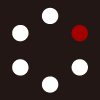
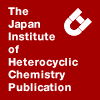
HETEROCYCLES
An International Journal for Reviews and Communications in Heterocyclic ChemistryWeb Edition ISSN: 1881-0942
Published online by The Japan Institute of Heterocyclic Chemistry
e-Journal
Full Text HTML
Received, 8th November, 2013, Accepted, 26th November, 2013, Published online, 3rd December, 2013.
DOI: 10.3987/COM-13-12880
■ Synthesis and Cyclization of a Proposed Biosynthetic Epoxy Intermediate of a Marine Monocyclic Ether Amide, Brevisamide
Tomohiro Shirai, Yuki Takimoto, Takefumi Kuranaga, Kazuo Tachibana, Masayuki Satake,* Daniel G. Baden, and Jeffrey L. C. Wright
Department of Chemistry, Graduate School of Science, The University of Tokyo, 7-3-1 Hongo, Bunkyo-ku, Tokyo 113-0033, Japan
Abstract
Proposed biosynthetic intermediates of a marine monocyclic ether alkaloid, brevisamide (1) were synthesized for biosynthetic studies on the marine ladder-frame polyethers. The intermediates comprising a linear backbone with trans-olefin (2) and epoxide (3) functionality were synthesized via a Suzuki–Miyaura cross coupling reaction and a Katsuki–Sharpless asymmetric epoxidation reaction. In protic solvents, 3 was unstable and readily cyclized to an unnatural 5-membered ether ring compound (4).INTRODUCTION
The red tide dinoflagellate Karenia brevis, produces a variety of ladder-frame polyethers, brevetoxins1-3 brevenal,4 brevisin,5 and tamulamides.6 A monocyclic ether amide, brevisamide (1)7 was also isolated from K. brevis together with those polyethers. The brevetoxins and brevenal are characterized by ladder-frame polyether scaffolds, while 1 consists of a single tetrahydropyran ring with a 3,4-dimethylhepta-2,4-dienal side chain and an acetylated terminal amine. The absolute configuration of 1 was determined by our chemical synthesis and a modified Mosher method.8,9 Following this, various synthetic studies of 1 including formal total syntheses were reported by independent groups.10-16 This cyclic ether amide has the same structural features of brevenal and brevisin containing the A-ring portion and the dienal side chain (Figure 1). Although intriguing 13C-incorporation patterns of the brevetoxins17 and yessotoxin produced by Protoceratium reticulatum18 were reported, a lack of genetic information and the notoriously low production of metabolites by dinoflagellates have hindered a complete understanding of the biosynthesis of the ladder-frame polyethers, specifically the formation of the fused or ladder-frame polyether ring systems. One particularly appealing hypothesis on the formation of these ladder-frame structures is that fused ether rings are formed by a stepwise or cascading series of an epoxide-opening process starting from a putative polyepoxide intermediate.9,19 Recent 18O labeling experiments of yessotoxin showed that all ether oxygens were labeled from 18O2.20 This result suggests that the ether oxygens of yessotoxin are introduced into the molecule by monooxygenation after the polyketide chain is constructed. Stereoselective epoxidation of an E-olefin intermediate affords an epoxy intermediate, and a subsequent endo-tet epoxide-opening process gives the desired cyclic ether compound. However, there have been no reports concerning the isolation of either of these proposed linear polyene and polyepoxide intermediates from the dinoflagellate. In order to obtain clues about this intriguing biosynthetic route, we undertook synthesis of an E-olefin (2) and the putative epoxide (3) intermediate (Figure 1) of 1 as artificial substrates and standard materials to explore monooxygenation and epoxy ring-opening enzymes present in the dinoflagellates. We reported synthesis of the E-olefin intermediate21 using a Suzuki–Miyaura cross coupling22 as the key reaction but the yield of the coupling reaction was not satisfiable. During the synthetic work of the intermediates, we improved the yield of the coupling reaction by optimizing the substrate in the Suzuki–Miyaura cross coupling reaction. In this paper we report successful synthesis of the proposed epoxy intermediate 3 using a Suzuki–Miyaura cross coupling and a Katsuki-Sharpless asymmetric epoxidation23 and improvement of synthesis of the E-olefin intermediate 2.
RESULTS AND DISCUSSION
Our synthetic strategy using Suzuki–Miyaura cross coupling and Katsuki–Sharpless asymmetric epoxidation as key reactions is shown in Scheme 1. A common intermediate, allylic alcohol 5 was synthesized by coupling between bromodienol side chain fragment 68 and iodide fragment 7 which has a TES protected primary alcohol. A non protected allylic alcohol was converted to an amide, after deprotection finally dienol was oxidized to generate the E-olefin intermediate 2. The epoxy intermediate 3 was converted from 5 by Katsuki–Sharpless asymmetric epoxidation to introduce an epoxide in the molecule.
Optically active homoallylic alcohol 8 was prepared stereoselectively following our previous synthesis.21 Protection of the secondary alcohol with TESOTf gave TES ether 9. The TES group was used because deprotection could be conducted under a milder condition than that of a TBS group in the final step. Hydroboration with 9-BBN followed by oxidative work-up, oxidation with TEMPO and PhI(OAc)2, and a one-pot Wittig reaction with Ph3PCHCO2Et afforded enoate 10 in 88% yield for two steps from the TES ether 9. Only an E isomer was isolated. Removal of the MPM group with DDQ in phosphate buffer/CH2Cl2 generated primary alcohol 11 in 92% yield. The resultant alcohol was converted to iodoenoate 12 with imidazole, PPh3, and I2 in 97% yield. The iodoenoate 12 was reduced with DIBALH to give allylic alcohol, and then the alcohol was protected by TESOTf to afford the iodide fragment 7 in 94% yield for two steps.
Connection of the bromodienol side chain fragment 6 and the iodide fragment 7 was accomplished by Suzuki–Miyaura cross coupling (Scheme 2).22,24 Treatment of 7 with t-BuLi and B-OMe-9-BBN produced a borate intermediate which was reacted in situ with the bromodienol 6 in the presence of aqueous Cs2CO3 and a catalytic amount of Pd(PPh3)4 to give rise a cross-coupled product. The yield of the coupling reaction was markedly improved compared with the synthesis of the olefin intermediate21 because the protected primary alcohol was used for the coupling reaction instead of the acetylated amine.
