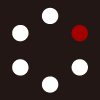
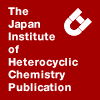
HETEROCYCLES
An International Journal for Reviews and Communications in Heterocyclic ChemistryWeb Edition ISSN: 1881-0942
Published online by The Japan Institute of Heterocyclic Chemistry
e-Journal
Full Text HTML
Received, 4th June, 2014, Accepted, 11th July, 2014, Published online, 4th August, 2014.
DOI: 10.3987/REV-14-SR(K)2
■ Light Absorption Spectral Patterns of Intact Garden Flowers in Relation to the Flower Colors and Anthocyanin Pigments
Norio Saito, Fumi Tatsuzawa,* and Toshio Honda
Laboratory of Olericultural and Floricultural Science, Faculty of Agriculture, Iwate University, 3-18-8 Ueda, Morioka, Iwate 020-8550, Japan
Abstract
Spectrophotometric studies were made on fresh flower petals of 139 species and/or cultivars in forty families on the basis of their absorption peaks in the visible light wavelength region. The absorption spectra measured were classified into mainly four flower groups according to the types of aglycones (anthocyanidins) and their absorption spectral patterns. The relationships between flower colors, absorption spectra and structures of anthocyanins were investigated for the above mentioned four flower groups. These investigations may give a clue to the chemical basis of color variation in the flowers.CONTENTS
Introduction
Results and Discussion
I. The three absorption peaks for the λmax A, B, and C of anthocyanidins in the light absorption spectral curves of polyacylated anthocyanins, platyconin, bletilla anthocyanin 1, and senecio pink anthocyanin 1
II. The first flower group: flowers comprised of delphinidin type aglycone as their principal anthocyanins
III. The second flower group: flowers comprised of cyanidin type aglycone as their principal anthocyanins
IV. The third flower group: flowers comprised of pelargonidin type aglycone as their principal anthocyanins
V. The fourth flower group: flowers having metal complex anthocyanins as their principal anthocyanins and also flowers showing characteristic absorption peaks in the long light wavelength region at 650 – 750 nm as their absorption spectra
Conclusion
INTRODUCTION
Anthocyanins are water-soluble pigments in the plant cell vacuoles that may appear red, purple, or blue depending on the various factors; such as their higher-order structures, the presence or absence of aromatic acids in their molecules, phenolic substances, metal ions, and pH. Most floral anthocyanins are derived from pelargonidin, cyanidin, and delphinidin, which differ only in the degree of hydroxylation in the B-ring and generally produce orange-red, magenta, and mauve colors, respectively (Figure 1).
Anthocyanin compositions in relation to the stabilization and variation of flower colors have widely been investigated, and more than 600 kinds of anthocyanins have been known to be present as flower color pigments.1-4
Anthocyanin pigments are generally stored in cell vacuoles of the flowers exhibiting a variety of flower colors. However, it is recognized that a discoloration phenomenon providing the corresponding colorless carbinol psuedobases (Figure 2) will be occurred with hydration of their basic skeleton, benzopyrilium ion, in most of isolated anthocyanins except for the polyacylated anthocyanins having extraordinary stack-structures and blue metal complex pigments (Figure 3), when anthocyanin pigments are dissolved in aqueous solutions.5-11 Recent studies on the stability of flower colors revealed that most of fresh flowers exhibiting weakly acidic or neutral in their cell saps are endowed with the mechanism for inhibition of the hydration to preserve their flower colors stably for relatively prolonged period of time. To explain the mechanism for prevention of discoloration phenomenon, the participations of inorganic ions and/or organic acids existed in intracellular solution of vacuoles, and also aromatic compounds bonded with anthocyanins have been advanced.2,5-11 It is also well recognized that the structures of anthocyanins are susceptible to the change of acidity (pH) of the solution providing a variety of tautomeric structures of anthocyanins (Figure 2).5-7 Actually, anthocyanins are able to show red colors by taking flavylium cation structure under acidic conditions and also show blue colors by taking ionized quinonoidal structures under alkaline conditions. These results are closely related to the fact that anthocyanins produce a wide range of colors depending on the humoral factor in the solution varying the region from acidic to alkaline.5-7,12,13
Taking account of the above humoral factors, it is reasonable to rationalize that the red color arising from the flavylium cation structure is preserved under strongly acidic conditions, though it is rather hard to understand the stability of the other tautomeric structures of anthocyanins under weakly acidic or neutral condition of vacuoles in the flowers.5,6 To demonstrate the stability of flower colors in the vacuoles, several mechanisms for the prevention of discoloration phenomenon have been proposed even under weakly acidic or neutral conditions. Although co-pigmentation had not previously been taken into account in stabilizing the flower colors, Robinson and Robinson advocated an intermolecular co-pigmentation phenomenon in 1931,14 in which polyphenols existed in the same vacuole, especially flavone, flavonol, and tannin derivatives, are associating with anthocyanins to