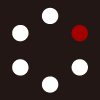
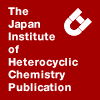
HETEROCYCLES
An International Journal for Reviews and Communications in Heterocyclic ChemistryWeb Edition ISSN: 1881-0942
Published online by The Japan Institute of Heterocyclic Chemistry
e-Journal
Full Text HTML
Received, 30th October, 2013, Accepted, 14th February, 2014, Published online, 21st February, 2014.
DOI: 10.3987/REV-13-786
■ Transition Metal-Catalyzed Synthesis of π-Conjugated Cyclic Esters and Amides from Alkynes and Carbonyl Reagents
Tetsuaki Fujihara and Yasushi Tsuji*
Deparment of Energy and Hydrocarbon Chemistry, Graduate School of Engineering, Kyoto University, Kyoudai-katsura, Nishikyo, Kyoto 615-8510, Japan
Abstract
The transition metal-catalyzed intermolecular reaction of carbonyl precursors with alkynes is a simple method for constructing various carbonyl compounds. We review the syntheses of π-conjugated cyclic carbonyl compounds from the reaction of alkynes with suitable carbonyl precursors in the presence of transition metal catalysts. These methods afford isocoumarins, chromones, 2-quinolones, 4-quinolones, and isoquinolones, and the reaction of diynes with carbon dioxide produces pyrones.CONTENTS
1. Introduction
2. π-Conjugated cyclic esters
2-1. Synthesis of isocoumarins
2-2 Synthesis of chromones
2-3 Synthesis of pyrones from carbon dioxide
3. π-Conjugated cyclic amides
3-1. Synthesis of 2-quinolones
3-2. Synthesis of 4-quinolones
3-3. Synthesis of isoquinolones
4. Conclusion
1. INTRODUCTION
The synthesis and functionalization of carbonyl-containing heterocycles have been extensively studied because they are common in biologically active molecules.1 Among them, π-conjugated cyclic esters such as isocoumarins can be synthesized by the reaction of 2-halophenols with an alkyne and carbon monoxide in the presence of a palladium catalyst.2 When 2-haloaniline derivatives instead of 2-halophenols are used, π-conjugated cyclic amides such as quinolones can be obtained.2 Although these methods afford the desired products in good to high yields, the use of highly toxic carbon monoxide is a disadvantage. Thus, transformations avoiding carbon monoxide gas are highly desirable.3 Meanwhile, the transition metal-catalyzed transformations of carbonyl reagents with carbon-carbon multiple bonds, such as alkynes and alkenes, are particularly important.4 The addition of aldehydes (hydroacylation),5 formamides (hydrocarbamoylation),6 formates (hydroesterification),7 and acid chlorides (chloroacylation)8 has been reported to date (Scheme 1a). These transformations are attractive because all the atoms in the substrates are retained in the product.9 An important step of the transformation is the activation of the carbonyl reagents with transition metal complexes via oxidative addition, to afford an intermediate with a metal-carbon(CO) bond (A), as shown in Scheme 1a. Cyclic carbonyl compounds can be obtained by activating a suitable carbonyl reagent, to afford a metallacycle intermediate containing a metal-carbon(CO) bond (B) followed by the insertion of carbon-carbon unsaturated compounds (Scheme 1b).
This review focuses on the transition metal-catalyzed synthesis of π-conjugated cyclic esters and amides from alkynes and carbonyl reagents. The elimination of a gaseous molecule such as carbon monoxide, carbon dioxide (CO2), or molecular nitrogen, from a carbonyl reagent is one of the important processes to afford the metallacycle species, which is an indispensable intermediate in the transformation. The activation of the C-H bond also gave the metallacycle intermediate. Isocoumarins, chromones, 2-quinolones, 4-quinolones, and isoquinolones can be obtained by these methods. The substrate scope and reaction mechanism of these reactions are discussed.
2. π-CONJUGATED CYCLIC ESTERS
2-1. Synthesis of isocoumarins
Sato and Miura found that rhodium complexes catalyzed the reaction of benzoic acids with alkynes via C-H bond activation, to afford isocoumarin derivatives (3) (Scheme 2).10 The reaction of alkynes (1) and benzoic acids (2) in the presence of a catalytic amount of [RhCl2(Cp*)]2 (Cp* = cyclopentadienyl) and Cu(OAc)2·H2O under air atmosphere afforded 3 in a high yield. The electron-withdrawing and electron-donating substituents on the aromatic rings of the carboxylic acids did not affect the yield. Internal alkynes, such as 4-octyne, participated in the reaction, to afford the corresponding products in high yields. The reactions of unsymmetrical internal alkynes such as 1-phenyl-1-octyne proceeded in a regioselective manner.