The crude product was treated with AcOH to give allylic alcohol 5 in 65% yield for two steps together with an undesired diol in 13% yield.
The resultant allylic alcohol 5 was converted to amide 14 in 70% yield over four steps. Removal of the protective groups with TBAF followed by chemoselective oxidation of the allylic alcohol at C-1 with MnO2 led to the putative biosynthetic olefin precursor 2 in 98% yield (Scheme 3). The overall yield of 2 was improved to 13% with 18 longest linear steps.
Asymmetric epoxidation of the allylic alcohol 5 was accomplished by treatment with (+)-DET and Ti(OiPr)4. The resultant epoxy alcohol 16 was converted to the iodide using imidazole, PPh3, and I2 which in turn was converted to the azide with NaN3 and then reduced to afford an amine. This amine was acetylated with acetic anhydride to give amide 17 in 67% yield over four steps. Removal of the protective groups with TBAF followed by chemoselective oxidation of the allylic alcohol at C-1 with MnO2 led to the putative biosynthetic epoxy precursor 3 in 50% yield for two steps (Scheme 4). During purification on silica gel with Et3N, isobrevisamide (4) was formed spontaneously by exo-tet opening of the epoxide and so the desired product 3 was obtained as a mixture with 4.
The epoxy intermediate was converted readily to unnatural isobrevisamide (4) by an exo-tet epoxide-opening mechanism under both acidic and basic conditions (Table 1). Even under neutral conditions such as Tris buffer or D2O, the epoxy intermediate 3 was converted completely to isobrevisamide in a few hours. In contrast, when 3 was kept in benzene at low temperature, the epoxide-opening reaction did not occur for 2 days. Protonation or hydrogen bonding of epoxide was presumed to cause the epoxide-opening reaction. Without enzymes in protic solvents, the epoxy intermediate was converted not to the endo-tet product, brevisamide but to the exo-tet product, isobrevisamide. Thus, the non-enzymatic cyclization of 3 conformed to the empirical rules by Baldwin.25 Our results here strongly suggest that an endo-tet epoxide-opening process of marine ladder frame polyether biosynthesis is catalyzed by an enzyme. Intriguing experimental results were reported that regioselective epoxide-opening cascade was promoted in water using polyepoxide intermediates with a preformed tetrahydropyran as a substrate which preceded a step wise mechanism.26 Future studies to explore the biosynthesis of marine ladder-frame polyethers will be directed towards epoxide-opening enzymes in dinoflagellates using the intermediate 3.
EXPERIMENTAL
General: All reactions sensitive to air and/or moisture were carried out in oven-dried (>100 °C) glassware under argon or nitrogen atmosphere, and under anhydrous conditions otherwise noted. Anhydrous dichloromethane (CH2Cl2), diethyl ether (Et2O), and tetrahydrofuran (THF) were purchased from Kanto Chemical Co. Inc and used without further drying. All other reagents and solvents were purchased at highest commercial grade and used as supplied unless otherwise noted. Analytical thin-layer chromatography (TLC) was performed using E. Merck silica gel 60 F254 plates. Column chromatography was performed using Kanto Chemical silica gel 60N (40–100 mesh, spherical, neutral). Optical rotations were recorded on a JASCO DIP-350 digital polarimeter. 1H and 13C NMR spectra were measured on JEOL ECA-500 and ECX-400 spectrometers, and chemical shift values are reported in ppm (δ) with reference to internal residual solvent [1H NMR, CHCl3 (7.24), CHD2OD (3.31), C6HD5 (7.21); 13C NMR, CDCl3 (77.0), CD3OD (49.0), C6D6 (128.0)]. Coupling constants (J) are reported in hertz (Hz). The following abbreviations are used to designate the multiplicities: s = singlet, d = doublet, t = triplet, q = qualtet, m = multiplet, br = broad. Low- and high-resolution mass spectra were recorded on a JEOL JMS-700P mass spectrometer under fast atom bombardment (FAB) conditions using m-nitrobenzyl alcohol (NBA) as a matrix and a JEOL JMS-T100TD mass spectrometer under direct analysis in real time (DART) conditions.
Triethyl((3S,4S)-1-(4-methoxybenzyloxy)-4-methylhex-5-en-3-yloxy)silane (9). To a solution of homoallylic alcohol 8 (2.99 g, 11.9 mmol) in CH2Cl2 (60 mL) at 0 °C were added 2,6-lutidine (2.0 mL, 14.3 mmol) and TESOTf (3.0 mL, 13.1 mmol). After being stirred at that temperature for 1 h, the reaction mixture was diluted with saturated aqueous NH4Cl and Et2O. The organic layer was separated, and the aqueous layer was extracted with Et2O. The combined organic layer was washed with saturated aqueous NH4Cl and brine, dried over MgSO4, filtered, and concentrated under reduced pressure. The residue was subjected to column chromatography (2 to 5% EtOAc/hexane) to give the TES ether 9 (3.88 g, 89%) as a colorless oil: [α]D21 –30.0 (c 0.34, CHCl3); 1H NMR (500 MHz, CDCl3) δ 7.24 (d, J = 8.8 Hz, 2H), 6.86 (d, J = 8.4 Hz, 2H), 5.86 (ddd, J = 17.2, 10.5, 6.8 Hz, 1H), 5.00 (d, J = 10.5 Hz, 1H), 4.99 (d, J = 17.2 Hz, 1H), 4.42 (d, J = 11.4 Hz, 1H), 4.38 (d, J = 11.4 Hz, 1H), 3.79 (s, 3H), 3.73 (dt, J = 8.4, 4.3 Hz, 1H), 3.49 (dd, J = 7.6, 5.9 Hz, 2H), 2.27 (m, 1H), 1.73 (m, 1H), 1.63 (m, 1H), 0.95 (d, J = 6.7 Hz, 3H), 0.94 (t, J = 8.0 Hz, 9H), 0.58 (q, J = 8.0 Hz, 6H); 13C NMR (100 MHz, CDCl3) δ 159.1, 140.8, 130.7, 129.3, 114.3, 113.7, 73.1, 72.6, 66.9, 55.2, 43.4, 33.7, 15.0, 7.0, 5.1; HRMS (FAB) calcd for C21H36NaO3Si [(M+Na)+] 387.2331, found 387.2351.
