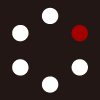
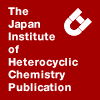
HETEROCYCLES
An International Journal for Reviews and Communications in Heterocyclic ChemistryWeb Edition ISSN: 1881-0942
Published online by The Japan Institute of Heterocyclic Chemistry
e-Journal
Full Text HTML
Received, 31st July, 2014, Accepted, 28th August, 2014, Published online, 8th September, 2014.
DOI: 10.3987/COM-14-S(K)98
■ Synthesis of Intermediary P3 Phosphazenium Framework and Its Derivatization to Chiral Cationic Macrocycles Including Two P3 Phosphazenium Units with Hydrogen Bond Donor Sites
Masahiro Terada,* Kengo Goto, Takashi Ikehara, and Azusa Kondoh
Department of Chemistry, Graduate School of Science, Tohoku University, Aramaki, Aoba-ku, sendai 980-8578, Japan
Abstract
A new synthetic route to key intermediary phosphonium possessing a P3 phosphazene framework was developed. The reaction of the obtained intermediary phosphonium with chiral diamine, (1S,2S)-1,2-diphenyl-1,2-ethanediamine, resulted in the formation of 18-membered macrocyclic bisphosphazenium, the structure of which was verified by single-crystal X-ray diffraction analysis. The macrocyclic bisphosphazenium showed potential application as a chiral anion receptor and a chiral template in asymmetric catalysis.Stable cationic organic molecules bearing hydrogen bond donor sites have attracted considerable attention in synthetic organic and supramolecular chemistry as well as molecular sensing, because those molecules bind strongly to negatively charged molecules through the formation of hydrogen bonds.1 Among them, positively charged guanidinium and related functional groups that form N+ ̶H•••X--type hydrogen bonds have been well investigated. During the past decade, chiral versions of those cationic molecules have triggered much interest because the chiral molecules enable recognition of chiral anionic molecules2 or function as a chiral template in the asymmetric catalysis of negatively charged nucleophilic species.3 However, the more stable cationic molecules in which the conjugate base of the cationic species is rendered more basic, rather than the molecules having guanidinium and related moieties, have rarely been exploited,4,5 despite the fact that the development of such stable cationic molecules would broaden the scope of anionic species to include the less stable and hence the more basic ones. Iminophosphorane derivatives are an attractive candidate for the development of such stable cationic molecules having hydrogen bond donor sites,6,7 precisely because the introduction of additional iminophosphorane subunits to the iminophosphorane core, which increases the number of iminophosphorane units, would stabilize its conjugate acid to afford a series of stable cationic molecules, namely, phosphazeniums.7,8 Inspired by the idea of developing chiral stable cationic molecules having hydrogen bond donor sites, we envisaged the synthesis of novel chiral phosphazeniums having plural iminophosphorane units. In this context, we adopted a P3 phosphazene framework,5 in which three iminophosphorane units are connected to realize resonance stabilization of the protonated form of phosphazenium, to construct a C2 symmetrical structure around the central iminophosphorane core, because the C2-symmetry has been proven to be a fundamental molecular design in chiral recognition and asymmetric catalysis. However, as methods for the preparation of P3 phosphazenes are quite limited,7 we exploited a new and efficient synthetic route to the P3 phosphazene framework from readily available chemical sources. Herein, we report the synthesis of key intermediary phosphonium 1 bearing the P3 phosphazene framework (Figure 1). We also attempted the reaction of 1 with the commercially available chiral diamine, (1S,2S)-1,2-diphenyl-1,2-ethanediamine ((S,S)-DPEN), to derivatize 1 into chiral P3 phosphazenium. As a result, the formation of 18-membered macrocyclic product 2 was accomplished, in which two P3 phosphazenium units were embedded into the formed macrocycle. In addition, the characterization of 2 by single-crystal X-ray diffraction analysis is documented.
The synthesis of key intermediary phosphonium 1 began with the Staudinger reaction of 2-chloro-1,3-dimethyl-1,3,2-diazaphosphorane (3)9 and thiophosphoryl triazide (4)10 (Scheme 1), which was prepared freshly from thiophosphoryl trichloride and sodium azide. The double Staudinger reaction of 3 with 4 was performed for six days at 45 °C,11 and the resultant mixture was quenched with saturated NH4Cl aq. solution and then purified by silica-gel column chromatography to afford thiophosphoryl azide 5, the precursor of intermediary phosphonium 1, in 65% isolated yield. Of particular interest is that further Staudinger reaction of 5 with 3 was completely suppressed to afford double reaction product 5 as the dominant isomer, presumably due to the decrease of the reactivity with the increase of the electron density of the central phosphorus atom of 5. Further transformation of 5 into phosphonium 1, the key intermediate of the P3 phosphazene framework, was carried out with oxalyl chloride. The introduction of a chiral source to phosphonium 1 was performed by using (S,S)-DPEN and in situ generated phosphonium 1. Although the reaction resulted in a mixture of several products, one of those products could be isolated. Thorough analysis of the isolated product suggested that 18-membered macrocyclic bisphosphazenium 2 was unexpectedly formed, albeit in a low yield (17% yield; two steps from 5), instead of the product composed of a single P3 phosphazenium unit (Scheme 2).