A plausible catalytic cycle is shown in Scheme 3. First, the coordination of the carboxylate oxygen atom to rhodium(III) gives rhodium benzoate A. Subsequent ortho-rhodation gives a rhodacycle intermediate B. The insertion of the alkyne to the rhodium-carbon bond followed by the reductive elimination affords 3. The rhodium(I) species is oxidized in the presence of the copper co-catalyst under air atmosphere to generate the rhodium(III) species, thus completing the catalytic cycle.
Kurahashi and Matsubara reported that the nickel-catalyzed decarbonylative addition of phthalic anhydride (4) to alkynes (1) gave 3 (Scheme 4).11 The reaction of phthalic anhydride and 4-octyne with the Ni-PMe3 (PMe3: trimethylphosphine) system in acetonitrile at 80 °C afforded the corresponding product in only 12% yield. The addition of ZnCl2 dramatically improved the yield to 96%. The diphenylacetylene produced a yield of 87%. Although a mixture of regioisomers was obtained for the unsymmetrical internal alkynes such as 2-octyne, the reaction of 1-trimethysilyl-1-propyne afforded a single regioisomer. Substituted phthalic anhydride derivatives also gave the corresponding products in good yields.
A plausible catalytic cycle is shown in Scheme 5. First, the oxidative addition of the O-CO bond of anhydride 4 to the Ni(0) complex with electron-rich phosphine ligands affords a nickelacycle intermediate A. Next, the intermediate is decarbonylated, to give B. Then, an alkyne is inserted into the nickel-carbon bond to afford a nickelacycle D. The reductive elimination of D gives 3 and regenerates the starting Ni(0) complex, thus completing the catalytic cycle. The coordination of ZnCl2 to the carbonyl group on intermediate D may have assisted the reductive elimination.
2.2. Synthesis of coumarins
A method for synthesizing coumarin derivatives (6) from o-arylcarbonyloxybenzonitriles (5) and alkynes (1) via the elimination of benzonitriles has been developed (Scheme 6).12 The reaction of o-benzoyloxybenzonitrile with 4-octyne in the presence of Ni(cod)2 (cod = cyclooctadiene) and P(CH2Ph)3 with methylaluminum bis(2,6-di-tert-butyl-4-methyphenoxide) (MAD)13 as the co-catalyst, in toluene at 120 °C, afforded the desired product in 65% yield. In the absence of MAD, the reaction did not proceed. The benzonitriles containing o-arylcarbonyloxy moiety with electron-donating substituents such as dimethylamino, methoxy, and methyl groups, gave the corresponding products in high yields. However, the reaction of o-acetoxybenzonitrile failed.
Although the reaction mechanism was not clear, a control reaction was carried out (eq. 1). When the product formed by the reaction of 5 with an alkyne via C-CN bond activation was treated with the nickel catalyst, 6 and the corresponding benzonitrile derivative were not obtained, indicating that the cycloaddition proceeded via the initial elimination of the benzonitrile derivative followed by the reaction with the alkyne.
2.3. Synthesis of chromones
Kurahashi and Matsubara reported a unique method for the synthesis of chromone derivatives (8) from acid ketals (7) and alkynes (1) via the elimination of ketones (Scheme 7).14 The reaction of salicylic acid acetal with 4-octyne catalyzed by Ni(cod)2 and tricyclohexylphosphine (PCy3) in the presence of pyridine (1 equiv) afforded the corresponding product in 99% yield. The reaction did not proceed in the absence of pyridine, and other additives such as DMAP and DABCO were not effective. The reaction of various internal alkynes afforded the corresponding products in good to high yields.