(5S,6S,E)-Ethyl 8-(4-methoxybenzyloxy)-5-methyl-6-(triethylsilyloxy)oct-2-enoate (10). To a solution of the TES ether 9 (2.16 g, 5.92 mmol) in THF (25 mL) was added 9-BBN (0.5 M solution in THF, 17.8 mL, 8.90 mmol). After being stirred at room temperature for 1.5 h, the reaction mixture was cooled to 0 °C. Then saturated aqueous NaHCO3 (24 mL) and 30% H2O2 (16 mL) were added. After being stirred at room temperature for 2 h, the resultant mixture was extracted with EtOAc. The combined organic layer was washed with brine, dried over MgSO4, filtered, and concentrated under reduced pressure. The residue was subjected to column chromatography (25% EtOAc/hexane) to give the alcohol that was used in the next reaction without further purification.
To a solution of the above alcohol in CH2Cl2 (50 mL) were added TEMPO (274 mg, 1.76 mmol) and iodobenzene diacetate (2.64 g, 8.20 mmol). After being stirred at room temperature for 4 h, disappearance of the alcohol was confirmed by TLC. Then to the reaction mixture was added Ph3PCHCO2Et (3.06 g, 8.78 mmol). After being stirred at room temperature for 16 h, the reaction mixture was diluted with Et2O and quenched with 1:1 mixture of saturated aqueous NaHCO3 and Na2S2O3. The organic layer was separated, and the aqueous layer was extracted with Et2O. The combined organic layer was washed with brine, dried over MgSO4, filtered, and concentrated under reduced pressure. The residue was subjected to column chromatography (5% EtOAc/hexane) to give the α,β-unsaturated ester 10 (2.36 g, 88% for 2 steps) as a colorless oil: [α]D24 –12.1 (c 0.28, CHCl3); 1H NMR (500 MHz, CDCl3) δ 7.23 (d, J = 9.3 Hz, 2H), 6.91 (ddd, J = 15.6, 8.4, 6.8 Hz, 1H), 6.86 (d, J = 8.4 Hz, 2H), 5.79 (d, J = 15.6 Hz, 1H), 4.42 (d, J = 11.4 Hz, 1H), 4.38 (d, J = 11.4 Hz, 1H), 4.16 (q, J = 7.2 Hz, 2H), 3.78 (s, 3H), 3.75 (m, 1H), 3.46 (m, 2H), 2.43 (m, 1H), 1.90 (m, 1H), 1.70 (m, 2H), 1.60 (m, 1H), 1.27 (t, J = 7.2 Hz, 3H), 0.92 (t, J = 8.0 Hz, 9H), 0.82 (d, J = 6.7 Hz, 3H), 0.55 (q, J = 8.0 Hz, 6H); 13C NMR (100 MHz, CDCl3) δ 166.6, 159.1, 148.8, 130.5, 129.3, 122.2, 113.7, 72.6 (2C), 67.1, 60.1, 55.2, 38.4, 34.6, 32.8, 14.7, 14.2, 6.9, 5.1; HRMS (FAB) calcd for C25H42NaO5Si [(M+Na)+] 473.2700, found 473.2671.
(5S,6S,E)-Ethyl 8-hydroxy-5-methyl-6-(triethylsilyloxy)oct-2-enoate (11). To a solution of α,β-unsaturated ester 10 (190 mg, 0.422 mmol) in CH2Cl2/pH 7 phosphate buffer (5:1, 4.2 mL) at 0 °C was added DDQ (201 mg, 0.885 mmol). After being stirred at that temperature for 1 h, the reaction mixture was quenched with saturated aqueous NaHCO3 and diluted with Et2O. The organic layer was separated, and the aqueous layer was extracted with Et2O. The combined organic layer was washed with saturated aqueous NaHCO3 and brine, dried over MgSO4, filtered, and concentrated under reduced pressure. The residue was subjected to column chromatography (12% EtOAc/hexane) to give the primary alcohol 11 (129 mg, 92%) as a colorless oil: [α]D25 –19.9 (c 0.22, CHCl3); 1H NMR (500 MHz, CDCl3) δ 6.90 (ddd, J = 15.5, 8.4, 6.3 Hz, 1H), 5.80 (d, J = 15.5 Hz, 1H), 4.16 (q, J = 7.2 Hz, 2H), 3.81 (m, 1H), 3.72 (m, 2H), 2.49 (m, 1H), 2.02 (ddd, J = 14.2, 9.7, 8.9 Hz, 1H), 1.89 (m, 1H), 1.76 (m, 1H), 1.63 (m, 1H), 1.26 (t, J = 7.2 Hz, 3H), 0.94 (t, J = 8.0 Hz, 9H), 0.84 (d, J = 7.2 Hz, 3H), 0.60 (q, J = 8.0 Hz, 6H); 13C NMR (100 MHz, CDCl3) δ 166.6, 148.4, 122.4, 74.7, 60.8, 60.2, 38.5, 34.0 (2C), 15.4, 14.2, 6.9, 5.1; HRMS (FAB) calcd for C17H34NaO4Si [(M+Na)+] 353.2124, found 353.2142.
(5S,6S,E)-Ethyl 8-iodo-5-methyl-6-(triethylsilyloxy)oct-2-enoate (12). To a solution of the primary alcohol 11 (105 mg, 0.318 mmol) in toluene (4 mL) at room temperature were added imidazole (32.4 mg, 0.477 mmol), PPh3 (100 mg, 0.382 mmol), and I2 (120 mg, 0.473 mmol). After being stirred at room temperature for 1 h, the reaction mixture was quenched with saturated aqueous Na2S2O3. The organic layer was separated, and the aqueous layer was extracted with Et2O. The combined organic layer was washed with brine, dried over Na2SO4, filtered, and concentrated under reduced pressure. The residue was subjected to column chromatography (60% EtOAc/hexane) to give the iodide 12 (136 mg, 97%) as a colorless oil: [α]D25 –24.6 (c 0.29, CHCl3); 1H NMR (500 MHz, CDCl3) δ 6.89 (ddd, J = 15.6, 8.4, 6.7 Hz, 1H), 5.80 (d, J = 15.6 Hz, 1H), 4.16 (q, J = 7.2 Hz, 2H), 3.68 (m, 1H), 3.24 (ddd, J = 9.7, 7.2, 5.5 Hz, 1H), 3.13 (ddd, J = 9.7, 8.0, 7.6 Hz, 1H), 2.46 (m, 1H), 1.86 (m, 3H), 1.74 (m, 1H), 1.26 (t, J = 7.2 Hz, 3H), 0.94 (t, J = 8.0 Hz, 9H), 0.82 (d, J = 6.7 Hz, 3H), 0.60 (q, J = 8.0 Hz, 6H); 13C NMR (100 MHz, CDCl3) δ 166.5, 148.3, 122.5, 75.6, 60.2, 38.2, 36.3, 34.3, 14.9, 14.3, 7.0, 5.2, 3.8; HRMS (FAB) calcd for C17H33NaIO3Si [(M+Na)+] 463.1141, found 463.1150.
