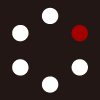
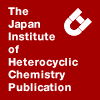
HETEROCYCLES
An International Journal for Reviews and Communications in Heterocyclic ChemistryWeb Edition ISSN: 1881-0942
Published online by The Japan Institute of Heterocyclic Chemistry
e-Journal
Full Text HTML
Received, 18th November, 2013, Accepted, 13th December, 2013, Published online, 6th January, 2014.
DOI: 10.3987/COM-13-12890
■ Simple Synthesis of New 3-Substituted 4-(3-Chloro-4-fluorophenyl)-1H-pyrrole Derivatives and Their Anticancer Activity in Vitro
Lan Lan, Xiaoping Zhan, Weixi Qin, Zenglu Liu, and Zhenmin Mao*
School of Pharmacy, Shanghai Jiaotong University , No.800 Dongchuan Road, Minhang District, Shanghai 200240, China
Abstract
The preparation of the new 3-substituted 4-(3-chloro-4-fluorophenyl)-1H-pyrrole derivatives was based on the Van Leusen pyrrole synthesis. In this article, eighteen compounds were readily synthesized in satisfactory to good yields and their cell proliferation inhibiting activities against 2 normal cell lines and 16 cancer cell lines were evaluated. It was particularly important that the new pyrroles displayed barely cytotoxicity against the tested normal cell, which meant these pyrrole analogues might show excellent selectivity towards cancer cell and normal cell. Cell cycle analysis by flow cytometry (FCM) showed that the G0/G1 phase decreased a lot and S phase arrested, in between a minor and transient G2/M block was observed. Cell death induced by 3-cyano-4-(3-chloro-4-fluorophenyl)-1H-pyrrole (2r) was also verified by multiple methods.INTRODUCTION
Pyrrole chemistry, which is developed with the isolation of substituted pyrroles from the degradation of various natural products, such as tremendous range of bacterial secondary metabolites,1-4 marine extracts,5,6 vital pigments of hemoglobin and chlorophyll,7 is drawing huge and highly specialized attention all around the world. Pyrrole moiety composes a series of compounds with potential biological significance. The past few decades have witnessed the appearance of a great number of single or multi substituted pyrrole derivatives. However, the anticancer activity of 3, 4-disubstituted pyrroles has not yet been reported, which means new exploit of this structural analogues might have been found. Our intention here is to discuss the convenient synthesis of new 3-substituted 4-(3-chloro-4-fluorophenyl)-1H-pyrrole derivatives, evaluate their anticancer activities in vitro and reveal the interconnection among different 3-substituents and the anticancer potency of these pyrroles with 4-pyrrole substituent unchanged. These pyrrole derivatives act as the model compounds to explain the impact of 3-substituents to the biological performance.
Many methodologys of affording pyrrole moiety have been explored, and Van Leusen pyrrole synthesis is one of the most effective and straightforward methods of preparation of 3, 4-disubstituted pyrroles. In this article, eighteen pyrrole derivatives were prepared steadily and their cell proliferation inhibiting activities against 2 normal cell lines (HUVEC and NIH/3T3 cell lines) and 16 cancer cell lines (including SGC-7901, MGC80-3, NCI-H460, L1210, DU 145, MCF-7, Hela, A375, K-562, HCT 116, HCT-15, CT-26, CHO, Hep G2, MG-63 and A549 cell lines) were determined by MTT assay with Paclitaxel as the positive control. It was found that 3-cyano-4-(3-chloro-4-fluorophenyl)-1H-pyrrole (2r) showed similar cytotoxicity against MGC80-3, CHO, A375 and Hela compared with Paclitaxel among these pyrroles. Then 2r was employed as the candidate compound, and cell lines of MGC80-3 and CHO were adopted for further biological study. The impact of 2r on cell cycle was testified. Meanwhile, cell death induced by 2r was also investigated by FCM (Annexin V-FITC/propidium iodide (PI) staining) and Hoechst 33342/PI double staining experiment. The intracellular environment of the apoptotic and necrotic CHO cells was observed by transmission electron microscope (TEM) before and after being treated 5µM 2r for 24 h.
RESULTS AND DISCUSSION
1.1 CHEMICAL
A simple synthesis of 3, 4-disubstituted pyrroles utilizing Van Leusen pyrrole methodology had been accomplished with good yields. The general preparation procedure was the same and was established as shown in Scheme 1. As one of the most effective and straightforward methods of preparing 3, 4-disubstituted pyrroles, it provide the possibility of getting 3, 4-bulky group-disubstituted pyrrole derivatives with α-H of pyrrole moiety free. We could see that α,β-unsaturated alkenes (1) were necessary for the cyclization reaction as the Michael acceptor. The synthesis of 1 varied as the 3-pyrrole substituent changed. Three chemical diversities (Entry a, b and c shown as below) of these pyrrole analogues were discussed as below.
1.1.1 Synthesis of 3-aroyl/acetyl-4-(3-chloro-4-fluorophenyl)-1H-pyrrole derivatives (Entry a and Entry b)
An equivalent molar amount of 3-chloro-4-fluorobenzaldehyde and acetyl aromatic compounds with various substituent groups were served as the useful starting materials to give corresponding intermediates 1 in 60-90% yield, which was conducted by the conventional base-catalyzed Claisen-Schmidt reaction. Two methods (method A and method B) were explored in Scheme 2. Claisen-Schmidt reaction was always carried out in aqueous medium with alcohols (method B). However, the same reaction in pure alcohols with a catalytic amount of sodium alkoxide (method A) showed slightly higher yield and shorter reaction time, which was due to better solubility of the reactants and lower moisture content. In method A, fresh prepared sodium alkoxide was used as the catalyst and methanol used as the only solvent, while in method B, sodium hydroxide and the mixture of methanol/water was served as the catalyst and co-solvent respectively. As the reactivity of acetylpyridine was much higher than the other acetophenone analogs and acetylthiophene, it was important to point out that acetylpyridine were added dropwise to make sure the excess of aromatic aldehydes to avoid the intermolecular self-condensation of acetylthiophene, and also the reaction time was much shorter. By the base-induced reaction of 1 and tosylmethyl isocyanide (TosMIC), seventeen 3-aroyl/acetyl-4-(3-chloro-4-fluorophenyl)-1H-pyrrole compounds were obtained effectively in 2 h at room temperature as shown in Table 1.
1.1.2 Synthesis of 3-ethoxycarbonyl/cyano-4-(3-chloro-4-fluorophenyl)-1H-pyrrole derivatives (Entry c)
It’s reported that the direct coupling of corresponding aldehyde and EtOAc/acetonitrile could only take place in strong alkaline condition (such as n-BuLi/LDA),8-11 inert atmosphere reflux environment,12,13 or by the usage of special pretreated composite alkaline14 and particular catalyst.11,15-17 Electrochemical methods18,19 were also found to be feasible. We believed that the Horner-Wadsworth-Emmons (HWE) olefination using triethylphosphite as the carbanion-stabilizing group had definite advantages over the conventional methods by avoiding high temperature and the usage of hazardous chemicals. The HWE reagent whose carbanion center was immediately adjacent to a P(V) center were remarkably versatile and adopted a wide range of coordination modes.20
The HWE reagent was a variant Wittig-type reagent which possesses plenty of significant advantages.21-23 The reaction with aldehydes and ketones could be carried out under mild conditions as a result of the good nucleophilic capability of phosphate carbanion. Besides, the byproduct was water-soluble phosphates, which make the separation of alkenes 1 easier. Another focus was that the HWE reagents were readily available and their remarkable stabilities to alkali and air. Ethyl chloroacetate and chloroacetonitrile reacted respectively with triethylphosphite under 150 °C to give the corresponding HWE reagents. The purification was conducted by distillation under reduced pressure in 90-95% yield, the condensation with aromatic aldehydes hereafter to afford the alkenes (1). The carbanion (3) is initiated after the deprotonation of the α-carbon by NaH or t-BuOK, with the crucial step being a simultaneous C-P and C-O bond cleavage in an intermediately formed oxaphosphetane (4) to afford the desired alkene and the phosphate byproduct in the inverse-[2+2] cycloaddition way seen in Scheme 3. The existence of –CO2Et and -CN accelerates the fracture of the carbon–phosphorus bond. The cyclization of pyrrole ring system was followed by the olefination, which was consistent with Scheme 1. Two compounds were generated conveniently and displayed in Table 2.