A plausible catalytic cycle is shown in Scheme 8. The oxidative addition of the O-CO bond of acid ketal 7 to Ni(0) complex with electron-rich phosphine ligands affords nickelacycle intermediate A. The subsequent elimination of benzophenone gives an intermediate B. Next, the alkyne is inserted into the nickel-carbon bond to afford a nickelacycle D. The reductive elimination of D gives 8 and regenerates the starting Ni(0) complex, thus completing the catalytic cycle
2.4 Synthesis of pyrones from carbon dioxide
Carbon dioxide (CO2) is a readily available, nontoxic, and renewable carbon source.15 The use of this environment-friendly raw material in the carbon-carbon bond-forming reactions is an important challenge in homogeneous transition metal catalysis.16 Useful transformations of CO2, such as the hydrocarboxylation of carbon-carbon multiple bonds and the carboxylation of organozinc or boron compounds and aryl halides, have been reported. Furthermore, the cycloaddition via metallacycles using CO2 and carbon-carbon multiple bonds is also a promising approach.
Tsuda and Saegusa reported that the Ni(0) complexes bearing bulky and basic phosphine ligands catalyzed the [2 + 2 + 2] cycloaddition of a diyne with CO2 to afford the corresponding pyrone derivatives.17 The reaction of the diyne in tetrahydrofuran (THF) in the presence of a nickel catalyst with PCy3 ligands under a high pressure of CO2 (50 kg/cm2) gave the corresponding pyrone (eq. 2).
Recently, a nickel catalyst bearing an N-heterocyclic carbene ligand was found to be effective for the synthesis of pyrons from diynes (9) and CO2 (Scheme 9).18 The reaction proceeded under atmospheric pressure of CO2 with diverse diynes as the substrates. Furthermore, the synthesis of pyrones from two equiv of alkyne and CO2 was also reported. However, the reaction required a high CO2 pressure (20-120 atm).19
A plausible catalytic cycle is shown in Scheme 10. First, the oxidative cyclization of one of the carbon-carbon triple bonds in the diyne (9) with CO2 catalyzed by a Ni(0) complex affords a nickelacycle intermediate A. The subsequent insertion of the second alkyne moiety into the nickel-carbon bond gives a seven-membered nickelacycle B. The reductive elimination of B affords the pyrone (10) and regenerates the starting Ni(0) complex.
Recently, we reported the copper complex-catalyzed silacarboxylation of alkynes. The reaction of internal alkynes with a silylborane, as a silicon source, in the presence of a copper catalyst under CO2 atmosphere afforded the corresponding silalactones (11) in good to high yields (eq. 3).20
3. π-CONJUGATED CYCLIC AMIDES
3.1. Synthesis of 2-quinolones
We have developed the efficient annulation of N-arylcarbamoyl chlorides (12) with internal alkynes (1) in the presence of an iridium catalyst to give 2-quinolones (13) (Scheme 11).21 The reaction of N-methyl-N-phenylcarbamoyl chloride with 5-decyne in the presence of a catalytic amount (2.5 mol%) of [IrCl(cod)]2 and cod (30 mol%) in refluxing o-xylene afforded 3,4-dibutyl-1-methyl-2-quinolone in 92% yield. Various aliphatic and aromatic internal alkynes were converted to the corresponding 2-quinolones. The reaction of diarylalkynes with N-(3-methoxyphenyl)-N-methylcarbamoyl chloride gave the corresponding product in high yields as the single regioisomers. Unsymmetrical alkynes afforded the corresponding products in high yields, albeit with low regioselectivity. The use of unsymmetrical alkynes bearing an ether group improved the regioselectivity of the products, possibly because of the directing effect of the oxygen atom.
The substrates with electron-rich and electron-poor phenyl moieties on the nitrogen participated in the cyclization to afford the corresponding products in good to high yields (Scheme 12). The reactions with a carbamoyl chloride bearing a meta-substituted aryl ring produced a single isomer. The substrate with 4-methoxyphenylmethyl substituent on the nitrogen atom afforded the corresponding 2-quinolones in good to excellent yields. The 4-methoxyphenylmethyl group was removed by treating trifluoroacetic acid to afford 3,4-dibutyl-2-quinolone in 91% yield. The condensed ring systems could be constructed. The reaction was effective used for N-aryl and N-alkenyl carbamoyl chlorides as the substrate.
To gain further insight into the catalytic reaction mechanisms, the stoichiometric reaction of [IrCl(cod)]2 with N-methyl-N-phenylcarbamoyl chloride was carried out in the presence of additional amount of cod. The reaction of N-methyl-N-phenylcarbamoyl chloride was completed in refluxing toluene after 12 h. Then, the addition of 5-decyne to the reaction mixture under reflux afforded the corresponding product in 64% yield (Scheme 13). When PPh3 (P/Ir = 2/1) was added to the reaction mixture instead of 5-decyne, an iridium(III) metallacycle complex was isolated in 69% yield and its structure was confirmed by X-ray crystallographic analysis.
