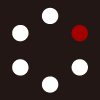
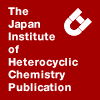
HETEROCYCLES
An International Journal for Reviews and Communications in Heterocyclic ChemistryWeb Edition ISSN: 1881-0942
Published online by The Japan Institute of Heterocyclic Chemistry
e-Journal
Full Text HTML
Received, 14th December, 2013, Accepted, 26th February, 2014, Published online, 13th March, 2014.
DOI: 10.3987/COM-13-12915
■ Synthesis and Photophysical Properties of New Pyrazolino[60]fullerenes with Fluorenyl and Fluorinated Phenyl Substituents
Subo Shen, Shanshan He, Haonan Cheng, Changrui Xu, Hongmei Deng, and Jianmin Zhang*
Department of Chemistry, University of British Columbia, 2036 Main Mall, Vancouver B.C., V6T 1Z1, Canada
Abstract
Two types of triads based on Pyrazolino[60]fullerenes with fluorenyl and fluorinated phenyl substituents have been synthesized via the [3+2] dipolar cycloaddition reaction between C60 and corresponding hydrazones in good yields. Photophysical properties of these triads have also been systematically investigated, and their weak emissions indicated fluorescent quenching induced by intramolecular charge transfer may occur between the donor and acceptor parts of these systems.INTRODUCTION
Since the disclosure of novel and unique electronic properties of fullerene in the past decades, it has become a burgeoning interest in the areas of organic photovoltaics,1-3,7 traditional organic synthesis,4-6 and nanotechnology.8-9 In recent years, the application of C60 fullerene derivatives10,11 in the field of optoelectronic devices has received great attention,12-17 and there is an urgent need to improve and optimize the synthetic methods of optoelectronic active C60 fullerene derivatives.
Our previous works have shown the efficient synthesis of fluorophore substituted pyrazolino[60]fullerene derivatives,18,19 and these studies were limited to single type of heterocyclic products, we are anxious to make innovation on the design of the product structures. Considering about good fluorescence properties of fluorene derivatives and special electronic effect of fluorine benzene, we decided to introduce fluorene and fluorinated phenyl to fullerene C60. Our continued interest promotes us to extend our research to multiple substituted pyrazolino[60]fullerene derivatives by using asymmetric 1,3-dipole hydrazones. Based on the symmetrical models (shown in Figure 1) we have designed two types of pyrazolino[60]fullerenes triads with fluorenyl and fluorinated phenyl substituents. Based on a large number of related literatures, such a design concept on the applications of fullerenes is not very common. Herein, we present optimized synthesis of these compounds and the detailed investigations of their photophysical properties by UV-VIS absorption and fluorescence spectra.
RESULTS AND DISCUSSION
Fluorenyl and fluorinated phenyl groups were chosen as fluorophores A and B. Type I and Type II products were obtained by treating the hydrazone intermediates with fullerene (Schemes 1 and 2). In view of our previous research on synthesis of pyrazoline derivatives,19 a series of hydrazones 1I-(a-i) were prepared in our general works for another publication, and they were introduced to C60 via the [3+2] dipolar cycloaddition reaction.20-22 The synthetic route of target compounds 2I-(a-i) is shown in Scheme 1.
The reaction of hydrazones 1I-a with C60 in the presence of PhI(OAc)2 was chosen as the model reaction to screen the optimized conditions. The screening results of the optimized conditions are summarized in Table 1.
It was found that the yield of the product 2I-a could be improved by increasing the reaction time (entries 1~3). However, the excessive extension of time had a negligible effect on the product yield. Changing the ratio of reactants could also affect the yield of product 2I-a (entries 2, 4, 5). In addition, an adverse effect was observed on the yields of products by raising the reaction temperature due to the appearance of a large number of impurities (entries 2, 6, 7). Using other solvents, such as benzene or chlorobenzene could not improve the reaction yield (entry 8). After careful comparisons, the best reaction condition was selected as C60 (0.1 mmol), 1I (0.2 mmol) and PhI(OAc)2 (0.2 mmol) in 40 mL of toluene at 30 °C for 5 h (entry 2).
According to the optimized reaction conditions, a series of target compounds 2I-(a-i) have been prepared. As shown in Table 2, all products of Type I afforded 32-37% isolated yields (70–76% yields based on reacted C60).
The other type of target compounds 2II-(a-f) has also been prepared in a similar manner, which is shown in Scheme 2.
Similar screening processes of the optimized reaction conditions between C60 and 1II-a are shown in Table 3.
