter and V. Schehlmann, Tetrahedron Lett., 1989, 30, 2893. CrossRef
33. T. Takahashi, Y. Li, T. Ito, F. Xu, K. Nakajima, and Y. Liu, J. Am. Chem. Soc., 2002, 124, 1144. CrossRef
34. T. Tsuda, T. Kiyoi, T. Miyane, and T. Saegusa, J. Am. Chem. Soc., 1988, 110, 8570. CrossRef
35. K. Tanaka, Y. Otake, A. Wada, K. Noguchi, and M. Hirano, Org. Lett., 2007, 9, 2203; CrossRef K. Tanaka, R. Tanaka, G. Nishida, and M. Hirano, Synlett, 2008, 2017; CrossRef T. Suda, K. Noguchi, and K. Tanaka, Angew. Chem. Int. Ed., 2011, 50, 4475; CrossRef Y. Miyauchi, M. Kobayashi, and K. Tanaka, Angew. Chem. Int. Ed., 2011, 50, 1092. CrossRef
36. Y. Yamamoto, H. Takagishi, and K. Itoh, J. Am. Chem. Soc., 2002, 124, 6844. CrossRef
37. J. Rodríguez-Otero, M. M. Montero-Campillo, and E. M. Cabaleiro-Lago, J. Phys. Chem. A, 2008, 112, 8116. CrossRef
38. B. M. Trost, R. E. Brown, and F. D. Toste, J. Am. Chem. Soc., 2000, 122, 5877. CrossRef
39. Y. Nishibayashi, M. Yoshikawa, Y. Inada, M. Hidai, and S. Uemura, J. Org. Chem., 2004, 69, 3408. CrossRef
40. M. Zhang, H.-F. jiang, H. Neumann, M. Beller, and P. H. Dixneuf, Angew. Chem. Int. Ed., 2009, 48, 1681. CrossRef
41. E. Müller, Synthesis, 1974, 761. CrossRef
42. Y. Wen, S. Zhu, H. Jiang, A. Wang, and Z. Chen, Synlett, 2011, 1023. CrossRef
43. K. Yamashita, Y. Yamamoto, and H. Nishiyama, J. Am. Chem. Soc., 2012, 134, 7660. CrossRef
44. X. Chen, J. Jin, Y. Wang, and P. Liu, Chem. Eur. J., 2011, 17, 9920. CrossRef
45. For selected reviews, see: R. Huisgen, Angew. Chem., Int. Ed. Engl., 1963, 2, 565; CrossRef R. Huisgen, Angew. Chem., Int. Ed. Engl., 1963, 2, 633; CrossRef S. T. Abu-Orabi, Molecules, 2002, 7, 302; CrossRef A. R. Katritzky, Y. Zhang, and S. K. Singh, Heterocycles, 2003, 60, 1225; CrossRef K. C. Majumdar and K. Ray, Synthesis, 2011, 3767. CrossRef
46. C. W. Tornøe, C. Christensen, and M. Meldal, J. Org. Chem., 2002, 67, 3057; CrossRef V. V. Rostovtsev, L. G. Green, V. V. Fokin, and K. B. Sharpless, Angew. Chem. Int. Ed., 2002, 41, 2596; CrossRef J. E. Hein and V. V. Fokin, Chem. Soc. Rev., 2010, 39, 1302. CrossRef
47. For recent reviews, see: S. K. Mamidyala and M. G. Finn, Chem. Soc. Rev., 2010, 39, 1252; CrossRef A. Qin, J. W. Y. Lam, and B. Z. Tang, Chem. Soc. Rev., 2010, 39, 2522; CrossRef V. Aragão-Leoneti, V. L. Campo, A. S. Gomes, R. A. Field, and I. Carvalho, Tetrahedron, 2010, 66, 9475; CrossRef X. Li, Chem. Asian J., 2011, 6, 2606; CrossRef A. C. Fahrenbach and J. F. Stoddart, Chem. Asian J., 2011, 6, 2660; CrossRef S. G. Agalave, S. R. Maujan, and V. S. Pore, Chem. Asian J., 2011, 6, 2696; CrossRef D. S. Pedersen and A. Abell, Eur. J. Org. Chem., 2011, 2399; CrossRef L. Liang and D. Astruc, Coord. Chem. Rev., 2011, 255, 2933. CrossRef
48. L. Zhang, X. Chen, P. Xue, H. H. Y. Sun, I. D. Williams, K. B. Sharpless, V. V. Fokin, and G. Jia, J. Am. Chem. Soc., 2005, 127, 15998. CrossRef
49. B. C. Boren, S. Narayan, L. K. Rasmussen, L. Zhang, H. Zhao, Z. Lin, G. Jia, and V. V. Fokin, J. Am. Chem. Soc., 2008, 130, 8923. CrossRef
50. S. Oppilliart, G. Mousseau, L. Zhang, G. Jia, P. Thueery, B. Rousseau, and J.-C. Cintrat, Tetrahedron, 2007, 63, 8094. CrossRef
51. C.-T. Zhang, X. Zhang, and F.-L. Qing, Tetrahedron Lett., 2008, 49, 3927.. CrossRef