A plausible catalytic cycle is shown in Scheme 14. The oxidative addition of carbamoyl chloride 12 to iridium(I) affords a carbamoyl-chloro-iridium(III) complex A. The intramolecular cyclization of A gives a five-membered iridacycle that may play a crucial role in suppressing the decarbonylation. The subsequent insertion of the alkyne (1) followed by the reductive elimination affords 2-quinolone 13 and regenerates the iridium(I) species, thus completing the catalytic cycle.
Kurahashi, Matsubara, and co-workers reported the nickel-catalyzed synthesis of 2-quinolones from o-cyanobenzamides (14) and alkynes via elimination of benzonitrile (Scheme 15).22 The reaction of o-cyanobenzamide with 4-octyne in the presence of MAD, as the co-catalyst, and Ni(cod)2 and PMe3, as the catalysts, in toluene at 120 °C afforded the desired product in 80% yield. When P(CH2Ph)3, an efficient ligand for the related reaction as shown in Scheme 6,12 was used as the ligand, the yield of the product decreased to 36%. The amide substituents on the nitrogen atom affected the yield, and the phenyl group was the best substituent.
Scheme 16 shows a plausible catalytic cycle. First, the oxidative addition of the C-CN bond23 in 14 to the Ni(0) complex gives aryl nickel species A. The electrophilic ipso-attack of the leaving aryl group affords seven-membered intermediate B. The subsequent elimination of the benzonitrile and coordination of the alkyne affords nickelacycle C. Finally, the insertion of the alkyne gives a seven-membered nickelacycle cycle D, and the reductive elimination affords 13 and regenerates the starting Ni(0) complex.
3-2. Synthesis of 4-quinolones
It has been reported that the nickel-catalyzed decarboxylative addition of isatoic anhydrides (15) to alkynes (1) afforded 4-quinolones (16).24 The reaction of N-phenylisatoic anhydride with 4-octyne, Ni(cod)2 and PCy3 in toluene at 80 °C for 24 h gave the corresponding 4-quinolone in 97% yield. Various internal alkynes were converted to the corresponding 4-quinolones in good to high yields. The regioselectivity of the reaction can be explained by the direction of the alkyne insertion, where the repulsive steric interaction is minimal between the bulkier substituents on the alkyne and ligand. The reaction with 4-methyl-2-pentyne afforded a 6:1 mixture of regioisomers when PCy3 was used as the ligands. In contrast, a less bulky ligand, PMe3, reduced the regioselectivity of the reaction and resulted in a 3:2 mixture of regioisomers.
A plausible catalytic cycle is shown in Scheme 18. First, the oxidative addition of the C-CO bond of the isatoic anhydride (15) to the Ni(0) complex affords a seven-membered nickelacycle intermediate A. The subsequent decarboxylation of A, and coordination of alkyne affords an intermediate C. The insertion of the alkyne into the nickel-carbon bond gives nickelacycle D. The reductive elimination affords 16 and regenerates the starting Ni(0) complex, thus completing the catalytic cycle.
3.3. Synthesis of isoquinolones
The nickel-catalyzed decarbonylative addition of phthalimides (17) to alkynes (1) to give isoquinolones (18) has been reported (Scheme 19).25 The reaction of N-(2-pyridyl)phthalimide with 4-octyne in the presence of Ni(cod)2 and PMe3 in toluene at 110 °C afforded the corresponding product in 84% isolated yield. Under the reaction conditions, N-phenylphthalimide was converted to the product only 18% yield, whereas an electron-withdrawing aromatic substituent on the phthalimide nitrogen atom favored the reaction. The reaction of aliphatic internal alkynes gave the corresponding products in high yields, even though the yield for diphenylacetylene was moderate.
A plausible catalytic cycle is shown in Scheme 20. First, the nucleophilic attack of the Ni(0) complex on the electron-rich phosphine ligands on the amide (17) gives a nickelacycle intermediate A. The subsequent decarbonylation affords a nickelacycle B. Next, the insertion of the alkyne into the nickel-carbon bond affords D. The reductive elimination of D gives 18 and regenerates the starting Ni(0) complex.