stabilize the flower color and also to give rise to a bluing effect with bathochromic shift. Metal-complex theory stabilizing the flower color by making complex of anthocyanin with metal ions was proposed by Shibata and his coworkers in 1919.15 Moreover, Saito and his coworkers reported an intramolecular co-pigmentation of anthocyanins in 197216 (Figure 3). They discovered that more than two molecules of aromatic acids acylated with sugar moieties in the same molecule are associating with aglycones by making sandwich structures via stacking of the aromatic rings to preserve the flower colors by inhibition of hydration.7-9 In 1972, Asen also proposed a self-association mechanism for the stabilization of flower colors.17,18 Afterwards these mechanisms of metal-complex theory, co-pigmentation and self-association were advanced in detail with spectral methods by Goto and other groups.2,5-11
As mentioned above, it is essential to acquaint not only primary structures (plain structures) of anthocyanins but also secondary structures (tautomeric structures) (Figure 4) and higher-order structures including structural arrangement (stacking state, inter- and intramolecular co-pigmentation or self association, metal complex formation etc) (Figure 3) to understand the stability of flower colors in vacuoles.7-11 For the explication of flower color variations, the measurements of absorption spectra for the anthocyanin contained in the cell-tissues of flowers are well recognized as one of the important and effective methods.6,19-23
We have studied the flower color variations based on the structures of anthocyanins by using RHS Colour Chart and CIELAB system, and the latter values were obtained by using Colour Measuring and Difference Counting Meter as the standard reference for plant color identification.21 We have also investigated the mechanism of color variation responsible for anthocyanins in the flowers in relation to their structures by measuring the absorption spectra of fresh petals in the visible light wavelength region.
In this review article, we would like to report the light absorption spectra of anthocyanin-containing tissues from fresh flowers by the use of a double-beam instrument (Shimadzu Multi-purpose Recording Spectrophotometer, Type MPS-2450).21 Actually, we have measured the light absorption spectra of 40 families and 139 species and/or cultivars of flowers. At first, visual and colorimetric evaluation of the flower colors was taken place by measuring Royal Horticultural Society (RHS) Colour Chart. Code numbers of RHS Colour Chart matched with the flower colors investigated were recorded by comparing directly with the flower petals of the plants,21 and the corresponding hue were calculated on the basis of the chromaticity values (a* and b*). The distributions of flower colors were also shown on the CIE chromaticity diagram. On the other hand, spectral absorption curves of the intact tissues of flowers were directly measured on the intact petals using a recording spectrophotometer operated as double-beam instrument (360 - 750 nm) to obtain the absorption maxima (λmax) and their relative absorbance ratio, and the absorbance at the λmax was normalized to zero at 750 nm. The λmax value observed as a shoulder of absorption curve was reconfirmed by measuring a parallel differential spectrum.24 Results are summarized in Tables 2 - 5, where main anthocyanins with the binding sites of sugars and the presence or absence of acylated glycosides were also mentioned.
Among a number of flowers investigated in this study, two or three absorption peaks for the λmax (A, B and C) are observed in their absorption spectral curves, which stem from coexistence of several secondary tautomeric structures of anthocyanins (Figure 4).5,7,9
These two or three absorption peaks for the λmax are usually observed in the order of negatively ionized quinonoidal form (A), neutral quinonoidal form (B), flavylium cation structure (C), and unidentified structure (D) from the long wavelength region of the absorption spectral curves (Table 1, Figure 4, Chart 1; A-4’ = A, A4’ = B, AH+ = C).
In the most cases, the same primary anthocyanin structure affords a mixture of three types of secondary structures under weakly acidic or neutral conditions. Parallel to this phenomenon, several absorption peaks for their λmax (A – D) are observed in the absorption spectral curves of anthocyanin solutions and also fresh flowers. These λmax (A – D) are closely related to the structural features of aglycones, and are shown in Tables 2 – 5. In general, when the flowers have the same aglycone in the structures, their absorption peaks for the λmax (A – D) indicate the numerical value of an approximation.
Thus, it is reasonable to classify the flowers investigated here into three groups; such as delphinidin, cyanidin, and pelargonidin groups, according to their structural features of aglycones. However, we noticed that 21 species out of 139 flowers were not able to belong to the above three groups, therefore, these flowers were categorized into the fourth group, independently. Interestingly, the absorption spectra of the fourth group exhibited the typical anomalous λmax at near 650-750 nm which could not be observed in the absorption spectra of the normal flowers in the above three groups. We investigated here the relationships between flower colors, absorption spectra and structures of pigments of the above mentioned four groups.