52. M. M. Majireck and S. M. Weinreb, J. Org. Chem., 2006, 71, 8680; CrossRef Also, see: A. H. Yap and S. M. Weinreb, Tetrahedron Lett., 2006, 47, 3035. CrossRef
53. D.-R. Hou, T.-C. Kuan, Y.-K. Li, R. Lee, and K.-W. Huang, Tetrahedron, 2010, 66, 9415. CrossRef
54. M. Lamberti, G. C. fortman, A. Poater, J. Broggi, A. M. Z. Slawin, L. Cavallo, and S. P. Nolan, Organometallics, 2012, 31, 756. CrossRef
55. E. Boz and N. Ş. Tüzün, J. Organomet. Chem., 2013, 724, 167. CrossRef
56. L. K. Rasmussen, B. C. Boren, and V. V. Fokin, Org. Lett., 2007, 9, 5337. CrossRef
57. J. R. Johansson, P. Lincoln, B. Nordén, and N. Kann, J. Org. Chem., 2011, 76, 2355. CrossRef
58. A. R. Kelly, J. Wei, S. Kesavan, J.-C. Marié, N. Windmon, D. W. Young, and L. A. Marcaurelle, Org. Lett., 2009, 11, 2257. CrossRef
59. P. N. Liu, J. Li, F. H. Su, K. D. Ju, L. Zhang, C. Shi, H. H. Y. Sung, I. D. Williams, V. V. Fokin, Z. Lin, and G. Jia, Organometallics, 2012, 31, 4904. CrossRef
60. D. Wang, L. Salmon, J. Ruiz, and D. Astruc, Chem. Commun., 2013, 49, 6956. CrossRef
61. Y. Yamamoto, K. Yamashita, T. Hotta, T. Hashimoto, M. Kikuchi, and H. Nishiyama, Chem. Asian J., 2007, 2, 1388. CrossRef
62. Y. Zou, Q. Liu, and A. Deiters, Org. Lett., 2011, 13, 4352. CrossRef
63. B. Dassonneville, B. Witulski, and H. Detert, Eur. J. Org. Chem., 2011, 2836; CrossRef F. Nissen and H. Detert, Eur. J. Org. Chem., 2011, 2845; CrossRef F. Nissen, V. Richard, C. Alayrac, and B. Witulski, Chem. Commun., 2011, 47, 6656. CrossRef
64. A. J. Salmon, M. L. Williams, A. Innocenti, D. Vullo, C. T. Supuran, and S.-A. Poulsen, Bioorg. Med. Chem. Lett., 2007, 17, 5032; CrossRef Also, see: A. J. Salmon, M. L. Williams, A. Maresca, C. T. Supuran, and S.-A. Poulsen, Bioorg. Med. Chem. Lett., 2011, 21, 6058. CrossRef
65. G. Appendino, S. Bacchiega, A. Minassi, M. G. Cascio, L. De Petrocellis, and V. Di Marzo, Angew. Chem. Int. Ed., 2007, 46, 9312. CrossRef
66. A. Tam, U. Arnold, M. B. Soellner, and R. T. Raines, J. Am. Chem. Soc., 2007, 129, 12670. CrossRef
67. D. Imperio, T. Pirali, U. Galli, F. Pagliai, L. Cafici, P. L. Canonico, G. Sorba, A. A. Genazzani, and G. C. Tron, Bioorg. Med. Chem., 2007, 15, 6748. CrossRef
68. W. S. Horne, C. A. Olsen, J. M. Beierle, A. Montero, and M. R. Ghadiri, Angew. Chem. Int. Ed., 2009, 48, 4718. CrossRef
69. M. Chemama, M. Fonvielle, M. Arthur, J.-M. Valéry, and M. Etheve-Quelquejeu, Chem. Eur. J., 2009, 15, 1929. CrossRef
70. R. Moumné, V. Larue, B. Seijo, T. Lecourt, L. Micouin, and C. Tisné, Org. Biomol. Chem., 2010, 8, 1154. CrossRef
71. D. Tietze, M. Tischler, S. Voigt, D. Imhof, O. Ohlenschläger, M. Görlach, and G. Buntkowsky, Chem. Eur. J., 2010, 16, 7572. CrossRef
72. M. Empting, O. Avrutina, R. Meusinger, S. Fabritz, M. Reinwarth, M. Biesalski, S. Voigt, G. Buntkowsky, and H. Kolmar, Angew. Chem. Int. Ed., 2011, 50, 5207. CrossRef
73. E. Chardon, G. L. Puleo, G. Dahm, G. Guichard, and S. Bellemin-Laponnaz, Chem. Commun., 2011, 47, 5864. CrossRef
74. K. Takasu, T. Azuma, and Y. Takemoto, Tetrahedron Lett., 2010, 51, 2737. CrossRef
75. A. Qin, J. W. Y. Lam, C. K. W. Jim, L. Zhang, J. Yan, M. Häussler, J. Liu, Y. Dong, D. Liang, E. Chen, G. Jia, and B. Z. Tang, Macromolecules, 2008, 41, 3808. CrossRef