Murakami and co-workers reported the synthesis of isoquinolones by the nickel-catalyzed denitrogenative alkyne insertion into 1,2,3-benzotriazin-4-ones (19) (Scheme 21).26 The catalytic cycle starts with the insertion of the Ni(0) species into the N-N bond of 1,2,3-benzotriazin-4-ones followed by the elimination of molecular nitrogen to afford a nickelacycle B (Scheme 19). The stoichiometric reaction of a 1,2,3-benzotriazin-4-one with Ni(cod)2 and diphenylphosphinobenzene in THF at room temperature afforded an azanickelacycle species in 79% yield, and the molecular structure of the complex was determined by X-ray crystallographic analysis (eq. 4).27 The enantioselective synthesis of 3,4-dihydroisoquinolone from 1,2,3-benzotriazin-4-ones and allenes using nickel catalysts with chiral phosphine ligands has also been reported.26
Guimond and co-workers reported the rhodium-catalyzed synthesis of isoquinolones (18) from N-substituted hydroxamic acids and alkynes via C-H bond activation (Scheme 22).28 The reaction of benzohydroxamic acid with diphenylacetylene in the presence of [RhCl2(Cp*)]2 and two equiv of Cu(OAc)·2H2O, as the oxidant, in DMF at 60 °C afforded a mixture of isoquinolones (eq. 5). Interestingly, in the absence of the oxidant, the reaction proceeded to afford 18 in a high yield. The reaction of diverse alkynes gave the corresponding products in good to high yields.
Recently, Guimond and co-workers reported that substituents on the nitrogen atom affected the reactivity.29 The pivaloyl group was the best substituent for the rhodium-catalyzed synthesis of isoquinolones. The reaction proceeded with a low catalyst loading at room temperature in the presence of 2 equiv of CsOAc (Scheme 23). Importantly, terminal alkynes participated in the reaction, and afforded the corresponding products in good to high yields and high regioselectivity.
A plausible catalytic cycle is shown in Scheme 24. The reaction of the rhodium species with 21 gives rhodacycle intermediate B via the C-H bond cleavage. The alkyne is inserted into the Rh-C bond of the intermediate B to afford D. The concerted or stepwise C-N bond formation and N-O bond cleavage provided the product (18) and regenerates the rhodium catalyst.
Li and Wang also reported the ruthenium-catalyzed isoquinolone synthesis via the C-H activation in the absence of oxidant (eq. 6).30 The method was similar to Guimond’s system that used rhodium catalysts.
Rovis and co-workers reported the rhodium-catalyzed the oxidative cyclization of benzamide with alkynes via C-H/N-H bond activation (Scheme 25).31 The reaction of N-methylbenzamide with diphenylacetylene in the presence of [RhCl2(Cp*)]2 and Cu(OAc)2, as the oxidant, in tAmOH afforded the corresponding product in 82% yield. The reactions of electron-rich aromatic internal alkynes gave the corresponding products in high yields, whereas electron-withdrawing substituents on the aromatic ring resulted in lower yields. The reaction of 5-decyne afforded the corresponding product in a moderate yield. The substituents on the nitrogen atom affected the yield strongly; methyl substituent gave the product in high yield, even though the reaction of N-benzyl substituted benzamide resulted in a lower yield.
A plausible reaction mechanism is shown in Scheme 26. The reaction of the rhodium complex bearing acetate ligands with benzamide gives a five-membered rhodacycle intermediate (B) and acetic acid. The subsequent insertion of the alkyne into the rhodium-carbon bond affords a seven-membered intermediate (D). The reductive elimination of D affords 18 and the rhodium complex is regenerated, thus completing the catalytic cycle.
A similar reaction was also reported by Ackermann and co-workers using ruthenium catalysts in the presence of Cu(OAc)2 as the oxidant (eq. 7).32
Chatani and co-workers reported the nickel-catalyzed synthesis of isoquinolones from alkynes and benzamides bearing a 2-pyridylmethyl group on the nitrogen atom (Scheme 27).33 The reaction of the amide with 4-octyne in the presence of Ni(cod)2/PPh3 catalyst system in toluene at 130 °C for 18 h afforded the product in 28% yield. The yield increased to 86% on increasing the reaction temperature. The reaction of diphenylacetylene and aromatic internal alkynes bearing electron-withdrawing substituents gave the products in high yields, whereas those with electron-donating aromatic alkyne substrates resulted in lower yields. The benzamide substituents also affected the yield.