RESULTS AND DISCUSSION
I. The three absorption peaks for the λmax A, B, and C of anthocyanidins in the light absorption spectral curves of polyacylated anthocyanins, platyconin, bletilla anthocyanin 1, and senecio pink anthocyanin 1.
In order to obtain the standard λmax values (reference values) of absorption spectra of anthocyanins in the aqueous solution at the visible wavelength region, three representative anthocyanins are selected as follows; such as platyconin9,16,25 to delphinidin type pigments, bletilla anthocyanin 19,26 to cyanidin type pigments, and senecio pink anthocyanin 19,27 to pelargonidin type pigments (Charts 1-1 ~ 1-3), since these pigments show relatively stable flower colors under weakly acidic or neutral conditions by making sandwich structures in vacuoles of the flower cell-tissues. The absorption spectra for these three types of pigments are measured in buffer solutions at pH 1.68 – 10.10, and the values of λmax correspond to the structures A, B, and C are obtained (Table 1). Three λmax values of A, B, and C for platyconin are detected at 618, 570, and 533 nm. In the same way, those for bletilla anthocyanin 1 are observed at 588, 546, and 510 nm, and those for senecio pink anthocyanin 1 at 564, 530, and 500 nm, respectively. The differences of the λmax values corresponding to the structures A between platyconin and bletilla anthocyanin 1, and also between bletilla anthocyanin 1 and senecio pink anthocyanin 1 are approximately 30 nm. The differences of the λmax values corresponding to the structures B are roughly 20 nm.
However, the λmax values corresponding to the structures C vary rather irregularly in their differences. For these anthocyanins, glycosylation and co-pigmentation are recognized to be important stabilizing modifications that help to maintain the anthocyanin in colored states. Considering the glycoside patterns of the three anthocyanidin types of pigments; such as 3,7-glycoside for platyconin and senecio pink anthocyanin 1, and 3,7,3’-glycoside for bletilla anthocyanin 1, their negatively ionized quinonoidal forms and neutral quinonoidal forms contribute to the λmax values of their A and B, respectively (Figure 4). The contributions to the observed λmax A and B may be stemmed from much more negatively ionized 4’-quinonoidal and neutral 4’-quinonoidal forms than 5-quinonoidal forms.5,6 On the contrary, because of none of glycosylation to 7-OH group of aglycone, the pigments bearing 3,5-glycoside or 3-glycoside, such as ipomoea red anthocyanin 1,28 are able to form 7-quinonoidal structure in addition to 4’- and 5-quinonoidal structures. Thus, it is difficult to identify the observed λmax with each structure, since the absorption spectra are generally observed as heavily overlapped spectral curves (Charts 1-1 ~ 1-4). The values of λmax corresponding to the structures of A, B, and C vary depending on pH values of the solution leading to the changes of their absorbance. The pigments with stabilized colors by making co-pigmentation are seriously implicated by their pH values. In fact, the flower color of bletilla anthocyanin 1 is extremely stable under neutral condition and exhibits typical three absorption peaks for the λmax of A, B, and C at pH 3.0 – 9.18 with little changes of the absorbance ratio (Chart 1-2).
In the case of senecio pink anthocyanin 1, three absorption peaks for the λmax A, B, and C are detected only at pH 5.6 – 7.4. In more acidic or more alkaline regions, the absorption spectrum of this pigment shows characteristic absorption curves exhibiting a single absorption peak corresponding to the λmax A or C, or similar absorption curves with a very weak shoulder corresponding to the λmax B as depicted in Charts 1-1 ~ 1-3. Thus, the analysis of the absorption spectra of anthocyanins may set up a standard way to understand the improvement for flower colors. Moreover, it will be possible to speculate the pH values in cell sap of fresh flowers based on the absorbance ratios of the λmax of A, B, and C.
II. The first flower group: flowers comprised of delphinidin type aglycone as their principal anthocyanins
Sixty-seven plants contain similar anthocyanidins (delphinidin type) having hydroxyl groups at 3, 5, 7, 3’, 4’, and 5’-positions of aglycone such as delphinidin, petunidin, malvidin, and hirsutidin as their main anthocyanins. These flowers are classified into the first flower color group as exhibiting similar color features (Table 2).