1.2 ANTICANCER ACTIVITY IN VITRO
1.2.1 Cytotoxicity studies
The quest for new chemical structures with selective anticancer activity properties without hurting normal tissue cell was a new emphasis in the last few years when exploring new anticancer agents. 3, 4-Disubstituted pyrroles were first developed as the candidates of antifungal agents.24-26 The anticancer activity and anticancer spectrum were never reported. Here new 3-substituted 4-(3-chloro-4-fluorophenyl)-1H-pyrrole derivatives and their in vitro cell proliferation inhibiting activity was evaluated using the standard 3-(4,5-dimethylthiazol-2-yl)-2,5-diphenyltetrazolium bromide (MTT) analysis against 2 normal cell lines and 16 cancer cell lines with Paclitaxel as the reference control. The anticancer potency of these 3,4-disubstituted pyrrole compounds was evaluated by IC50 values that were calculated by linear regression analysis of the concentration-response curve. IC50 for partial of the cell lines were charted in Table 3.
The cytotoxicity of the eighteen pyrrole derivatives revealed that the pyrroles were less active than, or comparative to the positive control Paclitaxel. For example, 2e, 2f, 2n, 2o and 2r showed good inhibiting ability (IC50<10.0 µM) against MGC80-3 cell line (the values of IC50 = 8.4, 9.0, 9.6, 8.0 and 4.5 µM respectively, mean values were shown), 2f, 2g and 2r displayed good inhibiting ability against A375 (the values of IC50 were 6.7, 6.4 and 6.1 µM respectively); 2r also showed better bioactivity against CHO (IC50 = 3.9 µM) then other pyrrole derivatives. It was also delightful to find that the inhibiting activity of 2c and 2h against HTC-15 (IC50 = 9.5 µM) and MCF-7 (IC50 = 8.7 µM) were also satisfying (data not shown). Although Paclitaxel showed better cytotoxicity towards most of these cancer cell lines, no selectivity was performed between the tested cancer cell lines and normal cell lines. Attention should be drawn to several aspects that these pyrroles analogues were of smaller molecular with more flexible reaction sites. Preliminary structure-activity relationship was summarized according to the cell proliferation inhibiting activity. In general, the cytotoxicity was sequenced as 3-cyanopyrrole (2r) > 3-benzoylpyrroles > 3-ethoxycarbonylpyrrole (2q) > 3-thiophenecarbonylpyrrole (2p) > 3- pyridinecarbonylpyrroles (2l and 2m). All of these structurally very dissimilar compounds contained aryl rings and electron donor and H-bond donor/acceptor functions. It was found that the cytotoxicity of 3-heterocycle carbonyl substituted pyrroles (2l, 2m and 2p) exhibited little impact to the tested cancer cell lines. It was interesting to point out that compounds 2l and 2m only showed limited cytotoxicity against A375 with the IC50 values were 32.3 and 24.0 µM respectively.
Compared with compound 2a, for the 3-benzoyl-4-(3-chloro-4-fluorophenyl)-1H-pyrroles (Entry a and b), the electron withdrawing group (EWG) substituted compounds at R3 and R1 showed more potent cancer cell inhibition compared with other R2 substituent. Good examples were illustrated that compound 2e was approximately 5-fold more active than 2d, and 2j was 3-fold better than 2k against MGC80-3 and CHO. Hence, we presumed that the cytotoxicity would increase as the electron density of pyrrole moiety decreased. In further studies, the introduction of -CO2Et and -CN at 3-position of pyrrole moiety was to lower the electron density of pyrrole moiety. It could be concluded from Table 3 that 3-CO2Et did not affected the anticancer activity significantly. Thus we presumed that the connection between the cytotoxicity and electron density of pyrrole moiety was not simply dependent, maybe there was a balanced interplay between the electron density of pyrrole moiety and steric bulk effect of 3-position substituent. This assumption was consistent with our findings on the 3-bulk substituted pyrroles 2n and 2o which exhibited much better bioactivity against MGC80-3 and Hela than most of the other compounds. We suggested that particular spatial effect of 3-substitution might be of great importance.
After discussing the influence of various substitutions on the cytotoxicity of the 3-substituted 4-(3-chloro-4-fluorophenyl)-1H-pyrrole derivatives, Log P was brought into consideration. Although the obvious connection of Log P and anticancer activities was not reported, the compounds possessing appropriate Log P values would be helpful to enhance the anticancer activities.
In summary, as a class of small molecule compounds, the new pyrrole derivatives showed specialized cytotoxicity against the tested cancer cells and excellent safety towards normal cells. The electron-withdrawing ability and steric hindrance of 3-position threw great influence on the cytotoxicity.
1.2.2 Cell cycle analysis
In the study assessing the efficacy of the pyrroles against 16 cancer cell lines, 2r showed the strongest anticancer potency against MGC80-3 and CHO. However, the mechanisms of action remained to be unrevealed. The cell cycle progression was determined by FCM (BD FACSCalibur, America) using staining of PI. The percentages of G0/G1, G2/M and S phase at three concentrations of 1.0 µM, 3.0 µM and 9.0 µM were demonstrated in Figure 1.
DNA biosyntheses often fluctuate with the state of the cell in the cell cycle. The cell cycle analysis in Figure 1 showed that 2r would obviously decrease the proportion of G0/G1 phase, and cause S phase arrest along with a minor and transient G2/M block. Approximately the proportion of G0/G1 phase for MGC80-3 and CHO were lowered by nearly 50%, that is from 41.01% to 22.58% for CHO cells (mean value was given) after a 9.0 µM 2r treatment for 24 h. One possible reason for the S phase accumulation could be that 2r-induced DNA damage was irreparable leaving the cells in S phase, which was later removed by the cytotoxic effect of the pyrroles compound; or the mass synthesis of DNA was suppressed directly by 2r, which leaded to the cell cycle irregulation. As a result, we believed that S phase accumulation would shut down the entrance to the G2/M phase, thereby blocked the cell growth and division, and eventually caused an inhibition of tumor growth. Further information for drug onset mechanism was not yet proven.
1.2.3 Cell death analysis by FCM
Annexin V-FITC/PI double staining was used so as to prove whether the cell death of CHO induced by 2r was related to cell apoptosis or cell necrosis. Staining with Annexin V-FITC was typically used in conjunction with PI by FCM to identify early cell apoptosis (Q1), end cell apoptosis or cell necrosis (Q2) and necrotic cell (Q3). In Figure 2 the percentages of each cell status were showed after being treated 0, 1.0, 3.0 and 9.0 µM of 2r for 12 h. The results indicated that the proportion of necrotic cell was 4-fold more than control group and the proportion of Q2 was 5-fold increased after being treated with 9.0 µM 2r for 12 h, but the early cell apoptosis did not change significantly.