(9S,10S,E)-3,3,12,12-Tetraethyl-10-(2-iodoethyl)-9-methyl-4,11-dioxa-3,12-disilatetradec-6-ene (7). To a solution of iodide 12 (107 mg, 0.243 mmol) in CH2Cl2 (2.5 mL) at –78 °C was added DIBALH (1.02 M solution in hexane, 0.50 mL, 0.510 mmol). After being stirred at –78 °C for 0.5 h, the reaction mixture was quenched with MeOH (0.1 mL), allowed to warm to room temperature, and diluted with EtOAc and saturated aqueous potassium sodium tartrate. The resultant mixture was stirred at room temperature until the layers became clear. The organic layer was separated, and the aqueous layer was extracted with EtOAc. The combined organic layer was washed with brine, dried over MgSO4, filtered, and concentrated under reduced pressure. The residual crude allylic alcohol was used in the next reaction without further purification.
To a solution of above allylic alcohol in CH2Cl2 (2 mL) were added 2,6-lutidine (0.04 mL, 0.345 mmol) and TESOTf (0.06 mL, 0.267 mmol) at 0 °C. After being stirred at that temperature for 15 min, the reaction mixture was diluted with saturated aqueous NH4Cl and Et2O. The organic layer was separated, and the aqueous layer was extracted with Et2O. The combined organic layer was washed with saturated aqueous NH4Cl and brine, dried over MgSO4, filtered, and concentrated under reduced pressure. The residue was subjected to column chromatography (1% EtOAc/hexane) to give the iodide fragment 7 (117 mg, 94% for 2 steps) as a colorless oil: [α]D27 –22.6 (c 0.28, CHCl3); 1H NMR (500 MHz, CDCl3) δ 5.57 (m, 2H), 4.10 (d, J = 3.8 Hz, 2H), 3.65 (m, 1H), 3.23 (m, 1H), 3.15 (dt, J = 8.0, 8.0 Hz, 1H), 2.29 (m, 1H), 1.88 (m, 2H), 1.70 (m, 1H), 1.59 (m, 1H), 0.95 (t, J = 8.0 Hz, 18H), 0.81 (d, J = 6.7 Hz, 3H), 0.60 (q, J = 8.0 Hz, 6H), 0.59 (q, J = 8.0 Hz, 6H); 13C NMR (100 MHz, CDCl3) δ 130.6, 130.2, 75.8, 63.6, 38.7, 36.8, 34.4, 14.7, 7.0, 6.8, 5.3, 4.5, 4.1; HRMS (FAB) calcd for C21H44IO2Si2 [(M–H)–] 511.1924, found 511.1933.
(2E,5S,6S,9E,11E)-13-(tert-Butyldiphenylsilyloxy)-5,10,11-trimethyl-6-(triethylsilyloxy)trideca-2,9,11-trien-1-ol (5). To a solution of the iodide 7 (217 mg, 0.423 mmol) in anhydrous Et2O (4.2 mL) at room temperature was added B-OMe-9-BBN (1.0 M solution in hexane, 1.1 mL, 1.1 mmol). After cooling to –78 °C, t-BuLi (1.58 M solution in pentane, 0.96 mL, 1.52 mmol) was added rapidly, followed by THF (4.2 mL). Then the reaction mixture was stirred at –78 °C for 10 min and allowed to warm to room temperature for 1.5 h. To the mixture were added 3 M aqueous Cs2CO3 (1.4 mL), the solution of the TBDPS-bromodienol 6 (250 mg, 0.582 mmol) in DMF (4.2 mL), and Pd(PPh3)4 (24.4 mg, 0.0212 mmol). After being stirred at 45 °C for 22 h, the resultant mixture was treated with saturated aqueous NH4Cl. The organic layer was separated, and the aqueous layer was extracted with Et2O. The combined organic layer was washed with brine, dried over Na2SO4, filtered, and concentrated under reduced pressure. The residue was subjected to column chromatography (1% EtOAc/hexane) to give the crude coupled product that was used in the next reaction without further purification.
To a solution of above crude product in THF/H2O (3:1, 4 mL) at 0 °C was added AcOH (1 mL) dropwise. After being stirred at that temperature for 2 h, the reaction mixture was quenched with saturated aqueous NaHCO3. The organic layer was separated, and the aqueous layer was extracted with Et2O. The combined organic layer was washed with saturated aqueous NaHCO3 and brine, dried over Na2SO4, filtered, and concentrated under reduced pressure. The residue was subjected to column chromatography (10% to 50% EtOAc/hexane) to give the allylic alcohol 5 (172 mg, 65% for 2 steps) and diol (28.7 mg, 13% for 2 steps) as a colorless oil:
Spectroscopic data for 5
[α]D27 –5.5 (c 0.31, CHCl3); 1H NMR (500 MHz, CDCl3) δ 7.68 (d, J = 6.3 Hz, 4H), 7.42–7.35 (m, 6H), 5.64 (m, 3H), 5.47 (t, J = 7.2 Hz, 1H), 4.35 (d, J = 5.9 Hz, 2H), 4.08 (br, 2H), 3.58 (m, 1H), 2.26 (dt, J = 13.5, 4.2 Hz, 1H), 2.18 (m, 1H), 2.03 (m, 1H), 1.80 (m, 1H), 1.75 (s, 3H), 1.60 (m, 1H), 1.58 (s, 3H), 1.51–1.38 (m, 2H), 1.03 (s, 9H), 0.95 (t, J = 8.0 Hz, 9H), 0.82 (d, J = 7.2 Hz, 3H), 0.59 (q, J = 8.0 Hz, 6H); 13C NMR (100 MHz, CDCl3) δ 136.7, 135.9, 135.6, 133.9, 132.5, 130.0, 129.5, 127.6, 126.6, 125.1, 75.5, 63.8, 61.9, 38.3, 35.1, 33.2, 26.8, 25.2, 19.2, 14.3, 14.1, 13.9, 7.0, 5.3; HRMS (FAB) calcd for C38H60NaO3Si2 [(M+Na)+] 643.3979, found 643.3946.