A plausible mechanism is displayed in Scheme 28. The coordination of the amide (23) to the nickel center as the N,N-donor followed by the activation of the N-H bond gives nickel hydride complex A. The insertion of the alkyne into the N-Ni bond of A affords vinylnickel intermediate B. The cleavage of the C-H bond and the concomitant formation of the alkene give an intermediate C. The insertion of the additional alkyne molecule into the nickel-carbon bond of C followed by the reductive elimination affords the isoquinolone (18) and regenerates the starting nickel catalyst.
4. CONCLUSION
This review summarizes the recent developments in the methods for synthesizing π-conjugated cyclic esters and amides from alkynes and carbonyl reagents. The key to the successful methods is the formation of the metallacycle in the reaction of carbonyl reagents with transition metal complexes. The elimination of gases such as carbon monoxide, carbon dioxide, and nitrogen is one of the important methods, to afford the metalacycle. In addition, the C-H bond activation also afforded the metallacycle intermediates. Isocoumarins, chromones, 2-quinolones, 4-quinolones, and isoquinolones, which are common motifs in biologically active compounds, could be obtained by employing these methods.
References
1. A. Katritzky, C. Ramsden, J. Joule, and V. Zhdankin, Handbook of Heterocyclic Chemistry, Elsevier, 2010; Modern Carbonylation Methods, ed. by L. Kollár, Wiley-VCH, Weinheim, 2008.
2. D. V. Kadnikov and R. C. Larock, J. Org. Chem., 2004, 69, 6772; CrossRef D. V. Kadnikov and R. C. Larock, J. Organomet. Chem., 2003, 687, 425. CrossRef
3. T. Morimoto and K. Kakiuchi, Angew. Chem. Int. Ed., 2004, 43, 5580; CrossRef T. Fujihara, T. Hosoki, Y. Katafuchi, T. Iwai, J. Terao, and Y. Tsuji, Chem. Commun., 2012, 48, 8012; CrossRef T. Ueda, H. Konishi, and K. Manabe, Org. Lett., 2012, 14, 3100; CrossRef T. Ueda, H. Konishi, and K. Manabe, Angew. Chem. Int. Ed., 2013, 52, 8611; CrossRef P. Hermange, A. T. Lindhardt, R. H. Taaning, K. Bjerglund, D. Lupp, and T. Skrydstrup, J. Am. Chem. Soc., 2011, 133, 6061; CrossRef S. T. Friis, R. H. Taaning, A. T. Lindhart, and T. Skrydstrup, J. Am. Chem. Soc., 2011, 133, 18114. CrossRef
4. T. Fujihara, T. Iwai, J. Terao, and Y. Tsuji, Synlett, 2010, 2537. CrossRef
5. M. C. Willis, Chem. Rev., 2010, 110, 725; CrossRef Y. J. Park, J.-W. Park, and C.-H. Jun, Acc. Chem. Res., 2008, 41, 222. CrossRef
6. T. Fujihara, Y. Katafuchi, T. Iwai, J. Terao, and Y. Tsuji, J. Am. Chem. Soc., 2010, 130, 2094; CrossRef Y. Nakao, H. Idei, K. S. Kanyiva, and T. Hiyama, J. Am. Chem. Soc., 2009, 131, 5070; CrossRef Y. Tsuji, S. Yoshii, T. Ohsumi, T. Kondo, and Y. Watanabe, J. Organomet. Chem., 1987, 331, 379; CrossRef T. Kondo, T. Okada, and T. Mitsudo, Organometallics, 1999, 18, 4123. CrossRef
7. S. Ko, Y. Na, and S. Chang, J. Am. Chem. Soc., 2002, 124, 750; CrossRef E. J. Park, J. M. Lee, H. Han, and S. Chang, Org. Lett., 2006, 8, 4355; CrossRef S. Fabre, P. Kalck, and G. Lavigne, Angew. Chem., Int. Ed. Engl., 1997, 36, 1092; CrossRef I. J. B. Lin and H. Alper, J. Chem. Soc., Chem. Commun., 1989, 248. CrossRef