1.2.4 Cell death analysis by Hoechst 33342/PI double staining
Staining living cells with Hoechst 33342 and PI and observation under fluorescence microscope was a simple and valid method to quantify apoptotic cell and necrotic cell in cultures (MGC80-3 and CHO cells) exposed to varying concentrations of 2r. Both cell necrosis and cell apoptosis were observed, and necrotic effect was more prominent. The percentage of necrotic cell was remarkably increased from 0.99% to 11.60% in a concentration-dependent manner after 27.0 µM 2r treated for 12 h, while the proportion of apoptotic cell was elevated from 0.83% to 2.64%. The percentage of cell apoptosis and cell necrosis were given after treated 0, 1.0, 3.0, 9.0 and 27.0 µM of 2r in Figure 3 (mean values were given). The mechanism of 2r inhibiting the growth of MGC80-3 and CHO in vitro might include both apoptotic effect and necrotic effect. Both the Hoechst 33342/PI staining experiment and Annexin V-FITC/PI staining by flow cytometry double-confirmed that cell necrosis played an overwhelming part in causing cell death accompanying by cell apoptosis (Figures 2 and 3).
1.2.5 Cellular substructure observed by TEM
Transmission electron microscope (TEM) is a powerful instrument to observe the fine substructure inside of cells. The 2r-induced necrotic (F-1, 2 and 3 in Figure 4) and apoptotic (G-1, 2 and 3) cells were observed under TEM. More specifically, E-1, 2 and 3 displayed the cellular substructure of normal CHO cell in different magnifications:27-29 the normal cell took an integral spherical shape with large nucleus of clear double membrane structure, the nucleoli were prominent, cell contents (such as free ribosomes, endoplasmic reticulum and mitochondria) were scattered in cytoplasm and their microstructural stay intact. The necrotic cell (shown in F-1, 2 and 3) demonstrated a swelling cell with disintegrated membrane (cell and nuclear membrane) and increased necrotic substances. The enhancement of membrane permeability resulted in the rupture of the plasma membrane and the lysis of the intracellular contents in to the extracellular milieu. The mitochondria and endoplasmic reticulum were also swollen and severely damaged. The nucleoli in apoptotic cell (G-1, 2 and 3, which shrank) disappeared, with chromatin condensed and marginated. The cell membrane surrounded and split the cytoplasm into apoptotic bodies and other vesicular bodies of bilayer or multilayer structure, but the membrane system was of good integrity. The differences between normal cell, necrotic cell and apoptotic cell were clear enough. The new 3, 4-disubstituted pyrroles possessed both the apoptosis and necrosis effect.
Here we described the design and synthesis of a novel series of 3-substituted 4-(3-chloro-4-fluorophenyl)-1H-pyrrole derivatives. 18 cell lines were tested using MTT assay to determine the cell proliferation inhibiting activity and anticancer spectrum. Those pyrroles were less active than, or comparative to the positive control Paclitaxel. Certain substituted pyrrole derivatives showed good cytotoxicity against A375, HCT-15, CT-26, H460, MGC80-3 and CHO. It was particularly important that the new pyrroles displayed barely cytotoxicity against the tested normal cell, which meant this pyrrole analogue might show excellent selectivity towards cancer cell and normal cell. The improvement of the electron-withdrawing ability of 3-substituent would contribute to the cytotoxicity to some extent, but the steric effect was also equally important. Among all these pyrrole analogues, 2r showed comparable cytotoxicity as Paclitaxel toward MGC80-3 (IC50 = 4.5 µM) and CHO (IC50 = 3.9 µM) after 24 h treatment. Cell cycle by FCM showed that the G0/G1 phase decreased a lot and S phase arrested, in between a minor and transient G2/M block was observed. Both the necrotic effect and apoptotic effect were detected by FCM and Hoechst 33342/PI double staining experiment. Accumulating evidence revealed that cell necrosis was the dominant factor in cell death induced by 2r and cell apoptosis was the concomitant occurring. The morphological features of necrotic and apoptotic cell was examined under TEM, which would be helpful to understand the action mechanism of the 3-substituted-4-(3-chloro-4-fluorophenyl)-(1H)-pyrrole derivatives. It was a disappointment to find that 3-heterocycle carbonyl substituted pyrroles (2l, 2m and 2p) were not as strong as expected against the tested cancer cell lines. More potent anticancer agents of 3, 4-disubstituted pyrrole moiety structure with optimized substituents awaited to be developed.
EXPERIMENTAL
2.1 CHEMISTRY
All chemicals and solvents were purchased from commercial supplies and all reagents used in current study were of analytical grade. 3-chloro-4-fluorobenzaldehyde, nitromethane (MeNO2), tetrahydrofuran (THF) and methanol were purified by standard method and dried before use. All reactions involving air or moisture sensitive or intermediates were carried out under nitrogen. Thin layer chromatography (TLC) was performed on silica gel 60 F254-plate with fluorescent indicator. Column chromatography was carried out on 200-300 mesh silica gel or neutral aluminum oxide. All the 1H NMR and 13C NMR spectra were recorded on Bruker AVANCE III 400 model NMR Spectrometer in DMSO-d6, and chemical shifts (δ) were recorded as parts per million (ppm). Melting points were determined on microscopic melting point apparatus (SGW X-4, Shanghai Precision & Scientific Instrument Co., Ltd.) and were uncorrected.
2.1.1 General procedure for Van Leusen pyrrole moiety synthesis
All the Van Leusen pyrrole synthesis was completed in THF (20 mL) solution of TosMIC (1.1 eq.), t-BuOK (1.2 eq.) and corresponding α,β-unsaturated alkenes at rt for 1 h under TLC monitoring. Ice water (50 mL) was poured into the mixture, removed THF under vacuum and the solution was then extracted by EtOAc (75 mL, 25 mL × 3), which was later washed with saturated brine and dried over anhydrous Na2SO4. The crude pyrroles product was purified by aluminum oxide column chromatography with EtOAc/hexanes as eluents to afford the 3-substituted 4-(3-chloro-4-fluorophenyl)-1H-pyrroles.
2.1.2. General procedure for the synthesis of pyrroles (2a-2p)
Finely cut Na (0.01g, 0.4 mmol) was added into dry MeOH (15 mL) with ice bath till no gas generated to make the fresh-prepared MeONa/MeOH solution, which was used only after cooling. 3-chloro-4-fluorobenzaldehyde (1.58 g, 10.0 mmol) was added to the solution of fresh prepared MeONa/MeOH (15 mL), soon after an equivalent amount of acetyl aromatic compounds was gradually added dropwise to the mixture with constant stirring for 2-8 h (36 h for 2n and 2o due to the steric hindrance of the side chain while 15 min for 2l and 2m due to the highly activity of acetylpyridine). The end of the reaction was indicated by TLC. Water (20 mL) and EtOAc (75 mL, 25 mL × 3) were added into the reaction mixture. The inorganic precipitate was removed, and the organic solution was washed with brine for 3 times and dried. After being evaporated, the crude product was chromatographed on silica gel with EtOAc/hexanes as eluent to give pure α,β-unsaturated alkenes (1). Van Leusen pyrrole synthesis was then conducted (see 2.1.1.).