Spectroscopic data for diol (13)
[α]D27 –6.6 (c 0.31, CHCl3); 1H NMR (500 MHz, CDCl3) δ 7.68 (dd, J = 8.0, 1.5 Hz, 4H), 7.42–7.34 (m, 6H), 5.66 (m, 3H), 5.50 (t, J = 7.2 Hz, 1H), 4.35 (d, J = 5.9 Hz, 2H), 4.08 (br, 2H), 3.54 (br, 1H), 2.31–2.13 (m, 3H), 1.95 (m, 1H), 1.77 (s, 3H), 1.58 (s, 3H), 1.51 (m, 1H), 1.41 (br, 1H), 1.36 (br, 1H), 1.04 (s, 9H), 0.87 (d, J = 6.8 Hz, 3H); 13C NMR (100 MHz, CDCl3) δ 136.6, 136.4, 135.6, 133.9, 131.5, 130.6, 129.5, 127.6, 126.2, 125.4, 74.3, 63.6, 61.9, 38.3, 36.3, 34.4, 26.8, 25.4, 19.2, 14.2, 13.9, 13.4; HRMS (FAB) calcd for C32H46NaO3Si [(M+Na)+] 529.3114, found 529.3132.
N-((2E,5S,6S,9E,11E)-13-(tert-Butyldiphenylsilyloxy)-5,10,11-trimethyl-6-(triethylsilyloxy)trideca- 2,9,11-trienyl)acetamide (14). To a solution of the allylic alcohol 5 (49.5 mg, 0.0797 mmol) in toluene (1 mL) at room temperature were added imidazole (21.7 mg, 0.319 mmol), PPh3 (41.8 mg, 0.159 mmol) and I2 (60.7 mg, 0.239 mmol). After being stirred at room temperature for 1 h, the reaction mixture was quenched with saturated aqueous Na2S2O3. The organic layer was separated, and the aqueous layer was extracted with Et2O. The combined organic layer was washed with brine, dried over Na2SO4, filtered, and concentrated under reduced pressure. The residue was subjected to column chromatography (2% EtOAc/hexane) to give the iodide that was immediately used in the next reaction without further purification.
To a solution of the above iodide in DMF (1 mL) at 0 °C was added NaN3 (70.4 mg, 0.108 mmol). After being stirred at that temperature for 2.5 h, the reaction mixture was diluted with Et2O and H2O. The organic layer was separated, and the aqueous layer was extracted with Et2O. The combined organic layer was washed with brine, dried over Na2SO4, filtered, and concentrated under reduced pressure. The residual crude azide was used in the next reaction without further purification.
To a solution of the above azide in THF (2 mL) at 0 °C was added PPh3 (28.0 mg, 0.107 mmol). After being stirred at room temperature for 18 h, the reaction mixture was cooled to 0 °C. Then H2O (0.15 mL) was added dropwise. After being stirred at 40 °C for 17 h, the resultant mixture was diluted with saturated aqueous NaHCO3. The organic layer was separated, and the aqueous layer was extracted with Et2O. The combined organic layer was washed with saturated aqueous NaHCO3, dried over Na2SO4, filtered, and concentrated under reduced pressure. The residual crude amine was used in the next reaction without further purification.
To a solution of the above amine in pyridine (1 mL) was added Ac2O (1 mL). After being stirred at room temperature for 7.5 h, the reaction mixture was diluted with toluene, and concentrated under reduced pressure. The residue was subjected to column chromatography (25 to 50% EtOAc/hexane) to give the allylic amide 14 (37.1 mg, 70% for 4 steps) as a colorless oil: [α]D28 –5.6 (c 0.10, CHCl3); IR (film) cm–1; 1H NMR (500 MHz, CDCl3) δ 7.68 (dd, J = 6.5, 1.5 Hz, 4H), 7.42–7.34 (m, 6H), 5.65 (t, J = 5.9 Hz, 1H), 5.56 (dt, J = 15.2, 7.6 Hz, 1H), 5.45 (m, 3H), 4.35 (d, J = 5.9 Hz, 2H), 3.80 (m, 2H), 3.56 (m, 1H), 2.19 (m, 2H), 2.03 (m, 1H), 1.96 (s, 3H), 1.78 (m, 1H), 1.75 (s, 3H), 1.59 (m, 1H), 1.58 (s, 3H), 1.45 (m, 2H), 1.03 (s, 9H), 0.95 (t, J = 8.0 Hz, 9H), 0.82 (d, J = 6.8 Hz, 3H), 0.58 (q, J = 8.0 Hz, 6H); 13C NMR (100 MHz, CDCl3) δ 169.7, 136.7, 135.9, 135.6, 133.9, 133.1, 129.5, 127.6, 126.7, 126.6, 75.5, 61.9, 41.6, 38.3, 35.1, 33.2, 26.8, 25.2, 23.3, 19.2, 14.2, 14.1, 13.9, 7.0, 5.3; HRMS (FAB) calcd for C40H63NNaO3Si2 [(M+Na)+] 684.4244, found 684.4264.