According to the optimized reaction conditions, a series of target compounds 2II-(a-f) have been prepared. As shown in Table 4, all the products of Type II afforded 33-38% isolated yields (70–79% yields based on reacted C60).
It's worth mentioning that the yields of the products 2I and 2II are very close to each other regardless of the number or the substitution position of fluorine atoms on the benzene ring. Generally, the reaction yield would be reduced by the influence of electron-withdrawing group, but the expected results were not observed. This phenomenon may be caused by the increasing of the reactants solubility in the solvent by the introduction of fluorine atoms.
The structures of all products 2I and 2II were fully characterized by spectroscopic methods (UV-VIS, FTIR, 1H NMR, 19F NMR, 13C NMR, and MALDI-FTICR-MS). The mass spectra of the products 2I and 2II exhibit the expected [M]+ or [M+H]+ peaks and well matching the calculated molecular weights. The 1H and 19F NMR spectra display the expected chemical shifts and splitting patterns for all protons and fluorine atoms. For instance, in the 13C NMR spectra of 2I-f, 48 peaks appear in the range of 119-148 ppm, and among them 4 peaks are due to the sp2 carbons of benzene ring, 12 peaks are due to the sp2 carbons of fluorenyl moiety and other 32 peaks are caused by C60 skeleton. It is indicated that the pyrazolelin ring is formed between a pentatomic ring and a hexatomic ring to get the [5,6] ring open products (Scheme 3). Two peaks at about 92.23 and 81.46 ppm are assigned to the two sp3 carbons of C60, and the peak at 37.11 ppm is related to the sp3 carbon of the fluorenyl moiety.
UV-VIS absorption and fluorescence spectra for comparison in symmetrical types
After testing different concentrations of the products, 6×10-6 mol/L in CHCl3 was chosen as the best condition for the ultraviolet analysis of 1I-(a-e), 1II-(a-e), 2I-(a-e) and 2II-(a-e). As shown in Figure 2, 2I-(a-e) show stronger ultraviolet absorption due to the expansion of better electron transportation after C60 connecting with 1I-(a-e), this rule also applies to Type II. Considering about the peak positions, the peaks of 1I-(a-e) and 1II-(a-e) in 250~270 nm are due to π~π* electronic transitions, and peaks in 300~350 nm are caused by fluorenyl moiety. The peaks of 2I-(a-e) and 2II-(a-e) in 280~330 nm are caused by fluorenyl moiety, peaks in 250~260 nm are caused by C60.
1II-(a-e) show two absorption bands centered at 250 and 325 nm respectively, which could be attributed to the π-π* transition and intramolecular charge tranfer process between fluorenyl (donor) and fluorinated substituents (acceptor). However, in the spectra of 1I-(a-e), only tiny peaks in the latter bands could be observed. The lack of charge transfer bands indicate the intramolecular charge tranfer process may largely be prohibited in the 1I-(a-e) series. This difference may arise from the better electron transportation of fluorenyl and fluorinated groups in the structure of the 1II-(a-e) series. This difference was not observed in the UV-vis spectra of 2I-(a-e) and 2II-(a-e), and all of them show the characteristic absorption bands of the π-π* transition and intramolecular charge tranfer process. This may indicate that the fullerene part as an acceptor takes part in the intramolecular charge tranfer process in the triads system, and this transfer pathway may be superior than the way through fluorenyl and fluorinated groups.
First of all, the fluorescence intensities of 1I-(a-e) are stronger than 1II-(a-e) as shown in Figure 3. The fluorescence intensities of 1II-(a-e) decrease along with the increasing of fluorine atom number on the phenyl. Second, it was found that products 2I-(a-e) and 2II-(a-e) both display weaker fluorescence intensity than reactants obviously which is caused by fluorescence quenching of the compounds with C60. The fluorescence spectra of 2I-(a-e) and 2II-(a-e) tend to be similar―two main absorption peaks appear at about 412 and 440 nm. The fluorescence intensities decrease obviously when the hydrazone derivatives 1I-(a-e) and 1II-(a-e) synthesized to be the pyrazolino C60 derivatives. We inferred that the too-strong electron-withdrawing effect of C60 changes the electron transferring in pyrazolino C60 derivatives, and this effect makes the fluorescence properties of 2I-(a-e) and 2II-(a-e) to be similar.