[4-(3-Chloro-4-fluorophenyl)-1H-pyrrole-3-yl](phenyl)methanone (2a)
White solid, 1H NMR (400 MHz, DMSO-d6) δ (ppm): 11.74 (s, 1H, pyrrole-NH), 7.75 (d, J = 8.0 Hz, 1H, ArH), 7.61 (dd, J1 = 4.0 Hz, J2 = 2.0 Hz, 1H, ArH), 7.58 (d, J = 8.0 Hz, 2H, ArH), 7.48 (t, J = 8.0 Hz, 2H, ArH), 7.39 (m, 1H, ArH), 7.30 (t, J = 8.0 Hz, 1H, ArH), 7.26 (t, J = 4.0 Hz, 1H, pyrrole H), 7.19 (t, J = 4.0 Hz, 1H, pyrrole H); 13C NMR (100 MHz, DMSO-d6) δ (ppm): 190.20, 155.61 JCF = 245.1 Hz, 139.89, 133.12 JCF = 4.0 Hz, 131.52, 129.95, 128.86 (2C), 128.79 JCF = 7.0 Hz, 128.68, 128.14 (2C), 123.03, 120.41, 120.34, 118.51 JCF = 17.6 Hz, 115.99 JCF = 20.7 Hz. ESI-HRMS m/z: calcd for C17H11ClFNO ([M+H]): 300.0593, 302.0563; found: 300.0491, 302.0488.
[4-(3-Chloro-4-fluorophenyl)-1H-pyrrole-3-yl](2-chlorophenyl)methanone (2b)
White solid, 1H NMR (400 MHz, DMSO-d6) δ (ppm): 11.82 (s, 1H, pyrrole-NH), 7.70 (dd, J1 = 7.2 Hz, J2 = 2.0 Hz, 1H, ArH), 7.55-7,45 (m, 4H, ArH), 7.41 (dd, J1 = 7.2 Hz, J2 = 2.0 Hz, 1H, ArH), 7.35 (t, J = 8.0 Hz, 1H, ArH), 7.16 (t, J = 4.0 Hz, 1H, pyrrole H), 7.03 (dd, J1 = 7.2 Hz, J2 = 2.0 Hz, 1H, pyrrole H); 13C NMR (100 MHz, DMSO-d6) δ (ppm): 188.48, 155.81 JCF = 245.2 Hz, 140.46, 132.61 JCF = 3.8 Hz, 130.63, 130.60, 130.26, 129.53 (2C), 129.05 JCF = 7.1 Hz, 128.58, 126.80, 122.65, 121.28, 120.92, 118.46 JCF = 17.6 Hz, 115.95 JCF = 20.6 Hz. ESI-HRMS m/z: calcd for C17H10Cl2FNO ([M+H]): 334.0203, 336.0173; found: 334.0122, 336.0095.
[4-(3-Chloro-4-fluorophenyl)-1H-pyrrole-3-yl](4-chlorophenyl)methanone (2c)
White solid, 1H NMR (400 MHz, DMSO-d6) δ (ppm): 11.80 (s, 1H, pyrrole-NH), 7.75 (d, J = 8.0 Hz, 2H, ArH), 7.60 (dd, J1 = 7.2 Hz, J2 = 2.0 Hz, 1H, ArH), 7.54 (d, J = 8.0 Hz, 2H, ArH), 7.39 (m, 1H, ArH), 7.34-7.28 (m, 2H, ArH + pyrrole H), 7.20 (t, J = 4.0 Hz, 1H, pyrrole H); 13C NMR (100 MHz, DMSO-d6) δ (ppm): 188.91, 155.66 JCF = 245.0 Hz, 138.56, 136.32, 132.97 JCF = 3.8 Hz, 130.72 (2C), 129.98, 128.92, 128.82 JCF = 7.0 Hz, 128.23 (2C), 123.05, 120.58, 120.07, 118.54 JCF = 17.6 Hz, 116.01 JCF = 20.6 Hz. ESI-HRMS m/z: calcd for C17H10Cl2FNO ([M+H]): 334.0203, 336.0173; found: 334.0098, 336.0092.
[4-(3-Chloro-4-fluorophenyl)-1H-pyrrole-3-yl](2-fluorophenyl)methanone (2d)
White solid, 1H NMR (400 MHz, DMSO-d6) δ (ppm): 11.82 (s, 1H, pyrrole-NH), 7.67 (dd, J1 = 8.0 Hz, J2 = 2.0 Hz, 1H, ArH), 7.57-7.44 (m, 3H, ArH), 7.33 (t, J = 8.0 Hz, 1H, ArH), 7.29 (d, J = 8.0 Hz, 1H, ArH), 7.26 (d, J = 8.0 Hz, 1H, ArH), 7.20 (t, J = 4.0 Hz, 1H, pyrrole H), 7.16 (t, J = 4.0 Hz, 1H, pyrrole H); 13C NMR (100 MHz, DMSO-d6) δ (ppm): 186.50, 158.76 JCF = 247.6 Hz, 155.77 JCF = 245.2 Hz, 132.68 JCF = 3.8 Hz, 131.95 JCF = 8.2 Hz, 130.26, 130.22, 129.72 JCF = 3.3 Hz, 129.30 JCF = 15.9 Hz, 129.06 JCF = 7.1 Hz, 124.15 JCF = 3.5 Hz, 122.67, 121.37, 121.02, 118.45 JCF = 17.7 Hz, 116.00 JCF = 5.4 Hz, 115.79 JCF = 6.4 Hz. ESI-HRMS m/z: calcd for C17H10ClF2NO ([M+H]): 318.0498, 320.0469; found: 318.0401, 320.0395.
[4-(3-Chloro-4-fluorophenyl)-1H-pyrrole-3-yl](4-fluorophenyl)methanone (2e)
White solid, 1H NMR (400 MHz, DMSO-d6) δ (ppm): 11.77 (s, 1H, pyrrole-NH), 7.81 (dd, J1 = 12.0 Hz, J2 = 8.0 Hz, 2H, ArH), 7.59 (dd, J1 = 8.0 Hz, J2 = 4.0 Hz, 2H, ArH), 7.39 (m, 1H, ArH), 7.33 (d, J = 4.0 Hz, 1H, ArH), 7.31 (d, J = 4.0 Hz, 1H, ArH), 7.29 (dd, J1 = 4.0 Hz, J2 = 2.0 Hz, 1H, pyrrole H), 7.19 (t, J = 4.0 Hz, 1H, pyrrole H); 13C NMR (100 MHz, DMSO-d6) δ (ppm): 188.78, 164.05 JCF = 249.4 Hz, 155.62 JCF = 245.0 Hz, 136.39 JCF = 3.0 Hz, 133.04 JCF = 3.8 Hz, 131.60 (2C, JCF = 9.1 Hz), 129.93, 128.78 JCF = 7.0 Hz, 128.57, 123.03, 120.44, 120.21, 118.54 JCF = 17.6 Hz, 116.02 JCF = 20.8 Hz, 115.09 (2C, JCF = 21.8 Hz). ESI-HRMS m/z: calcd for C17H10ClF2NO ([M+H]): 318.0498, 320.0469; found: 318.0374, 320.0359.