N-((2E,5S,6S,9E,11E)-6,13-Dihydroxy-5,10,11-trimethyltrideca-2,9,11-trienyl)acetamide (15). To a solution of the allylic amide 14 (26.1 mg, 0.0394 mmol) in THF (1 mL) at 0 °C was added TBAF (1 M solution in THF, 0.16 mL, 0.16 mmol). After being stirred at room temperature for 11 h, the reaction mixture was diluted with saturated aqueous NH4Cl and CHCl3. The organic layer was separated, and the aqueous layer was extracted with CHCl3. The combined organic layer was washed with saturated aqueous NH4Cl, dried over Na2SO4, filtered, and concentrated under reduced pressure. The residue was subjected to column chromatography (5% MeOH/EtOAc) to give the diol 15 (12.2 mg, quant.) [α]D27 –12.8 (c 0.26, CHCl3); IR (film) 3287, 2928, 2869, 1658, 1641, 1631, 1441, 1378, 1284, 1110, 1001, 970 cm–1; 1H NMR (500 MHz, CD3OD) δ 5.66–5.58 (m, 3H), 5.46 (dt, J = 15.1, 5.9 Hz, 1H), 4.22 (d, J = 6.3 Hz, 2H), 3.72 (d, J = 5.9 Hz, 2H), 3.47 (dt, J = 4.2, 6.3 Hz, 1H), 2.30 (ddd, J = 14.5, 14.5, 7.2 Hz, 1H), 2.19 (m, 2H), 1.93 (s, 3H), 1.92–1.87 (m, 1H), 1.81 (s, 3H), 1.80 (s, 3H), 1.58–1.49 (m, 3H), 0.87 (d, J = 7.2 Hz, 3H); 13C NMR (100 MHz, CDCl3) δ 172.9, 139.3, 137.3, 132.8, 128.2, 128.0, 125.5, 74.6, 60.1, 42.3, 39.8, 37.4, 35.3, 26.4, 22.5, 14.2, 14.2, 14.0; HRMS (FAB) calcd for C18H31O3NNa [(M+Na)+] 332.2202, found 332.2214.
Olefin precursor (2). To a solution of the diol 15 (27.4 mg, 0.0885 mmol) in CH2Cl2 (3 mL) at 0 °C was added MnO2 (274 mg). After being stirred at that temperature for 2 h, the mixture was filtered through celite and concentrated under reduced pressure. The residue was subjected to column chromatography (5% MeOH/EtOAc) to give the olefin precursor 2 (26.7 mg, 98%) as a colorless oil: [α]D27 –14.3 (c 0.12, CHCl3); IR (film) 3299, 2929, 1657, 1442, 1375, 1283, 1252, 1153, 1046, 970, 843, 750 cm–1; 1H NMR (500 MHz, CD3OD) δ 10.10 (d, J = 8.0 Hz, 1H), 6.26 (dd, J = 7.0, 7.0 Hz, 1H), 6.05 (d, J = 8.0 Hz, 1H), 5.61 (ddd, J = 15.1, 7.5, 7.5 Hz, 1H), 5.47 (dt, J = 15.1, 5.9 Hz, 1H), 3.72 (d, J = 5.9 Hz, 2H), 3.48 (m, 1H), 2.41 (m, 1H), 2.34 (s, 3H), 2.30 (m, 1H), 2.21 (m, 1H), 1.93 (s, 3H), 1.93–1.87 (m, 1H), 1.88 (s, 3H), 1.56 (m, 3H), 0.89 (d, J = 6.7 Hz, 3H); 13C NMR (100 MHz, CDCl3) δ 194.4, 172.9, 161.0, 137.0, 132.7, 128.3, 126.2, 74.7, 42.3, 40.0, 37.4, 34.7, 27.2, 22.5, 14.5, 14.1, 14.0; HRMS (FAB) calcd for C18H29O3NNa [(M+Na)+] 330.2045, found 330.2059.
((2S,3S)-3-((2S,3S,6E,8E)-10-(tert-Butyldiphenylsilyloxy)-2,7,8-trimethyl-3-(triethylsilyloxy)deca-6,8-dienyl)oxiran-2-yl)methanol (16). To a suspension of the allylic alcohol 5 (246 mg, 0.396 mmol) and MS4A (247 mg) in CH2Cl2 (4 mL) at –30 °C were added diethyl L-(+)-tartrate (0.020 mL, 0.119 mmol) and Ti(OiPr)4 (0.023 mL, 0.0792 mmol). The reaction mixture was stirred at that temperature for 0.5 h, then to the mixture was added TBHP (5.5 M solution in nonane, 0.36 mL, 1.98 mmol). After being stirred at that temperature for 11 h, the mixture was filtered through celite, diluted with EtOAc, and quenched with saturated aqueous Na2S2O3. The organic layer was separated, and the aqueous layer was extracted with EtOAc. The combined organic layer was washed with brine, dried over Na2SO4, filtered, and concentrated under reduced pressure. The residue was subjected to column chromatography (10 to 15% EtOAc/hexane) to give the epoxide 16 (203 mg, 80%) as a colorless oil: [α]D28 –16.1 (c 0.21, CHCl3); 1H NMR (500 MHz, CDCl3) δ 7.68 (d, J = 8.0 Hz, 4H), 7.42–7.34 (m, 6H), 5.65 (t, J = 5.9 Hz, 1H), 5.47 (t, J = 7.1 Hz, 1H), 4.35 (d, J = 5.9 Hz, 2H), 3.91 (m, 1H), 3.63 (ddd, J = 12.6, 7.2, 4.2 Hz, 1H), 3.58 (m, 1H), 2.98 (dd, J = 6.3, 5.5 Hz, 1H), 2.90 (m, 1H), 2.19 (m, 1H), 2.04 (m, 1H), 1.80 (m, 1H), 1.75 (s, 3H), 1.65 (m, 1H), 1.58 (s, 3H), 1.51–1.36 (m, 2H), 1.34–1.26 (m, 1H), 1.03 (s, 9H), 0.95 (t, J = 8.0 Hz, 9H), 0.91 (d, J = 6.7 Hz, 3H), 0.58 (q, J = 8.0 Hz, 6H); 13C NMR (100 MHz, CDCl3) δ 136.7, 136.0, 135.6, 133.9, 129.5, 127.6, 126.4, 125.2, 75.8, 61.9, 61.6, 59.2, 55.0, 35.8, 34.4, 33.1, 26.8, 25.2, 19.2, 14.5, 14.1, 13.9, 7.0, 5.2; HRMS (FAB) calcd for C38H60NaO4Si2 [(M+Na)+] 659.3928, found 659.3950.