8. T. Iwai, T. Fujihara, J. Terao, and Y. Tsuji, J. Am. Chem. Soc., 2012, 134, 1268; CrossRef T. Iwai, T. Fujihara, J. Terao, and Y. Tsuji, J. Am. Chem. Soc., 2009, 131, 6668. CrossRef
9. R. A. Sheldon, Pure Appl. Chem., 2000, 72, 1233; CrossRef B. M. Trost, Acc. Chem. Res., 2002, 35, 695. CrossRef
10. K. Ueura, T. Sato, and M. Miura, Org. Lett., 2007, 9, 1407; CrossRef Y. Unoh, K. Hirano, T. Satoh, and M. Miura, Tetrahedron, 2013, 69, 4454. CrossRef
11. Y. Kajita, T. Kurahashi, and S. Matsubara, J. Am. Chem. Soc., 2008, 130, 17226. CrossRef
12. K. Nakai, T. Kurahashi, and S. Matsubara, J. Am. Chem. Soc., 2011, 133, 11066. CrossRef
13. S. Saito and H. Yamamoto, Chem. Commun., 1997, 1585. CrossRef
14. A. Ooguri, K. Nakai, T. Kurahashi, and S. Matsubara, J. Am. Chem. Soc., 2009, 131, 13194. CrossRef
15. Carbon Dioxide as Chemical Feedstock; ed. by M. Aresta, Wiley-VCH, Weinheim, 2010; CrossRef T. Sakakura, J.-C. Choi, and H. Yasuda, Chem. Rev., 2007, 107, 2365. CrossRef
16. Y. Tsuji and T. Fujihara, Chem. Commun., 2012, 48, 9956; CrossRef L. Zang and Z. Hou, Chem. Sci., 2013, 4, 3395; CrossRef K. Huang, C.-L. Sun, and Z.-J. Shi, Chem. Soc. Rev., 2011, 40, 2435; CrossRef M. Cokoja, C. Bruckmeier, B. Rieger, W. A. Herrmann, and F. E. Kühn, Angew. Chem. Int. Ed., 2011, 50, 8510. CrossRef
17. T. Tsuda, S. Morikawa, R. Sumiya, and T. Saegusa, J. Org. Chem., 1988, 53, 3140. CrossRef
18. J. Louie, J. E. Gibby, M. V. Farnworth, and T. N. Tekavec, J. Am. Chem. Soc., 2002, 124, 15188. CrossRef
19. Y. Kishimoto and I. Mitani, Synlett, 2005, 2141. CrossRef
20. T. Fujihara, Y. Tani, K. Semba, J. Terao, and Y. Tsuji, Angew. Chem. Int. Ed., 2012, 51, 11487. CrossRef
21. T. Iwai, T. Fujihara, J. Terao, and Y. Tsuji, J. Am. Chem. Soc., 2010, 132, 9602. CrossRef
22. K. Nakai, T. Kurahashi, and S. Matsubara, Org. Lett., 2013, 15, 856. CrossRef
23. Y. Nakao, S. Oda, and T. Hiyama, J. Am. Chem. Soc., 2004, 126, 13904; CrossRef Y. Nakao, A. Yada, S. Ebata, and T. Hiyama, J. Am. Chem. Soc., 2007, 129, 2428; CrossRef N. M. Brunkan, D. M. Brestensky, and W. D. Jones, J. Am. Chem. Soc., 2004, 126, 3627. CrossRef
24. Y. Yoshino, T. Kurahashi, and S. Matsubara, J. Am. Chem. Soc., 2009, 131, 7494. CrossRef
25. Y. Kajita, T. Kurahashi, and S. Matsubara, J. Am. Chem. Soc., 2008, 130, 6058. CrossRef
26. T. Miura, M. Yamauchi, and M. Murakami, Org. Lett., 2008, 10, 3085. CrossRef
27. M. Yamauchi, M. Morimoto, T. Miura, and M. Murakami, J. Am. Chem. Soc., 2010, 132, 54. CrossRef
28. N. Guimond, C. Gouliaras, and K. Fagnou, J. Am. Chem. Soc., 2010, 132, 6908. CrossRef
29. N. Guimond, S. I. Gorelsky, and K. Fagnou, J. Am. Chem. Soc., 2011, 133, 6449. CrossRef
30. B. Li, H. Feng, S. Xu, and B. Wang, Chem. Eur. J., 2011, 17, 12573. CrossRef
31. T. K. Hyster and T. Rovis, J. Am. Chem. Soc., 2010, 132, 10565. CrossRef
32. L. Ackermann, A. Lygin, and N. Hofmann, Angew. Chem. Int. Ed., 2011, 50, 6379. CrossRef
33. H. Shiota, Y. Ano, Y. Aihara, Y. Fukumoto, and N. Chatani, J. Am. Chem. Soc., 2011, 133, 14952. CrossRef