[4-(3-Chloro-4-fluorophenyl)-1H-pyrrole-3-yl](4-methoxyphenyl)methanone (2f)
White solid, 1H NMR (400 MHz, DMSO-d6) δ (ppm): 11.69 (s, 1H, pyrrole-NH), 7.77 (d, J = 8.0 Hz, 2H, ArH), 7.58 (dd, J1 = 8.0 Hz, J2 = 4.0 Hz, 1H, ArH), 7.36 (m, 1H, ArH), 7.30 (t, J = 8.0 Hz, 1H, ArH), 7.25 (dd, J1 = 4.0 Hz, J2 = 2.0 Hz, 1H, pyrrole H), 7.19 (t, J = 4.0 Hz, 1H, pyrrole H), 7.03 (d, J = 8.0 Hz, 2H, ArH), 3.84 (s, 3H, PhOCH3); 13C NMR (100 MHz, DMSO-d6) δ (ppm): 189.07, 162.15, 155.52 JCF = 244.8 Hz, 133.25 JCF = 3.9 Hz, 132.23, 131.25 (2C), 129.73, 128.61 JCF = 7.0 Hz, 127.51, 122.88, 120.52, 120.04, 118.54 JCF = 17.5 Hz, 116.04 JCF = 20.7 Hz, 113.44 (2C), 55.38. ESI-HRMS m/z: calcd for C18H13ClFNO2 ([M+H]): 330.0698, 332.0669; found: 330.0599, 332.0600.
[4-(3-Chloro-4-fluorophenyl)-1H-pyrrole-3-yl](3-methoxyphenyl)methanone (2g)
White solid, 1H NMR (400 MHz, DMSO-d6) δ (ppm): 11.75 (s, 1H, pyrrole-NH), 7.60 (dd, J1 = 8.0 Hz, J2 = 4.0 Hz, 1H, ArH), 7.45-7.35 (m, 2H, ArH), 7.35-7.25 (m, 3H, ArH), 7.23 (dd, J1 = 4.0 Hz, J2 = 2.0 Hz, 1H, pyrrole H), 7.18 (t, J = 4.0 Hz, 1H, pyrrole H), 7.14 (m, 1H, ArH), 3.79 (s, 3H, PhOCH3); 13C NMR (100 MHz, DMSO-d6) δ (ppm): 189.86, 158.92, 155.61 JCF = 244.8 Hz, 141.29, 133.12 JCF = 3.9 Hz, 129.96, 129.25, 128.78 JCF = 7.2 Hz, 128.68, 123.04, 121.34, 120.41, 120.32, 118.50 JCF = 17.6 Hz, 117.53, 115.98 JCF = 20.7 Hz, 113.58, 55.16. ESI-HRMS m/z: calcd for C18H13ClFNO2 ([M+H]): 330.0698, 332.0669; found: 330.0610, 332.0617.
[4-(3-Chloro-4-fluorophenyl)-1H-pyrrole-3-yl](4-methylphenyl)methanone (2h)
White solid, 1H NMR (400 MHz, DMSO-d6) δ (ppm): 11.71 (s, 1H, pyrrole-NH), 7.67 (d, J = 8.0 Hz, 2H, ArH), 7.60 (dd, J1 = 8.0 Hz, J2 = 2.0 Hz, 1H, ArH), 7.38 (m, 1H, ArH), 7.33 (d, J = 8.0 Hz, 2H, ArH), 7.29 (s, 1H, ArH), 7.25 (dd, J1 = 4.0 Hz, J2 = 2.0 Hz, 1H, pyrrole H), 7.19 (t, J = 4.0 Hz, 1H, pyrrole H), 2.39 (s, 3H, PhCH3); 13C NMR (100 MHz, DMSO-d6) δ (ppm): 189.91, 155.58 JCF = 244.9 Hz, 141.68, 137.16, 133.19 JCF = 3.6 Hz, 129.85, 129.10 (2C), 128.75, 128.70 (2C), 128.24, 122.96, 120.43, 120.27, 118.52 JCF = 17.5 Hz, 116.01 JCF = 20.7 Hz, 21.00. ESI-HRMS m/z: calcd for C18H13ClFNO ([M+H]): 314.0749, 316.0719; found: 314.0577, 316.0601.
[4-(3-Chloro-4-fluorophenyl)-1H-pyrrole-3-yl](2,4-dimethylphenyl)methanone (2i)
White solid, 1H NMR (400 MHz, DMSO-d6) δ (ppm): 11.69 (s, 1H, pyrrole-NH), 7.65 (dd, J1 = 8.0 Hz, J2 = 4.0 Hz, 1H, ArH), 7.47 (m, 1H, ArH), 7.33 (t, J = 8.0 Hz, 1H, ArH), 7.27 (d, J = 8.0 Hz, 1H, ArH), 7.14 (t, J = 4.0 Hz, 1H, pyrrole H), 7.08 (s, 1H, ArH), 7.04 (d, J = 8.0 Hz, 1H, ArH), 7.69 (dd, J1 = 4.0 Hz, J2 = 2.0 Hz, 1H, pyrrole H), 2.31(s, 3H, -phCH3), 2.23 (s, 3H, PhCH3); 13C NMR (100 MHz, DMSO-d6) δ (ppm): 192.18, 115.68 JCF = 244.9 Hz, 138.84, 138.16, 135.26, 133.01 JCF = 3.8 Hz, 131.12, 130.13, 129.61, 128.93 JCF = 7.0 Hz, 128.02, 125.46, 122.72, 121.91, 120.72, 118.40 JCF = 17.6 Hz, 115.88 JCF = 20.7 Hz, 20.78, 19.31. ESI-HRMS m/z: calcd for C19H15ClFNO ([M+H]): 328.0906, 330.0876; found: 328.0654, 330.0530.
[4-(3-Chloro-4-fluorophenyl)-1H-pyrrole-3-yl](3-bromophenyl)methanone (2j)
Pale yellow solid, 1H NMR (400 MHz, DMSO-d6) δ (ppm): 11.81 (s, 1H, pyrrole-NH), 7.80 (t, J = 4.0 Hz, 1H, ArH), 7.78 (d, J = 8.0 Hz, 1H, ArH), 7.72 (dt, J1 = 8.0 Hz, J2 = 2.0 Hz, 1H, ArH), 7.60 (dd, J1 = 8.0 Hz, J2 = 2.0 Hz, 1H, ArH), 7.44 (t, J = 8.0 Hz, 1H, ArH), 7.38 (m, 1H, ArH), 7.32 (m, 1H, pyrrole H), 7.31 (t, J = 12.0 Hz, 1H, ArH), 7.19 t, J = 4.0 Hz, 1H, pyrrole H); 13C NMR (100 MHz, DMSO-d6) δ (ppm): 188.52, 155.67 JCF = 245.2 Hz, 142.02, 134.06, 132.91 JCF = 3.9 Hz, 131.29, 130.38, 130.05, 129.10, 128.85 JCF = 7.0 Hz, 127.80, 123.10, 121.46, 120.63, 120.01, 118.57 JCF = 17.6 Hz 116.00 JCF = 20.8 Hz. ESI-HRMS m/z: calcd for C17H10BrClFNO ([M+H]): 377.9698, 379.9677; found: 377.9492, 379.9458.
[4-(3-Chloro-4-fluorophenyl)-1H-pyrrole-3-yl](4-bromophenyl)methanone (2k)
Pale yellow solid, 1H NMR (400 MHz, DMSO-d6) δ (ppm): 11.80 (s, 1H, pyrrole-NH), 7.68 (m, 4H, ArH), 7.60 (dd, J1 = 8.0 Hz, J2 = 4.0 Hz, 1H, ArH), 7.39 (m, 1H, ArH), 7.34 (d, J = 12.0 Hz, 1H, ArH), 7.30 (dd, J1 = 4.0 Hz, J2 = 2.0 Hz, 1H, pyrrole H), 7.20 (t, J = 4.0 Hz, 1H, pyrrole H); 13C NMR (100 MHz, DMSO-d6) δ (ppm): 189.05, 155.66 JCF = 245.0 Hz, 138.91, 132.97 JCF = 3.7 Hz, 131.18 (2C), 130.89 (2C), 129.99, 128.98, 128.83 JCF = 7.0 Hz, 125.29, 123.05, 120.60, 120.04, 118.54 JCF = 17.7 Hz, 116.01 JCF = 20.7 Hz. ESI-HRMS m/z: calcd for C17H10BrClFNO ([M+H]): 377.9698, 379.9677; found: 377.9505, 379.9479.