N-(((2S,3S)-3-((2S,3S,6E,8E)-10-(tert-Butyldiphenylsilyloxy)-2,7,8-trimethyl-3-(triethylsilyloxy)deca-6,8-dienyl)oxiran-2-yl)methyl)acetamide (17). To a solution of the epoxide 16 (28.7 mg, 0.0451 mmol) in toluene (1 mL) at room temperature were added imidazole (11.1 mg, 0.163 mmol), PPh3 (24.9 mg, 0.0949 mmol) and I2 (44.0 mg, 0.173 mmol). After being stirred at room temperature for 1.5 h, the reaction mixture was quenched with saturated aqueous Na2S2O3. The organic layer was separated, and the aqueous layer was extracted with Et2O. The combined organic layer was washed with brine, dried over Na2SO4, filtered, and concentrated under reduced pressure. The residue was subjected to column chromatography (5% EtOAc/hexane) to give the iodide that was immediately used in the next reaction without further purification.
To a solution of the above iodide in DMF (1 mL) at 0 °C was added NaN3 (25.2 mg, 0.388 mmol). After being stirred at room temperature for 1.5 h, the reaction mixture was diluted with Et2O and H2O. The organic layer was separated, and the aqueous layer was extracted with Et2O. The combined organic layer was washed with brine, dried over Na2SO4, filtered, and concentrated under reduced pressure. The residual crude azide was used in the next reaction without further purification.
To a solution of the above azide in THF (1 mL) at 0 °C was added PPh3 (12.2 mg, 0.0466 mmol). The reaction mixture was stirred at room temperature for 22 h, then to the mixture H2O (0.07 mL) was added dropwise. After being stirred at 40 °C for 19 h, the resultant mixture was diluted with saturated aqueous NaHCO3. The organic layer was separated, and the aqueous layer was extracted with Et2O. The combined organic layer was washed with saturated aqueous NaHCO3, dried over Na2SO4, filtered, and concentrated under reduced pressure. The residual crude amine was used in the next reaction without further purification.
To a solution of the above amine in pyridine (1 mL) was added Ac2O (1 mL). After being stirred at room temperature for 6 h, the reaction mixture was diluted with toluene, and concentrated under reduced pressure. The residue was subjected to column chromatography (30 to 40% EtOAc/hexane) to give the allylic amide 17 (20.6 mg, 67% for 4 steps) as a colorless oil: [α]D28 –20.6 (c 0.08, CHCl3); 1H NMR (500 MHz, CDCl3) δ 7.67 (d, J = 6.3 Hz, 4H), 7.41–7.34 (m, 6H), 5.69 (br, 1H), 5.65 (t, J = 5.9 Hz, 1H), 5.47 (t, J = 6.7 Hz, 1H), 4.35 (d, J = 5.9 Hz, 2H), 3.72 (ddd, J = 14.7, 5.9, 3.0 Hz, 1H), 3.56 (m, 1H), 3.22 (ddd, J = 14.7, 5.9, 5.5 Hz, 1H), 2.85 (m, 1H), 2.79 (ddd, J = 5.9, 5.9, 2.1 Hz, 1H), 2.18 (m, 1H), 2.03 (m, 1H), 1.98 (s, 3H), 1.80–1.69 (m, 2H), 1.74 (s, 3H), 1.57 (s, 3H), 1.50–1.35 (m, 2H), 1.29 (m, 1H), 1.03 (s, 9H), 0.94 (t, J = 8.0 Hz, 9H), 0.90 (d, J = 6.7 Hz, 3H), 0.57 (q, J = 8.0 Hz, 6H); 13C NMR (100 MHz, CDCl3) δ 170.2, 136.7, 136.0, 135.6, 133.9, 129.5, 127.6, 126.4, 125.2, 75.8, 61.9, 57.6, 56.1, 40.3, 35.7, 34.3, 33.1, 26.8, 25.2, 23.2, 19.2, 14.4, 14.1, 13.9, 7.0, 5.2; HRMS (FAB) calcd for C40H63NNaO4Si2 [(M+Na)+] 700.4193, found 700.4199.
Epoxy intermediate (3). To a solution of the epoxyamide 17 (5.9 mg, 0.00870 mmol) in THF (0.5 mL)at 0 °C was added TBAF (1 M solution in THF, 0.025 mL, 0.025 mmol) dropwise. After being stirred at that temperature for 4 h and at room temperature for 2 h, the reaction mixture was directly subjected to column chromatography (4% MeOH/EtOAc, contains 2% Et3N) to give diol that was used in the next reaction immediately.
To a solution of the above diol in CH2Cl2 (1 mL) at 0 °C was added MnO2 (88.8 mg). After being stirred at that temperature for 25 min, the mixture was filtered through celite and concentrated under reduced pressure to give the mixture of the epoxy intermediate 3 and isobrevisamide 4 (2.0 mg, 50% for 3, 21% for 4 ca. from 1H NMR, 2 steps) as an amorphous solid:
Estimated spectroscopic data for 3: 1H NMR (500 MHz, C6D6) δ 10.12 (d, J = 8.0 Hz, 1H), 6.20 (d, J = 8.0 Hz, 1H), 5.88 (t, J = 7.1 Hz, 1H), 3.54 (m, 1H), 3.43 (ddd, J = 14.7, 5.9, 5.5 Hz, 1H), 2.97 (ddd, J = 5.5, 3.0, 2.5 Hz, 1H), 2.66 (m, 1H), 2.56 (m, 1H), 2.19–2.06 (m, 2H), 1.83 (s, 3H), 1.71–1.64 (m, 1H), 1.60 (s, 3H), 1.62–1.56 (m, 1H), 1.54–1.43 (m, 1H), 1.51 (s, 3H), 1.42–1.31 (m, 1H), 1.31–1.22 (m, 1H), 0.86 (d, J = 6.7 Hz, 3H); 13C NMR (125 MHz, C6D6) δ 190.7, 169.2, 156.6, 134.1, 133.9, 126.1, 73.4, 57.4, 56.1, 40.6, 37.2, 36.3, 33.6, 26.3, 22.5, 14.1, 13.9, 13.7; HRMS (FAB) calcd for C18H29NNaO4 [(M+Na)+] 346.1994, found 346.2005.