In general, it is recognized that at least one of phenolic hydroxy groups of anthocyanins in flowers is glycosylated with various types of sugars. Some pigments are present accompanying the sugars acylated with aromatic acids in plants. The observed λmax values of the methylated or glycosylated anthocyanidins are usually shifted to shorter wavelength regions than those of unsubstituted anthocyanidins. For the sake of clarifying, the positions of methylated or glycosylated hydroxy group are summarized in addition to the presence or absence of acylated sugars with aromatic acid in Table 2.
In this study the relationships between the absorption spectra and flower colors are first investigated by using 67 species and/or cultivars of flowers belonging to this group. As the reference data for comparison of absorption spectra, the measured values of platyconin were employed, where the λmax of negatively ionized quinonoidal form (A) appeared at 618 nm, neutral quinonoidai form (B) at 570 nm, and flavylium cation form (C) at 533 nm, respectively (Table 1, Chart 1-1). As the results of measurement of 67 flowers, their λmax for A, B, and C appear in the regions at 642 – 611 nm, 590 – 556 nm, and 555 – 531 nm, respectively. These values are closely related to those of platyconin within ±20 nm indicating the presence of the same structures for the anthocyanin pigmentation in these flowers. Again, it is reasonable to conjecture that the flower colors of these 67 flowers investigated here arise from the contribution of coexistence or alone of the secondary structures (A, B, and C) as similar to the case of platyconin.
It should be noted that the spectra of this group show a typical absorption peak or a shoulder peak at 510 – 500 nm, these peaks are not able to assign to any secondary structural feature, and simply summarized as the λmax for D in Tables.
This group is further divided into four sub-groups depending on the presence or absence of all three absorption peaks for the λmax A, B, and C, and also the difference of the intensities for their specific absorbance. These results are reflected in pH values of the vacuoles and also the stalwartness of co-pigmentation of anthocyanins in their flowers. For the sake of understanding the relationships between the absorption spectra and flower colors, the flower color data of these 67 flowers are obtained by the measurement with RHS Colour Chart and also colorimetric method. Their color data recorded by CIELAB system are exhibited on the CIE chromaticity diagram, a* and b* values in the diagram (Charts 2-1 ~ 5-3).
II-1: Flowers exhibiting blue to violet, and three absorption peaks for the λmax A, B, and C independently
Among the above 67 species and/or cultivars of flowers, 16 flowers exhibiting blue to violet-blue color are classified into this sub-group (Table 2, II-1). As the representative flower of this sub-group, we illustrate the absorption spectral curve of the blue flower of Heliophila coronopifolia in Chart 2-1-1, where three absorption peaks for the λmax A, B, and C are found to exist independently. It was recognized that the blue color of this flower was produced by the co-pigmentation of acylated delphinidin 3-sambubioside-5-glucoside with acylated kaempferol 3,4’-diglucoside-7-cellobioside.29 As noted above, all three absorption peaks for the λmax A, B, and C appear independently in the absorption spectral curves of this group flowers as similar to that of the solution of platyconin at pH 5.00 – 6.85. The flower color of platyconin is known to be stabilized by taking place an intramolecular co-pigmentation between anthocyanidin (delphinidin) and aromatic acid (caffeic acid). The similar phenomenon for anthocyanin coloration was reported in the blue flowers of Senecio curuentus,30,31 Leschenaultia ‘Violet Lena’,32 Aconitum spp.,33 Platycodon grandiflorus,16,25 Triteleia bridgesii,34 and Campanula medium.35,36 Moreover, another intra- or inter-molecular co-pigmentation between anthocyanidin and flavone or flavonol is also reported in the flowers of Heliophila coronopifolia,29 Allium ‘Blue Perfume’,37 Agapanthus ‘Blue Heaven’,38 and Primula ‘Julian Blue’.39 The distribution of flower colors of this sub-group (II-1) is shown on CIE chromaticity diagram in Chart 2-1-2, and is located in rather narrow blue color region as shown in its diagram. From these results, the flower colors of this sub-group show relatively stable colors of blue or violet-blue which are produced by the co-pigmentation.