[4-(3-Chloro-4-fluorophenyl)-1H-pyrrole-3-yl](2-pyridinyl)methanone (2l)
Pale yellow solid, 1H NMR (400 MHz, DMSO-d6) δ (ppm): 11.76 (s, 1H, pyrrole-NH), 8.67 (d, J = 4.0 Hz, 1H, pyridine H), 7.97 (t, J = 8.0 Hz, 1H, pyridine H), 7.90-7.82 (m, 2H), 7.62 (dd, J1 = 8.0 Hz, J2 = 4.0 Hz, 1H), 7.58 (dd, J1 = 8.0 Hz, J2 = 4.0 Hz, 1H), 7.42 (m, 1H, pyrrole H), 7.33 (t, J = 8.0 Hz, 1H, PhH), 7.12 (s, 1H); 13C NMR (100 MHz, DMSO-d6) δ (ppm): 187.27, 156.45,155.68 JCF = 245.07 Hz, 148.28, 137.16, 133.45 JCF = 3.8 Hz, 131.00, 130.29, 129.14 JCF = 7.1 Hz, 125.86, 123.61, 123.07, 120.11, 118.84, 118.33 JCF = 17.5 Hz, 115.83 JCF = 20.7 Hz. ESI-HRMS m/z: calcd for C16H10ClFN2O ([M+H]): 301.0545, 303.0516; found: 301.0365, 303.0402.
[4-(3-Chloro-4-fluorophenyl)-1H-pyrrole-3-yl](3-pyridinyl)methanone (2m)
Pale yellow solid, 1H NMR (400 MHz, DMSO-d6) δ (ppm): 11.86 (s, 1H, pyrrole-NH), 8.86 (d, J = 4.0 Hz, 1H, pyridine H), 8.74 (dd, J1 = 4.0 Hz, J2 = 2.0 Hz, 1H, pyridine H), 8.08 (dt, J1 = 8.0 Hz, J2 = 2.0 Hz, 1H, pyridine H), 7.65 (dd, J1 = 8.0 Hz, J2 = 2.0 Hz, 1H), 7.52 (dd, J1 = 8.0 Hz, J2 = 4.0 Hz, 1H), 7.42 (m, 1H), 7.37 (dd, J1 = 4.0 Hz, J2 = 2.0 Hz, 1H, pyrrole H), 7.31 (t, J = 8.0 Hz, 1H, PhH), 7.21 (t, J = 4.0 Hz, 1H, pyrrole H); 13C NMR (100 MHz, DMSO-d6) δ (ppm): 188.43, 155.70 JCF = 245.0 Hz, 151.82, 149.34, 136.27, 135.42, 132.87 JCF = 3.8 Hz, 130.13, 129.55, 128.96 JCF = 7.1 Hz, 123.38, 123.04, 120.83, 120.22, 118.54 JCF = 17.7 Hz, 116.01 JCF = 20.7 Hz. ESI-HRMS m/z: calcd for C16H10ClFN2O ([M+H]): 301.0545, 303.0516; found: 301.0396, 303.0381.
[4-(3-Chloro-4-fluorophenyl)-1H-pyrrole-3-yl](4-biphenyl)methanone (2n)
Yellow solid, 1H NMR (400 MHz, DMSO-d6) δ (ppm): 11.76 (s, 1H, pyrrole-NH), 7.86 (d, J = 8.0 Hz, 2H), 7.79 (d, J = 8.0 Hz, 2H), 7.75 (d, J = 8.0 Hz, 2H), 7.63 (dd, J1 = 8.0 Hz, J2 = 4.0 Hz, 1H), 7.51 (t, J = 8.0 Hz, 2H), 7.42 (t, J = 8.0 Hz, 2H), 7.34 (t, J = 4.0 Hz, 1H, pyrrole H), 7.32 (d, J = 8.0 Hz, 1H), 7.21 (t, J = 4.0 Hz, 1H, pyrrole H); 13C NMR (100 MHz, DMSO-d6) δ (ppm): 189.72, 155.63 JCF = 244.9 Hz, 143.12, 139.17, 138.67, 133.14 JCF=3.7 Hz, 129.95, 129.68 (2C), 129.03 (2C), 128.78 JCF=6.8 Hz, 128.59, 128.08, 126.84 (2C), 126.41 (2C), 123.05, 120.43, 120.40, 118.54 JCF=17.9 Hz, 116.02 JCF=20.7 Hz. ESI-HRMS m/z: calcd for C23H15ClFNO ([M+H]): 376.8306, 378.0876; found: 376.8007, 378.0656.
[4-(3-Chloro-4-fluorophenyl)-1H-pyrrole-3-yl](1-naphthyl)methanone (2o)
Pale yellow solid, 1H NMR (400 MHz, DMSO-d6) δ (ppm): 11.74 (s, 1H, pyrrole-NH), 8.10-7.95 (m, 3H), 7.72 (dd, J1 = 8.0 Hz, J2 = 2.0 Hz, 1H), 7.66 (dd, J1 = 8.0 Hz, J2 = 2.0 Hz, 1H), 7.60-7.45 (m, 4H), 7.32 (t, J = 8.0 Hz, 1H), 7.18 (t, J = 4.0 Hz, 1H, pyrrole H), 7.06 (dd, J1 = 4.0 Hz, J2 = 2.0 Hz, 1H); 13C NMR (100 MHz, DMSO-d6) δ (ppm): 191.49, 155.75 JCF = 245.0 Hz, 138.59, 133.15, 132.97 JCF = 3.8 Hz, 130.30, 130.26, 130.09, 129.88, 129.09 JCF = 7.1 Hz, 128.23, 126.73, 126.20, 126.15, 125.26, 124.63, 123.04, 122.31, 120.94, 118.43 JCF = 17.6 Hz, 115.87 JCF = 20.7 Hz. ESI-HRMS m/z: calcd for C21H13ClFNO ([M+H]): 350.0749, 352.0720; found: 350.0583, 352.0609.
[4-(3-Chloro-4-fluorophenyl)-1H-pyrrole-3-yl](2-thienyl)methanone (2p)
White solid, 1H NMR (400 MHz, DMSO-d6) δ (ppm): 11.78 (s, 1H, pyrrole-NH), 7.96 (d, J = 4.0 Hz, 1H, thiophene H), 7.77 (d, J = 4.0 Hz, 1H, thiophene H), 7.63-7.56 (m, 2H), 7.41-7.36 (m, 1H), 7.32 (t, J = 8.0 Hz, 1H, thiophene H), 7.25-7.18 (m, 2H); 13C NMR (100 MHz, DMSO-d6) δ (ppm): 181.63, 155.62 JCF = 244.8 Hz, 145.29, 133.37, 133.02, 133.00, 129.72, 128.68 JCF = 7.0 Hz, 128.22, 127,22, 122.68, 120.37, 120.07, 118.61 JCF = 17.6 Hz, 116.11 JCF = 20.6 Hz. ESI-HRMS m/z: calcd for C15H9ClFNOS ([M+H]): 306.0157, 308.0127; found: 305.9962, 308.0009.