Spectroscopic data for 4: [α]D29 –16.9 (c 0.22, CHCl3); 1H NMR (500 MHz, CDCl3) δ 10.12 (d, J = 8.0 Hz, 1H), 6.11 (t, J = 7.2 Hz, 1H), 6.06 (d, J = 8.0 Hz, 1H), 6.00 (br, 1H), 3.95 (ddd, J = 7.2, 6.7, 6.7 Hz, 1H), 3.84 (m, 1H), 3.63–3.57 (m, 2H), 3.29 (br, 1H), 3.14 (ddd, J = 13.5, 7.2, 5.0 Hz, 1H), 2.36–2.21 (m, 3H), 2.28 (s, 3H), 2.04 (m, 1H), 2.00 (s, 3H), 1.83 (s, 3H), 1.70 (ddd, J = 12.6, 6.8, 3.8 Hz, 1H), 1.58–1.45 (m, 2H), 0.92 (d, J = 7.1 Hz, 3H); 13C NMR (100 MHz, CDCl3) δ 192.2, 171.9, 158.1, 136.0, 134.8, 125.6, 81.7, 77.8, 73.9, 43.2, 36.1, 35.3, 29.9, 29.7, 26.4, 23.1, 14.4, 14.0, 13.9.
ACKNOWLEDGEMENTS
This work was financially supported by JSPS KAKENHI (No. 22404006) and the Global COE Program for Chemistry Innovation, the University of Tokyo. J.L.C.W. acknowledges funding from NOAA-ECOHAB (MML-106390A) and NCDHHS (01515-04).
References
1. Y.-Y. Lin, M. Risk, S. M. Ray, D. Van Engen, J. Clardy, J. Golik, J. C. James, and K. Nakanishi, J. Am. Chem. Soc., 1981, 103, 6773. CrossRef
2. Y. Shimizu, H. N. Chou, H. Bando, G. Van Duyne, and J. Clardy, J. Am. Chem. Soc., 1986, 108, 514. CrossRef
3. D. G. Baden, A. J. Bourdelais, H. Jacocks, S. Michelliza, and J. Naar, J. Environ. Health Perspect., 2005, 113, 621. CrossRef
4. A. J. Bourdelais, H. M. Jacocks, J. L. C. Wright, P. M. Jr. Bigwarfe, and D. G. Baden, J. Nat. Prod., 2005, 68, 2; CrossRef H. Fuwa, M. Ebine, A. J. Bourdelais, D. G. Baden, and M. Sasaki, J. Am. Chem. Soc., 2006, 128, 16989. CrossRef
5. M. Satake, A. Campbell, R. M. Van Wagoner, A. J. Bourdelais, H. Jacocks, D. G. Baden, and J. L. C. Wright, J. Org. Chem., 2009, 74, 989; CrossRef T. Kuranaga, M. Satake, G. Baden, J. L. C. Wright, and K. Tachibana, Tetrahedron Lett., 2010, 51, 4673; CrossRef T. Kuranaga, N. Ohtani, R. Tsutsumi, D. G. Baden, J. L. C. Wright, M. Satake, and K. Tachibana, Org. Lett., 2011, 13, 696. CrossRef
6. L. T. Truxal, A. J. Bourdelais, H. Jacocks, W. M. Abraham, and D. G. Baden, J. Nat. Prod., 2010, 73, 536. CrossRef
7. M. Satake, A. J. Bourdelais, R. M. Van Wagoner, D. G. Baden, and J. L. C. Wright, Org. Lett., 2008, 10, 3465. CrossRef
8. T. Kuranaga, T. Shirai, D. G. Baden, J. L. C. Wright, M. Satake, and K. Tachibana, Org. Lett., 2009, 11, 217; CrossRef R. Tsutusmi, T. Kuranaga, J. L. C. Wright, D. G. Baden, E. Ito, M. Satake, and K. Tachibana, Tetrahedron, 2010, 66, 6775. CrossRef
9. R. M. Van Wagoner, M. Satake, A. J. Bourdelais, D. G. Baden, and J. L. C. Wright, J. Nat. Prod., 2010, 73, 1177. CrossRef
10. O. O. Fadeyi and C. W. Lindsley, Org. Lett., 2009, 11, 3950. CrossRef
11. A. K. Ghosh and J. Li, Org. Lett., 2009, 11, 4164. CrossRef
12. J. Lee and J. S. Panek, Org. Lett., 2009, 11, 4390. CrossRef
13. A. B. Smith, III, N. Kutsumura, and J. Potuzak, Tetrahedron Lett., 2011, 52, 2117. CrossRef
14. G. Sabitha, S. Nayak, M. Bhikshapathi, and J. S. Yadav, Org. Lett., 2011, 13, 382; CrossRef J. S. Yadav, A. Raju, K. Ravindar, and B. V. S. Reddy, Tetrahedron Lett., 2013, 54, 3227; CrossRef J. S. Yadav, N. M. Reddy, Md. A. Rahman, A. R. Prasad, and B. V. S. Reddy, Tetrahedron, 2013, 69, 8618. CrossRef
15. A. T. Herrmann, S. R. Martinez, and A. Zakarian, Org. Lett., 2011, 13, 3636. CrossRef
16. G. Kumaraswamy, A. N. Murthy, V. Narayanarao, S. P. B. Vemulapalli, and J. Bharatam, Org. Biomol. Chem., 2013, 11, 6751. CrossRef
17. M. S. Lee, D. J. Repeta, K. Nakanishi, and M. G. Zagorski, J. Am. Chem. Soc., 1986, 108, 7855; CrossRef H.-N. Chou and Y. Shimizu, J. Am. Chem. Soc., 1987, 109, 2184. CrossRef
18. M. Yamazaki, K. Tachibana, and M. Satake, Tetrahedron, 2011, 67, 877. CrossRef
19. K. Nakanishi, Toxicon, 1985, 23, 473. CrossRef
20. M. Yamazaki, M. Izumikawa, K. Tachibana, M. Satake, Y. Itoh, and M. Hashimoto, J. Org. Chem., 2012, 77, 4902. CrossRef
21. T. Shirai, T. Kuranaga, J. L. C. Wright, D. G. Baden, M. Satake, and K. Tachibana, Tetrahedron Lett., 2010, 51, 1394. CrossRef
22. A. Suzuki and N. Miyaura, Chem. Rev., 1995, 95, 2457. CrossRef
23. T. Katsuki and K. B. Sharpless, J. Am. Chem. Soc., 1980, 102, 5974. CrossRef
24. G. Seidel and A. Fürstner, Chem. Comm., 2012, 48, 2055. CrossRef
25. J. E. Baldwin, J. Chem. Soc. Chem. Com., 1976, 734. CrossRef
26. J. A. Byers and T. F. Jamison, Proc. Natl. Acad. Sci. USA, 2013, 110, 16724. CrossRef