II-2: Flowers exhibiting blue to violet-blue, and two absorption peaks for the λmax A and B
The absorption spectra of the flowers in this sub-group (II-2) also show three absorption peaks for the λmax A, B, and C as similar to those of sub-group II-1. However, the λmax for A or C is observed as a shoulder, which is the characteristic feature of the spectra of this sub-group. Among the 67 flowers investigated in this study, 19 flowers are classified into this sub-group, in which 12 flowers are arranged to the type as II-2-1, and 7 flowers are arranged to another type as II-2-2 depending upon their absorption peaks. As the typical example of II-2-1 bearing a shoulder for the λmax C, the absorption spectrum of the flowers of Clitoria ternatea40 is demonstrated in Chart 2-2-1A. On the other hand, the absorption spectral curve of the flowers of Tradescantia virginiana41 showing a shoulder for the λmax A is shown in Chart 2-2-1B as a typical example of II-2-2 type. As seen in the model case of platyconin (Chart 1-1), it is interesting to note that the absorption peak of the λmax C is only observed as a shoulder when the solution has > pH 6.85. Whereas, when the solution has < pH 4.0, the absorption peak for the λmax A is observed as a shoulder, or it sometimes disappears in the absorption spectral curve. These results suggested that the pH values of cell vacuoles of the flowers (II-2-1 type) are inclined to more alkaline region than those of the flowers (II-1 sub-group). On the contrary, the pH values of cell vacuoles of the flowers (II-2-2 type) tend to more acidic region than those of the flowers (II-1 sub-group). Again, this group of flowers also produces the anthocyanin pigmentation by forming mainly the co-pigmentation similar to the case of the flowers of II-1 group. The distribution of flower colors of this sub-group II-2 is shown on CIE chromaticity diagram in Chart 2-2-2, and their distribution area is spread more widely than that of the flower sub-group II-1. Furthermore, by comparing the flower colors of sub-group II-2-1 type with II-2-2 type, the distribution of group II-2-1 type is displaced somewhat to blue region than that of group II-2-2 type.
II-3: Flowers exhibiting blue to purple, and one strong peak for the λmax B or C with a weak absorption peak and a shoulder
The absorption spectra of this sub-group exhibiting one major absorption peak for the λmax together with other two very weak peaks or shoulders are quite different from those of the sub-group II-2, and 21 plants are classified to this sub-group. This sub-group is again arranged to II-3-1 and II-3-2 types in detail according to the types of their major λmax. Eleven flowers showing the major absorption peak for the λmax B belong to the type II-3-1, and 10 flowers exhibiting a major peak for the λmax C belong to the type II-3-2. As the typical example of II-3-1 type, the absorption spectral curve of the flowers of Torenia fournieri42 is shown in Chart 2-3-1A, and that of Dianthus caryophyllus ‘Moondust Velvet Blue’43 is demonstrated in Chart 2-3-1B as a typical example of II-3-2 type. In the absorption spectral curves of both type II-3-1 and II-3-2, the λmax for A are observed as only shoulders. From the results of absorbance ratio (B/C) in the absorption spectral curves of both II-3-1 and II-3-2 types, pH values of flower cell-vacuoles of the II-3-2 type are assumed to be inclined to rather acidic regions, and those of the II-3-2 type show rather stronger tendency for inclination than those of the II-3-1 type.
As the glycoside types occurred in flowers of the II-3-1 type are mainly 3,5-glycosides, and a mixture of 3,5-glycosides and 3-glycosides in those of the II-3-2 type, negatively ionized and/or neutral 7-quinonoidal structures appear in addition to 4’-quinonoidal structure as their secondary structures in both types. The mixture of these structures affords heavily overlapped and complicated broaden spectral curves to meet with difficulty for assignment of the λmax for A, B, and C, respectively. It is suggested based on the analysis of absorbance ratio from the absorption peaks for the λmax A, B, and C that the flower colors of the II-3-1 type are produced mostly due to the presence of neutral quinonoidal structure (B) together with some presence of flavylium cation form (C). On the contrary, the flower colors of the II-3-2 type are generated mainly due to the presence of flavylium cation form (C) in addition to some contribution of neutral quinonoidal structure (B). It is rationalized by assuming that the flower colors of the sub-group II-3 is preserved owing to not only the presence of co-pigmentation but also self-association mechanism especially for maintain the flavylium cation structures. As shown in Chart 2-3-2, the distribution of flower colors of this sub-group II-3 are more spread out from blue to purple region. Particularly the distribution of flower colors of the II-3-2 type is present in the more reddish region of violet or purple color because of increasing of the λmax C.