Electrochemical examinations
In order to further verify electronic transmission of Type I and Type II products, some of them were chosen to be tested by CV(cyclic voltammetry). In Figure 4, products 2I-a and 2II-a show a quasi-reversible electrochemical behavior, product 2I-a has two one-electron reduction waves at -1.91V and -1.27V. However, product 2II-a shows three one-electron reduction waves at -1.96V, -1.54V and -0.93V. Furthermore, the products of Type I show similar shape to 2I-a, which is also applied to Type II.
All the initial reduction of voltages vs Fc/Fc+ were used to calculate LUMO energies of the products. If the initial oxidation voltages were chosen to calculate HOMO energies, values of HOMO energies and LUMO-HOMO energy gaps (Eg) would be get out of parentheses in Table 5. On the other hand, combined with the edge of the UV peak wavelength, LUMO-HOMO energy gaps (Eg) could be obtained approximately in parentheses, then HOMO energies could also been calculated by LUMO energies and LUMO-HOMO energy gaps. After data analysis, it was found that the results obtained by the two different calculation methods are relatively uniform. The most important point should be emphasized that LUMO-HOMO energy gaps (Eg) of Type II products are about 0.1~0.3 eV smaller than that of Type I products, enabling an easier electron-transfer process.
In summary, two types of asymmetric pyrazolino[60]fullerenes derivatives with various fluorenyl and fluorinated substituents have been synthesized via the [3+2] dipolar cycloaddition reaction between C60 and corresponding hydrazones. By this method, we have successfully introduced fluorescent fluorenyl groups onto C60 backbones, and it offers an effective way to synthesize asymmetric pyrazolino[60]fullerenes with different substituents. The investigation of UV-VIS absorption and fluorescence spectra indicated that the existence of effective intramolecular charge tranfer process between fluorenyl and fluorinated substituents in the 1I and 1II series, and this process could be perturbed by the intramolecular charge tranfer process between fluorenyl groups and fullerene in 2I and 2II series. Further studies on the synthesis of other fluorophores substituted pyrazolino[60]fullerenes, and detailed investigation of the intramolecular charge tranfer process in our systems are ongoing.
EXPERIMENTAL
Typical procedure for the synthesis of 1-(N-fluorenyl)-3-(3'–fluorophenyl)pyrazolino[C60]fullerene (2I-a). A mixture of C60 (72 mg, 0.1 mmol), hydrazone 1I-a (60.4 mg, 0.2 mmol) and PhI(OAc)2 (64 mg, 0.2 mmol) were dissolved in 40 mL of toluene and stirred at 30 °C for 5 h. The solvent was then evaporated in vacuum and the residue was separated on a silica gel column using CS2 or CS2-toluene as the eluent to afford unreacted C60 and the adduct 2I-a; Brown solid; IR (KBr, cm-1): ν 3455, 2919, 2850, 1605, 1578, 1047, 574, 526; 1H NMR (500 MHz, CS2–CDCl3): δ 8.32−8.29 (m, 2H), 8.06 (s, 1H), 7.95 (d, J = 8.0 Hz, 1H), 7.81 (d, J = 8.0 Hz, 1H), 7.74 (d, J = 7.5 Hz, 1H), 7.52 (d, J = 7.5 Hz, 1H), 7.36 (t, J = 7.5 Hz, 1H), 7.28 (t, J = 7.5 Hz, 1H), 7.21 (t, J = 8.5 Hz, 2H), 3.98 (s, 2H); 19F NMR (470 MHz, CS2–CDCl3): δ-109.02 (s, 1F); 13C NMR (125 MHz, CS2–CDCl3): δ 162.50, (d, J = 246.25 Hz), 147.23, 146.85, 146.03, 145.92, 145.64, 145.59, 145.53, 145.46, 145.43, 145.14, 144.90, 144.82, 144.80, 144.17, 143.94, 143.93, 143.08, 142.83, 142.81, 142.59, 142.53, 142.12, 142.06, 141.93, 141.84, 141.58, 141.41 (d, J = 2.5 Hz); 140.83, 139.99, 139.47, 139.06, 137.17, 136.21, 135.95, 134.45 (d, J = 8.75 Hz), 130.01 (d, J = 8.75 Hz), 128.74, 128.00, 126.76, 126.50, 125.11, 124.79, 123.93, 123.90, 122.97, 120.78, 120.03, 119.63, 115.82 (d, J = 21.25 Hz), 115.44 (d, J = 22.5 Hz), 92.30 (sp3 C of C60 sphere), 80.83 (sp3 C of C60 sphere), 37.05; MALDI-FTICR-MS: [M]+ Calculated for C80H13N2F1: 1020.1073; found 1020.10573.