As illustrated in Figure 2, the structure of macrocyclic bisphosphazenium 2 was successfully verified by single-crystal X-ray diffraction analysis.12 Interestingly, one of two counter anions, chloride, is located inside the macrocycle where it effectively interacts with the six hydrogen atoms through the formation of
N+ ̶H•••Cl--type hydrogen bonds. In fact, the distances between the chlorine atom and the nitrogen atoms bearing those six hydrogen atoms range from 3.23 to 3.46 Å.
The formation of macrocyclic bisphosphazenium 2 is attributed to the difference in the reactivity of the phosphorus atoms of 1 and the formed ring size in the reaction of phosphorus atom(s) with the chiral 1,2-diamine, (S,S)-DPEN. It can be expected that the central phosphorus atom of phosphonium 1 is the most reactive, because electron-withdrawing chloride and azide are attached to it. As shown in Figure 3, the formation of the P-N bond between the central phosphorus atom and one of the two amino groups of 1,2-diamine, giving intermediate 6, is followed by the cyclization step. The other amino group of 1,2-diamine forms a ring-closing P-N bond with either the central phosphorus atom or one of the two terminal phosphorus atoms. The ring closure with the central phosphorus atom leads to kinetically favorable five-membered ring product 7, whereas the cyclization with the terminal phosphorus atom affords kinetically less favorable seven-membered ring product 8. It is obvious that macrocyclic bisphosphazenium 2 was afforded via the formation of five-membered ring product 7 despite the fact that the terminal phosphorus atoms have a better leaving group, chloride, than the central phosphorus atom bearing an azide as the less reactive leaving group. The observed result strongly suggests that the kinetic advantage of the five-membered ring formation significantly dominates over the difference in reactivity of the leaving group.
In conclusion, we have developed a new synthetic route to the key intermediary phosphonium bearing the P3 phosphazene framework. Thus-obtained intermediary phosphonium was further reacted with the chiral diamine, (1S,2S)-1,2-diphenyl-1,2-ethanediamine, to afford unexpected 18-membered macrocyclic bisphosphazenium. The structure of the macrocyclic bisphosphazenium was verified by single-crystal X-ray diffraction analysis. The present macrocyclic bisphosphazenium is potentially useful as a chiral anion receptor and a chiral template in asymmetric catalysis. Further studies will be conducted with an eye to applying the intermediary phosphonium to the construction of novel chiral P3 phosphazenium derivatives and macrocyclic bisphosphazenium derivatives for catalytic enantioselective transformation as well as chiral anion recognition.
EXPERIMENTAL
Infrared spectra were recorded on a JASCO FT/IR-4100 spectrometer. 1H NMR spectra were recorded on a JEOL JMTC-600 (600 MHz) spectrometer and a JEOL JNM-ECS400 (400 MHz) spectrometer. Chemical shifts are reported in ppm from the solvent resonance or tetramethylsilane (TMS) as the internal standard (CDCl3: 7.26 ppm, TMS: 0.00 ppm). Data are reported as follows: chemical shift, integration, multiplicity (s = singlet, d = doublet, t = triplet, brs = broad, m = multiplet), and coupling constants (Hz). 13C NMR spectra were recorded on a JEOL JNM-ECS400 (100.5 MHz) spectrometer with complete proton decoupling. Chemical shifts are reported in ppm from the solvent resonance as the internal standard (CDCl3: 77.0 ppm). 31P NMR spectra were recorded on a JEOL JNM-ECS400 (169 MHz) spectrometer. Chemical shifts are reported in ppm from 85% H3PO4 aq. as the external standard (H3PO4 in CDCl3: 0.0 ppm). X-Ray data were collected on a BrukerAXS APEXII diffractometer with graphite monochromated Mo-Ka radiation (λ 0.71073 Å). Optical rotations were measured on a JASCO P-1020 digital polarimeter with a sodium lamp and reported as follows: [α] T ºC D (c = g/100 mL, solvent). Analytical thin-layer chromatography (TLC) was performed on Merck precoated TLC plates (silica-gel 60 GF254, 0.25 mm). Flash column chromatography was performed on silica-gel 60 N (Merck 230-400 mesh). High-resolution mass spectral analysis was performed on a Bruker Daltonics solariX 9.4T spectrometer at the Research and Analytical Center for Giant Molecules, Graduate School of Science, Tohoku University.