II-4: Flowers exhibiting only one absorption peak for the λmax A, B or C
The absorption spectra of flowers of this sub-group II-4 show finely simple bilateral symmetric curves, characteristically, as can be seen in the absorption spectral curve of Petunia x hybrida ‘Baccara Magenta’44 as a representative example (Chart 2-4-1), and 11 flowers belong to this sub-group (II-4). The flowers of this sub-group usually exhibit reddish colors except for Parochetus communis,44 and their spectral curves exhibit only one absorption peak corresponding to the λmax B or C at 564 – 534 nm without any absorption shoulder. Since it is difficult to assign the observed λmax for B or C, the flowers are tentatively sorted to two types as a rough indication. The flower colors of the type II-4-1 showing the λmax at shorter wavelength than 548 nm is considered to be produced on the flavylium cation form (C). The other type II-4-2 showing the λmax at longer wavelength than 556 nm is considered based on the neutral quinonoidal form (B) (see in Table 2). As mentioned above as an exception, the spectrum of the flowers of Parochetus communis shows the λmax at 603 nm, which is presumed to be derived from the negatively ionized quinonoidal form (A). Therefore, its vacuole is assumed to indicate relatively strong alkaline as the same as the cases of Ipomoea nil,45 I. tricolor,46 and Strongylodon macrobotrys,47 however, there is no report on the pH study of this flower, unfortunately. The major glycoside of this sub-group II-4 is 3-glycoside type which is much simpler than that of the sub-group II-3. Since the hydroxy groups at 5, 7 and 4’-positions are free from glycosides, the absorption spectral curves of this sub-group are produced by the mixture of 4’-, 7- and/or 5-quinonoidal structures in addition to the flavylium cation form. Therefore, their absorption spectral curves are observed as one heavily overlapped spectral curve.
It is also rationalized by assuming that the flower colors of this sub-group are stabilized by forming a relatively unpunctual weak intermolecular co-pigmentation and/or self-association because of the absence of intricately acylated sugars with aromatic acids on the anthocyanin for this sub-group. The distribution of flower colors of this sub-group II-4 is shown on CIE chromaticity diagram in Chart 2-4-2. As shown in this diagram, their distribution is shifted to most reddish regions in this group II. Thus, the flowers of this sub-group show from purple-violet to red-purple color with an exception of the blue flower color of Parochetus communis. This shift to reddish region may be responsible for the increase of the flavylium cation form (C) in their flowers.
III. The second flower group: flowers comprised of cyanidin type aglycone as their principal anthocyanins
The anthocyanins having hydroxy groups at 3, 5, 7, 3’, and 4’-positions of aglycones such as cyanidin and peonidin, are classified to the floral pigments of this second flower group. Thirty-eight flowers belong to this group, and the relationships between their absorption spectra and flower colors are investigated as above (Table 3).
As the reference data for comparison with the absorption spectra of flowers in this group, the absorption spectral data of bletilla anthocyanin 1 in the buffer solution were employed just as those of platyconin for the first flower group (Table 1). The purple-red flower color of bletilla anthocyanin 1, one of the most stable polyacylated anthocyanins, is believed to be stabilized by making an intense co-pigmentation via a vertical stacking process (higher-order structure).9,26,48 The results of the absorption spectral measurement for the flowers of this group are summarized in Table 3.
For bletilla anthocyanin 1, three absorption peaks for the λmax A, B, and C corresponding to the negatively ionized quinonoidal form (A), neutral quinonoidal form (B), and flavylium cation form (C) appear at 588, 546, and 510 nm, and those of the second flower group appear at 605 – 570, 564 – 520, and 532 – 486 nm, respectively. The observed differences in the λmax values are almost the same as those of platyconin, a delphinidin type anthocyanin, within ±20 nm. Based on these results, the flowers of this second group are further classified into three sub-groups (III-1, III-2, and III-3) according to the particular absorption spectral patterns of the λmax A, B, and C.
III-1: Flowers exhibiting violet-blue to purple, and three or two absorption peaks for the λmax A, B and/or C
Flowers of nine species and/or cultivars are classified into this sub-group III-1 (Table 3), and two absorption spectral peak patterns are observed in these flowers. These flowers are further divided into III-1-1 and III-1-2 types. As the former III-1-1 type, 6 flowers clearly show three absorption spectral peaks for the λmax corresponding to A, B, and C independently, and the typical absorption spectral curve of Bletilla striata26 is demonstrated in Chart 3-1-1A. For the later III-1-2 type, three flowers are grouped and recognized to exhibit two absorption spectral peaks corresponding to the λmax A and B, and a shoulder for the λmax C. In Chart 3-1-1B, the spectral curve of Aubrieta xcultorum ‘Royal Violet’49 is shown. In the III-1-1 type, the flowers of Bletilla striata contain bletilla anthocyanin 1 as its main anthocyanin pigment, and other flowers of this type comprised of polyacylated anthocyanins, intensely associated with an intramolecular co-pigmentation, as the major anthocyanins.9
In the III-1-2 type, the flowers of Lobelia erinus50 and Anemone coronaria ‘St. Brigid’51 containing polyacylated anthocyanins as the major pigments, are believed to form an intramolecular co-pigmentation. However, the flowers of Aubrieta xcultorum ‘Royal Violet’ containing acylated anthocyanins (acylated with only one molecule of aromatic acid) as its major pigments, are recognized to form an intermolecular co-pigmentation with flavonol.49 Both intra- and intermolecular co-pigmentations are observed to maintain stable flower colors in flowers of the III-1-2 type. The distribution of flower colors of this sub-group III-1 is shown on CIE chromaticity diagram (Chart 3-1-2). Their flower colors distribute in the region of violet-blue to purple.