Electrochemical measurements were performed using a Base 2000 CV system electrochemical analyzer. Cyclic voltammetric measurements were carried out in o-dichlorobenzene/acetonitrile (4:1) containing 0.1 M n-Bu4NPF6 as a supporting electrolyte, using a Pt working electrode (φ=1 mm) with ferrocene, and a vitreous carbon rod counter electrode, scan rate: 100 mV/s.
ACKNOWLEDGEMENTS
This work was supported by the National Natural Science Foundation of China (Nos. 21272151). The authors thank the Instrumental Analysis & Research Center of Shanghai University and Doctor Deng, Hongmei.
References
1. I. S. Doe, J. Smith, and P. Roe, J. Am. Chem. Soc., 1968, 90, 8234.
2. Y. Y. Liang, Z. Xu, J. B. Xia, S. T. Tsai, Y. Wu, G. Li, C. Ray, and L. P. Yu, Adv. Mater., 2010, 22, 135. CrossRef
3. H. Y. Chen, J. Hou, S. Zhang, Y. Liang, G. Yang, Y. Yang, L. Yu, Y. Wu, and G. Li, Nat. Photonics, 2009, 3, 649. CrossRef
4. R. Lydia, J. L. Sabina, R. D. Luis, A. J. John, and R. Ponnadurai, Heterocycles, 2012, 84, 719. CrossRef
5. C. B. Miao, Z. Y. Tian, X. J. Ruan, X. Q. Sun, and H. T. Yang, Heterocycles, 2011, 83, 1615. CrossRef
6. K. Tsubaki, Y. Murata, K. Komatsu, T. Kinoshita, and K. Fuji, Heterocycles, 1999, 51, 2553. CrossRef
7. C. J. Brabec, N. S. Sariciftci, and J. C. Hummelen, Adv. Funct. Mater., 2001, 11, 15. CrossRef
8. D. Gust, T. A. Moore, and A. L. Moore, Acc. Chem. Res., 2001, 34, 40. CrossRef
9. H. B. Liu, J. L. Xu, Y. J. Li, and Y. L. Li, Acc. Chem. Res., 2010, 43, 1496. CrossRef
10. S. S. Babu, H. Mohwald, and T. Nakanishi, Chem. Soc. Rev., 2010, 39, 4021. CrossRef
11. J. L. Delgado, N. Martin, P. Cruz, and F. Langa, Chem. Soc. Rev., 2011, 40, 5232. CrossRef
12. H. Y. Zhao, Z. B. Liu, X. L. Zhang, and J. G. Tian, Chin. J. Chem., 2012, 30, 1766. CrossRef
13. T. Bura, N. Leclerc, S. Fall, P. Leveque, and T. Heiser, J. Am. Chem. Soc., 2012, 134, 17404. CrossRef
14. C. D. Guo, W. R. Teng, H. X. Wu, J. Z. Shen, and X. M. Deng, Chin. J. Chem., 2005, 23, 1113. CrossRef
15. T. Michinobu, K. Okoshi, Y. Murakami, K. Shigehara, K. Ariga, and T. Nakanishi, Langmuir, 2013, 29, 5337. CrossRef
16. D. Peckus, A. Devizis, R. Augulis, S. Graf, D. Hertel, and V. Gulbinas, J. Phys. Chem. C., 2013, 117, 6039. CrossRef
17. J. D. Wood, J. L. Jellison, A. D. Finke, and L. C. Wang, J. Am. Chem. Soc., 2012, 134, 15783. CrossRef
18. B. Q. Lu, J. M. Zhang, and J. Li, Tetrahedron, 2012, 68, 8924. CrossRef
19. B. Q. Lu, J. M. Zhang, and M. J. Wang, Chin. J. Chem., 2012, 30, 1345. CrossRef
20. H. T. Yang, X. J. Ruan, C. B. Miao, and X. Q. Sun, Tetrahedron Lett., 2010, 51, 6056. CrossRef
21. Y. Matsubara, H. Tada, S. Nagase, and Z. I. Yoshida, J. Org. Chem., 1995, 60, 5372. CrossRef
22. F. Langa, P. Cruz, E. Espildora, A. Hoz, J. L. Bourdelande, L. Sánchez, and N. Martín, J. Org. Chem., 2001, 66, 5033. CrossRef
23. A. A. Peyghan, H. Soleymanabadi, and M. Moradi, J. Phys. Chem. Solids, 2013, 74, 1594. CrossRef