2.1.3. The synthesis of 3-ethoxycarbonyl-4-(3-chloro-4-fluorophenyl)-1H-pyrrole (2q)
Triethylphosphite (33.2 g, 0.2 mol) was heated to 120 °C in a 100 mL 3-neck flask, and ethyl chloroacetate (29.4 g, 0.24 mol) was added dropwise over a period of 2 h. The mixture was held for 2 more hours at 165 °C till no chloroethane evolvement. The reaction mixture was fractionationally distilled under reduced pressure give the colorless liquid triethyl phosphonoacetate in 93% yield.30 t-BuOK (1.34 g, 12.0 mmol) were added in dry THF (15 mL), and triethyl phosphonoacetate (2.69 g, 12.0 mmol) was metered in, at room temperature the suspension was stirred for 30 min. A solution of 3-chloro-4-fluorobenzaldehyde (1.58 g, 10.0 mmol in 10 mL dry THF) was added dropwise into the reaction mixture and stirred for 2 h. The mixture was added to 20 mL water. After removing THF the mixture was extracted with EtOAc (75 mL, 25 mL × 3). The crude product was chromatographed on silica gel to furnish pure white solid 1-ethoxycarbonyl-2-(3-chloro-4-fluorophenyl)ethylene (2.51 g, 8.5 mmol, 85% yield). The construction of pyrrole moiety was the same as 2.1.1.
White solid, 1H NMR (400 MHz, DMSO-d6) δ (ppm): 11.64 (s, 1H, pyrrole-NH), 7.67 (dd, J1 = 8.0 Hz, J2 = 2.0 Hz, 1H, ArH), 7.51 (dd, J1 = 8.0 Hz, J2 = 2.0 Hz, 1H, pyrrole H), 7.44 (m, 1H, ArH), 7.34 (t, J = 8.0 Hz, 1H, ArH), 7.04 (t, J = 4.0 Hz, 1H, pyrrole H), 4.14 (dd, J1 = 12.0 Hz, J2 = 4.0 Hz, 2H, -COOCH2-), 1.20 (t, J = 8.0 Hz, 3H, -CH3); 13C NMR (100 MHz, DMSO-d6) δ (ppm): 163.97, 155.73 JCF = 245.0 Hz, 132.90 JCF = 3.9 Hz, 130.50, 129.22 JCF = 7.0 Hz, 126.16, 122.60, 119.70, 118.32 JCF = 17.6 Hz, 115.81 JCF = 20.6 Hz, 111.93, 58.87, 14.15. ESI-HRMS m/z: calcd for C13H11ClFNO2 ([M+H]): 268.0542, 270.0512; found: 268.0503, 270.0395.
2.1.4. The synthesis of 3-cyano-4-(3-chloro-4-fluorophenyl)-1H-pyrrole (2r)
Triethylphosphite (33.2 g, 0.2 mol) was heated to 150 °C in a 100 mL 3-neck flask, and chloroacetonitrile (18.1 g, 0.24 mol) was added dropwise over a period of 2 h. The mixture was held for 2 more hours at 150 °C till no chloroethane evolvement. The mixture was distilled under reduced pressure after cooling. The last fraction with bp 110-139 °C at 100pa contained the colorless liquid diethyl cyanomethylphosphonate at the yield of 92%.31 t-BuOK (1.34 g, 12.0 mmol) was added in dry THF (15 mL), and diethyl cyanomethylphosphonate (2.12 g, 12.0 mmol) was metered in, at rt the suspension was stirred for 30 min. A solution of 3-chloro-4-fluorobenzaldehyde (1.58 g, 10.0 mmol in 10 mL dry THF) was added dropwise into the reaction mixture and stirred for 2 h. The mixture was added to water (20 mL). After removing THF the mixture was extracted with EtOAc (75 mL, 25 mL × 3). The crude product was chromatographed on silica gel to furnish pure white solid 1-cyano-2-(3-chloro-4-fluorophenyl) ethylene (1.57 g, 8.7 mmol, 87% yield). The construction of pyrrole moiety was the same as 2.1.1.
White solid, 1H NMR (400 MHz, DMSO-d6) δ (ppm): 12.03 (s, 1H, pyrrole-NH), 7.83 (dd, J1 = 8.0 Hz, J2 = 2.0 Hz, 1H, ArH), 7.74 (dd, J1 = 8.0 Hz, J2 = 2.0 Hz, 1H, pyrrole H), 7.65 (m, 1H, ArH), 7.48 (t, J = 8.0 Hz, 1H, ArH), 7.43 (t, J = 4.0 Hz, 1H, pyrrole H); 13C NMR (100 MHz, DMSO-d6) δ (ppm): 155.97 JCF = 246.2 Hz, 131.17 JCF = 3.8 Hz, 128.95, 127.49, 126.16 JCF = 7.2 Hz, 122.55, 119.84 JCF = 17.9 Hz, 118.50, 117.25 JCF = 21.0 Hz, 117.10, 89.16. ESI-HRMS m/z: calcd for C11H6ClFN2 ([M+H]): 221.0283, 223.0253; found: 221.0192, 223.0185.
2.2 ANTICANCER ACTIVITY IN VITRO
2.2.1 Cytotoxicity toward 18 cell lines
All the 18 cell lines (Table 4) were purchased from Type Culture Collection of the Chinese Academy of Science, Shanghai, China, and were grown in RPMI-1640 (HyClone) or high glucose DMEM (HyClone) with 10% heat-inactivated fetal bovine serum (FBS, HyClone) and 1% Penicillin-Streptomycin Solution (HyClone) under a fully humidified atmosphere of 95% room air and 5% CO2 at 37 °C. Cells were washed with cold PBS (HyClone, phosphate-buffered Saline) and were trypsinized, collected and counted using a hemocytometer into a cell solution with the concentration of 5×104 cells/ml and 100 µL such cell solution was seeded in 96-well plate. After cultured for 24 h the cells were treated with desired doses of pyrrole derivatives for another 24 h. Four parallel wells were arranged for each concentration. 20 mL of MTT solution (5 mg/mL in PBS) was added to each well. After placing the 96-well plate in cell incubator for 4 h, the supernatant was removed and 100 mL of DMSO was added to each well for dissolving the formazan crystals. The percentage of cell viability was determined by measuring the absorbance at 570 nm using a Multiskan MK3 microplate reader (Thermo, USA).
2.2.2 Cell cycle analysis by FCM
Briefly, 1 × 105 (each well) MGC80-3 or CHO cells were plated in 6-well plate under standard culture condition. After 24 h, cells were fed with fresh medium and treated with 2r with the end concentration of 0, 1.0, 3.0 and 9.0 µM for 24 h, and three parallel wells were arranged. Cells were washed with ice cold PBS, trypsinized, and collected into single cell suspension. After fixed in 70% ice cold ethanol solution for 12 h, the cells were harvested and washed with ice cold PBS for 3 times to get rid of the residual ethanol and counted by a hemocytometer. 0.5 mL PI stain solution (50 µg/mL in PBS) was added into a cell solution of 5 × 105 cells/mL and incubated for 15 min at 4 °C. The cell solution was ready for FCM at 488 nm.
2.2.3 Cell death by FCM
1 × 105 (each well) CHO cells were plated in 6-well plate under standard culture condition. After 24 h, cells were fed with fresh medium and treated with 2r with the end concentration of 0, 1.0, 3.0 and 9.0 µM for 12 h, and three parallel wells were arranged. Cells were washed with ice cold PBS, trypsinized, collected and counted. Noting that all the medium components were needed for the test, centrifugal rotation should be increased. Annexin V-FITC stain solution (Annexin V: FITC Apoptosis Detection Kit, BD) was added and incubated for 20 min at rt After the removal of Annexin V-FITC stain solution, PI stain solution was added and incubated at 0 °C for 10 min. The cell solution was ready for testing by FCM.