III-2: Flowers exhibiting violet-blue to orange-red, and only one absorption spectral peak for the λmax B or C with shoulders
Thirteen flowers are grouped into the sub-group III-2 as shown in Table 3, and their absorption spectral peak show only one high absorption peak for the λmax B or C with two shoulders. Eleven flowers from this sub-group having one absorption peak for the λmax B together with two shoulders for A and C are further divided as III-2-1 type, in which the flowers of Orychophragmus violaceus52 are involved and its absorption spectral curve is shown in Chart 3-2-1A.
On the other hand, 2 flowers showing an absorption spectral peak for the λmax C together with two shoulders corresponding to the λmax A and B are put into the same type of III-2-2, in which the flowers of Lunaria annua53 are involved and its spectral curve is demonstrated in Chart 3-2-1B.
It is noteworthy that the major glycosides in the flowers of the sub-groups III-1 and III-2 are mainly reported to be 3,7,3’-glycosides and 3,5-glycosides, respectively (Table 3). As mentioned in the case of the sub-group II-3, the flowers of the sub-group III-2 also contain negatively ionized and/or neutral 7-quinonoidal forms in addition to 4’-quinonoidal form as their secondary structures. Again, the mixture of these secondary structures affords heavily overlapped and complicated broaden absorption spectral curves. The spectral features of III-2-2 type of flowers are mainly based on the λmax C of the flavylium cation form, therefore, the cell vacuole in this type of flowers is assumed to be rather acidic.
The stable flower colors of this sub-group III-2 are thought to be maintained by forming an intramolecular co-pigmentation, since the flowers are comprised of polyacylated anthocyanins having several aromatic acids in their molecules. However, the acylation of aromatic acids is mainly restricted to take place with sugars which are present at the 3-position of anthocyanidin, except for some flowers in this sub-group. Thus, the sandwich-like stacking effect of this sub-group for the co-pigmentation is estimated to be a bit weaker than the case of III-1 possessing acylated sugars both at 7- and 3’-positions.9 In Chart 3-2-2, the flower color distribution of this sub-group III-2 are indicated to be from the violet-blue to red regions except for orange-red flowers of Sophronitis coccinea in which carotenoid pigments are contained.54,55 The distribution pattern of the sub-group III-2 is similar to that of the sub-group III-1 (Chart 3-1-2) with slight displacement to red color region in the diagram.
III-3: Flowers exhibiting only one absorption spectral peak for the λmax A, B or C
Flowers of sixteen species and/or cultivars are grouped to this sub-group III-3, and the characteristic features of their absorption spectra are observed as the simple bilateral symmetric curves without accompanying any shoulder peaks, as similar to those of the sub-group II-4 (Chart 2-4-1). Their absorption spectral curves showing only one peak for the λmax are quite different from those of sub-groups III-1 and III-2. Furthermore, the flowers of this sub-group III-3 are divided into three types III-3-1, III-3-2, and III-3-3, dependent on the wavelength values of their λmax (see Table 3). The flowers showing the λmax corresponding to negatively ionized quinonoidal form (A) are grouped as the III-3-1 type involving 2 species of Ipomoea flowers, I. tricolor56,57 and I. purpurea.58 Similarly, the flowers showing the λmax corresponding to neutral quinonoidal form (B) and flavylium cation form (C) are grouped as III-3-2 and III-3-3 types, respectively. It is important to note that the flowers of III-3-1 type contain simple 3,5-glycosides, and those of III-3-2 and III-3-3 types have much simpler 3-glycosides. Moreover, the heavily acylated anthocyanins with aromatic acids does not take place in flowers of this sub-group III-3 except for Ipomoea plants. These facts suggested that the flower colors of this sub-group III-3 are stabilized by forming both an intermolecular co-pigmentation and/or self-association just like the case of the sub-group II-4. This assumption is supported by the isolation of the pigments, genuine red anthocyanins, possessing a quinonoidal forms of cyanin and pelargonidin from the deep red flowers of Rosa hybrid, Centaurea cyanus, and Dahlia variabilis, without the use of acidic solvents.59,60 Again, the difficulty is encountered to assign each absorption peak corresponding to the λmax A, B, or C in the absorption spectral curves of this sub-group, since the mixture of the structures due to 7- and 5-quinonoidal forms in addition to 4’-quinonoidal form affords heavily overlapped and complicated broaden absorption spectral curves. The flowers of Ipomoea tricolor belong to the III-3-1 type, and its absorption spectrum is demonstrated in Chart 3-3-1A as a typical example of this III-3-1 type of flowers. Both flowers of genus Ipomoea plants comprise of polyacylated anthocyanins,56-58 and the vacuoles of I. tricolor and I. nil indicate strong alkaline.45,46 Thus, the absorption spectrum of I. tricolor is observed as just a bit of desymmetrized curves at the short wavelength region. Although the observed peak of the λmax is obviously due to the structure A, some contribution of the structures B and C is also imagined for this phenomenon in its absorption spectral curve.