Materials. 2-Chloro-1,3-dimethyl-1,3,2-diazaphosphorane (3)9 and thiophosphoryl triazide (4)10 were prepared following the reported procedure. Unless otherwise noted, all reactions were carried out under nitrogen atmosphere in dried glassware. Dichloromethane (CH2Cl2) was supplied by Kanto Chemical Co., Inc. as “Dehydrated Solvent System”. Reagents were purchased from commercial suppliers and used without further purification. Other simple chemicals were used as received.
Preparation of Thiophosphoryl Azide 5. To a solution of freshly prepared thiophosphoryl triazide (4) (1.0 mmol) in MeCN (5.0 mL) was added 2-chloro-1,3-dimethyl-1,3,2-diazaphosphorane (3) (366 mg, 2.4 mmol) at room temperature. The reaction mixture was warmed to 45 °C and stirred for six days.11 The reaction was quenched by adding sat. NH4Cl aq. solution, and the mixture was extracted with CH2Cl2. The combined organic layer was washed with brine, dried over anhydrous Na2SO4, and concentrated under reduced pressure after filtration. The residue was purified by flash column chromatography (silica-gel, elution with hexane/EtOAc = 3/1 to 1/1) to give product 5 in 65% yield as a white solid. Caution! This column chromatography should be conducted in a hood because some byproducts could be toxic. 1H NMR (400 MHz, CDCl3): δ 2.75 (12H, dd, J = 8.0, 13.4 Hz), 3.07-3.15 (4H, m), 3.35-3.91 (4H, m); 13C NMR (100.5 MHz, CDCl3): δ 31.7, 46.4 (dt, J = 3.8, 6.7 Hz); 31P NMR (162 MHz, CDCl3): δ 19.4 (d, J = 36.9 Hz), 37.6 (t, J = 36.9 Hz); IR (ATR) 2935, 2883, 2821, 2132, 1472, 1459, 1457, 1381, 1253, 1212, 1159, 1037, 949, 863, 813 cm-1; HRMS (ESI): Calcd for C8H20Cl2N9P3S ([M+H]+) 438.0225, Found 438.0225.
Preparation of 18-Membered Macrocyclic Bisphosphazenium 2 from 5 via in situ Generated 1. To a solution of 5 (2.0 g, 4.5 mmol) in toluene (9.0 mL) was added oxalyl chloride (0.74 mL, 8.6 mmol) at 0 °C. After stirring for 5 min, the reaction mixture was warmed to room temperature and stirred for 2 h. After removing the organic solvent in vacuo, CH2Cl2 (10 mL) was added. This solution was added to a solution of (S,S)-1,2-diphenyl-1,2-ethanediamine (1.4 g, 6.8 mmol) and triethylamine (4.4 mL, 32 mmol) in CH2Cl2 (440 mL) at -78 °C via a cannula. After stirring for 30 min, the reaction mixture was warmed to 0 °C and stirred for 30 min. Then, the mixture was refluxed for 60 h. The reaction was quenched with 1N HCl. The mixture was extracted with CH2Cl2, and dried over anhydrous Na2SO4. After removal of the solvent, the residue was purified by flash column chromatography (silica-gel, elution with CH2Cl2/MeOH = 35/1 to 15/1). A mixture of acetone and hexane was added to the obtained product and the resultant suspension was stirred for 2 h at room temperature. Then, the suspension was filtered to give product 2 in 17% yield (calculated as X = Cl) as a white solid; [α]26D +6.7 (c 1.01, CHCl3); 1H NMR (600 MHz, CDCl3): δ 1.15 (8H, s), 2.39 (6H, d, J = 10.8 Hz), 2.87 (6H, d, J = 11.1 Hz), 3.15-3.55 (8H, m), 7.15-7.35 (6H, m), 7.43 (4H, t, J = 10.8 Hz); 13C NMR (100.5 MHz, CDCl3): δ 26.7, 29.8 (d, J = 5.8 Hz), 30.2 (d, J = 5.8 Hz), 45.7 (d, J = 14.3 Hz), 46.3 (d, J = 14.3 Hz), 84.1, 120.0, 125.2, 128.7, 140.7 (d, J = 4.7 Hz), 149.6 (dd, J = 12.5, 18.6 Hz); 31P NMR (162 MHz, CDCl3): δ 30.5 (t, J = 42.6 Hz), 36.2 (d, J = 42.6 Hz); IR (ATR) 3227, 3064, 3028, 2879, 1496, 1472, 1457, 1455, 1433, 1380, 1354, 1294, 1255, 1165, 1116, 1097, 1066, 1063, 1041, 980, 951 cm-1; HRMS (ESI): Calcd for C72H96ClN20P6 ([M-X]+) 1461.6236, Found 1461.6239.