2.2.4 Cell death by Hoechst 33342/PI staining
1 × 105 (each well) MGC80-3 or CHO cells were seeded in 6-well plate under standard culture condition. After 24 h, cells were fed with fresh medium and treated with 2r with the end concentration of 0, 1.0, 3.0, 9.0 and 27.0 µM for 12 h, and two parallel wells were arranged. Cells were washed with ice cold PBS gently for 2 times and Hoechst 33342 stain solution (10 µg/mL in PBS) was added and incubated for 30 min at 4 °C. Later the plate was washed with ice cold PBS gently for 2 times again and PI stain solution (10 µg/mL) was added and incubated for 15 min at 0 °C. The plate was washed with ice cold PBS gently for 2 times and 1.0 mL PBS was left inside. The 6-well plate was ready for observing and counting under fluorescence microscope (Olympus DP72+TL4+U-RFL-T, Japan).
2.2.5 Cell apoptosis and cell necrosis by TEM
Untreated and treated (5.0 µM 2r for 24 h) CHO cells were washed with ice cold PBS, trypsinized, and collected in 1.5 mL PE centrifuge tube. The cell sample was soaked in 2.5% fresh-prepared glutaraldehyde overnight at 4 °C, then washed with cold PBS for 3 times. The dehydration was conducted successively in 30%, 50%, 70%, 90% ethanol, 50% EtOH-acetone solution and 90% acetone, each time for 15-20 min at 4 °C, followed by the final dehydration which was fulfilled in 100% acetone at rt for 3 times. After the epoxy resin embedment and curing, the cell sample was ready for ultrathin section (70 nm) using an ultramicrotome (Leica Ultracut UCT, Germany). The ultrathin section was stained with lead citrate/uranyl acetate and examined under TEM (Tecnai G2 Spirit Biotwin, FEI Company) at 120 kV.
ACKNOWLEDGEMENTS
This project is supported by National Major Scientific and Technological Special Project of the PR China (2010ZX09401-404), and sincere thanks are given to Instrumental Analysis Center of Shanghai JiaoTong University for the comprehensive identification of the pyrrole compounds and the examination of ultrathin section under TEM.
References
1. K. Arima, H. Imanaka, M. Kousaka, A. Fukuda, and G. Tamura, Agr. Biol. Chem., 1964, 28, 575. CrossRef
2. K. Arima and M. Beppu, J. Bacteriol., 1964, 88, 143.
3. H. Nakano, S. Umio, K. Kariyone, K. Tanaka, T. Kishimoto, H. Noguchi, I. Ueda, H. Nakamura, and Y. Morimoto, Tetrahedron Lett., 1966, 7, 737. CrossRef
4. J. N. Roitman, N. E. Mahoney, W. J. Janisiewicz, and M. Benson, J. Agric. Food Chem., 1990, 38, 538. CrossRef
5. S. Forenza, L. Minale, R. Riccio, and E. Fattorusso, J. Chem. Soc., D, 1971, 1129. CrossRef
6. D. Kumar and D. S. Rawat, Opp. Challenge Scope Nat. Prod. Med. Chem., 2001, 37, 213.
7. P. S. Skella and G. P. Bean, J. Am. Chem. Soc., 1962, 84, 4655. CrossRef
8. H. Scheibler and H. Friese, Justus Liebigs Ann. Chem., 1925, 445, 141. CrossRef
9. T. Hirashita, K. Kinoshita, H. Yamamura, M. Kawai, and S. Araki, J. Chem. Soc., Perkin Trans. 1, 2000, 825. CrossRef
10. H. S. Lee, S. H. Kim, and J. N. Kim, Bull. Korean Chem. Soc., 2011, 32, 1748. CrossRef
11. T. Tomioka, Y. Takahashi, T. G. Vaughan, and T. Yanase, Org. Lett., 2010, 12, 2171. CrossRef
12. M. Swetha, P. V. Ramana, and S. G. Shirodkar, Asian J. Chem., 2011, 23, 522.
13. Y. H. Ma and J. X. Xu, Synthesis, 2012, 2225. CrossRef
14. D. Villemin and M. Ricard, Tetrahedron Lett., 1984, 25, 1059. CrossRef
15. P. Kisanga, D. McLeod, B. D’Sa, and J. G. Verkade, J. Org. Chem., 1999, 64, 3090. CrossRef
16. K. Wadhwa and J. G. Verkade, J. Org. Chem., 2009, 74, 4368. CrossRef
17. L. Fan and O. V. Ozerov, Chem. Commun., 2005, 4450. CrossRef
18. K. Shibata, K. Urano, and M. Matsui, Chem. Lett., 1987, 3, 519. CrossRef
19. E. M. Abbot, A. J. Bellamy, J. B. Kerr, and L. S. Mackirdy, J. Chem. Soc., Perkin Trans. 2, 1982, 425. CrossRef
20. K. Izod, Coord. Chem. Rev., 2002, 227, 153. CrossRef
21. A. Maercker, ‘The Wittig Reaction’, John Wiley & Sons, Inc., 2011.
22. A. B. Flynn and W. W. Ogilvie, Chem. Rev., 2007, 107, 4698. CrossRef
23. A. I. Arkhypchuk, Y. V. Svyaschenko, A. Orthaber, and S. Ott, Angew. Chem. Int. Ed., 2013, 52, 6484. CrossRef
24. M. Artica, R. Di Santo, R. Costi, S. Massa, A. Retico, M. Artico, G. Apuzzo, G. Simonetti, and V. Strippoli, J. Med. Chem., 1995, 38, 4223. CrossRef
25. A. Tafi, R. Costi, M. Botta, R. Di Santo, F. Corelli, S. Massa, A. Ciacci, F. Manetti, and M. Artico, J. Med. Chem., 2002, 45, 2720. CrossRef
26. R. Di Santo, A. Tafi, R. Costi, M. Botta, M. Artico, F. Corelli, M. Forte, F. Caporuscio, L. Angiolella, and A. T. Palamara, J. Med. Chem., 2005, 48, 5140. CrossRef
27. J. U. Schweichel and H. J. Merker, Teratology, 1973, 7, 253. CrossRef
28. L. Yu, A. Alva, H. Su, P. Dutt, E. Freundt, S. Welsh, E. H. Baehrecke, and M. J. Lenardo, Science, 2004, 304, 1500. CrossRef
29. L. Galluzzi, I. Vitale, J. M. Abrams, E. S. Alnemri, E. H. Baehrecke, M. V. Blagosklonny, T. M. Dawson, V. L. Dawson, W. S. El-Deiry, S. Fulda, E. Gottlieb, D. R. Green, M. O. Hengartner, O. Kepp, R. A. Knight, S. Kumar, S. A. Lipton, X. Lu, F. Madeo, W. Malorni, P. Mehlen, G. Nunez, M. E Peter, M. Piacentini, D. C. Rubinsztein, Y. Shi, H.-U. Simon, P. Vandenabeele, E. White, J. Yuan, B. Zhivotovsky, G. Melino, and G. Kroemer, Cell Death and Differ., 2012, 19, 107. CrossRef
30. A. L. Gutman, E. Shkolnik, B. Tishin, G. Nisnevich, and I. Zaltzman, Finetech Laboratories, US Pat. 6492522 B1, 2002.
31. J. Gebhardt, N. Gotz, H. Jaedicke, G. Mayer, and M. Rack, BASF Aktiengesellschaft, Interational Pat.2005/063780 A1, 2005.