ACKNOWLEDGEMENTS
This work was partially supported by a Grant-in-Aid for Scientific Research on Innovative Areas “Advanced Molecular Transformations by Organocatalysts” from MEXT, Japan, and a Grant-in-Aid for Scientific Research (B) (Grant No. 24350045) from the JSPS. We also thank Professor Takeaki Iwamoto and Professor Shintaro Ishida for their kind support in the single-crystal X-ray diffraction analysis.
References
1. For reviews on cationic receptors, see: A. Bianchi, K. Bowman-James, and E. Garcia-Espana, Supramolecular Chemistry of Anion. WILEY-VCH. New York, 1997; R. Martínez-Máñez and F. Sancenón, Chem. Rev., 2003, 103, 4419; CrossRef F. P. Schmidtchen and M. Berger, Chem. Rev., 1997, 97, 1609; CrossRef M. D. Best, S. L. Tobey, and E. V. Anslyn, Coord. Chem. Rev., 2003, 240, 3; CrossRef P. A. Gale, Coord. Chem. Rev., 2003, 240, 191; CrossRef C. Schmuck, Coord. Chem. Rev., 2006, 250, 3053; CrossRef A. E. Hargrove, S. Nieto, T, Zhang, J. L. Sessler, and E. V. Anslyn, Chem. Rev., 2011, 111, 6603. CrossRef
2. For reviews on chiral guanidinium-containing receptors, see: K. A. Schug and W. Lindner, Chem. Rev., 2005, 105, 67 and references therein; CrossRef P. Blondeau, M. Segura, R. Pérez-Fernández, and J. de Mendoza, Chem. Soc. Rev., 2007, 36, 198. CrossRef
3. For reviews on enantioselective catalysis by using chiral guanidines, see: T. Ishikawa and T. Kumamoto, Synthesis, 2006, 737; CrossRef D. Leow and C.-H. Tan, Chem. Asian J., 2009, 4, 488; CrossRef Y. Sohtome and K. Nagasawa, Synlett, 2010, 1; M. Terada, J. Synth. Org. Chem. Jpn., 2010, 68, 1159. CrossRef
4. D. Uraguchi and T. Ooi, J. Synth. Org. Chem. Jpn., 2010, 68, 1185; CrossRef J. S. Bander and T. H. Lambert, J. Am. Chem. Soc., 2012, 134, 5552; CrossRef T. Takeda and M. Terada, J. Am. Chem. Soc., 2013, 135, 15306; CrossRef T. Takeda and M. Terada, Aust. J. Chem., 2014, 67, 1124; CrossRef M. G. Núňes, A. J. M. Farley, and D. J. Dixon, J. Am. Chem. Soc., 2013, 135, 16348. CrossRef
5. M. Terada, K. Goto, M. Oishi, T. Takeda, E. Kwon, and A. Kondoh, Synlett, 2013, 24, 2531. CrossRef
6. R. Schwesinger, Chimia, 1985, 39, 269.
7. R. Schwesinger, H. Schlemper, C. Hasenfratz, J. Willaredt, T. Dambacher, T. Breuer, C. Ottaway, M. Fletschinger, J. Boele, H. Fritz, D. Putzas, H. W. Rotter, F. G. Bordwell, A. V. Satish, G.-Z. Ji, E.-M. Peters, H. G. von Schnering, and L. Walz, Liebigs Ann., 1996, 1055; R. Schwesinger and H. Schlemper, Angew. Chem., Int. Ed. Engl., 1987, 26, 1167. CrossRef
8. I. A. Koppel, R. Schwesinger, T. Breuer, P. Burk, K. Herodes, I. Koppel, I. Leito, and M. Mishima, J. Phys. Chem. A, 2001, 105, 9575. CrossRef
9. F. Ramirez, A. V. Patwardhan, H. J. Kugler, and C. P. Smith, J. Am. Chem. Soc., 1967, 89, 6276. CrossRef
10. K. B. Dillon, A. W. G. Platt, and T. C. Weddington, Inorg. Nucl. Chem. Lett., 1978, 14, 511. CrossRef
11. It is recommended that the reaction temperature be kept lower than 50 °C because 5 is gradually decomposed when the reaction temperature exceeds 50 °C.
12. Recrystallization of 2 from 3-methylbutan-2-one/heptane gave a clear needle-like crystal. The structure of 2 was unambiguously determined by single-crystal X-ray diffraction analysis. During the structure refinement of 2, highly disordered outer counter anions that cocrystallized in the single crystals could not be modeled properly. Thus, the data were corrected for the presence of disordered counter anions by using the SQUEEZE procedure. CCDC 1010957 contains the crystallographic data of this compound. The data can be obtained free of charge from The Cambridge Crystallographic Data Center via www.ccdc.cam.ac.uk/data_request/cif.