In the absorption spectral curves of flowers of the III-3-2 type, the λmax corresponding to B are found at 554 – 532 nm, and the spectral curve of Gloriosa rothschildiana61 is shown in Chart 3-3-1B as the representative curve of this type. Interestingly, the flower colors of Leschenaultia formosa ‘Red Splash’62 and Disa ‘Sid Cywes Marlene’63 are known to be affected by the presence of carotenoids.
The spectrum of Petunia x hybrida ‘Baccara Pink’64 is demonstrated in Chart 3-3-1C as a typical example of the III-3-3 type of 7 flowers, in which the λmax corresponding to C is found as similar to the case of the sub-group II-4. The flower vacuoles of III-3-3 type is thus conceivable to be acidic, and the same mechanism for preservation of the flower colors, an intermolecular co-pigmentation and/or self-association, is considered to take place as in the case of the sub-group II-4. The flower color distribution is shown on CIE chromaticity diagram, and their flower colors are present in the region of purple-red to red except for three flower colors of I. tricolor (blue), I. purpurea (violet-blue), and Malcolmia maritima (purple-violet).65 The flower color location of the sub-group III-3 is situated in the most reddish color regions in the group III except for the above three flowers (Chart 3-3-2).
IV. The third flower group: flowers comprised of pelargonidin type aglycone as their principal anthocyanins
The anthocyanins having hydroxy groups at 3, 5, 7, and 4’-positions of aglycones, such as pelargonidin, are classified to the floral pigments of this group IV, and 13 flowers belong to this group (Table 4) in this study. As discussed in the section I, the spectral data of senecio pink anthocyanin 1 is employed as the reference data where the absorption spectral peak for the λmax A appears at 564 nm, together with the λmax for B and C at 530 and 500 nm, respectively, for comparison of the absorption spectra of this group. Actually, three absorption peaks of this pelargonidin type flowers appear in the regions at 577 – 558 nm, 542 – 510 nm, and 518 – 488 nm for the λmax A, B, and C, respectively (Table 4). These varying values are closely related to those of delphinidin-type flowers and also cyanidin-type flowers within ±20 nm difference from their reference data indicating the presence of pelargonidin structure as the aglycones for their main anthocyanin pigments in these flowers. Thirteen flowers of this group are further classified into two sub-groups according to the absorption peak pattern of the λmax for A, B, and C. The sub-group IV-1 includes 4 flowers which show three absorption peaks for the λmax A, B, and C, separately, or at least one peak and two shoulders in their absorption spectral curves. As a typical example of this sub-group, the absorption spectral curve of Campanula medium ‘May Pink’36 is shown in Chart 4-1-1. The similar spectral curve is also demonstrated for the flowers of Senecio cruentus27 (Table 4).
Both flowers in this sub-group IV-1 comprise of polyacylated anthocyanins with the glycoside type of 3,7-glycosides. Other 2 flowers of this sub-group have 3,5-glycoside instead of 3,7-glycoside, and two shoulders and one peak for the λmax A, B, or C are observed in their absorption spectral curves. The glycoside types of the sub-group IV-2 consisting of 9 flowers is mainly a simple 3-glycoside, and the broaden absorption band with only one peak for the λmax B or C at 550 – 450 nm is observed as the characteristic feature for the absorption spectral curve in this sub-group flowers just as similar to the cases of the sub-groups II-4 and III-3. As a typical example of this sub-group IV-2, the absorption spectral curve of Anemone coronaris ‘St. Brigid Red’66 is shown in Chart 4-2-1. In the sub-group IV-2, nine flowers are further subdivided into two types (IV-2-1 and IV-2-2) according to their observed λmax corresponding to B or C. The flowers having the λmax at 541 – 520 nm for the λmax B are grouped as the IV-2-1 type, and 7 flowers belong to this type.
Whereas, the flowers having the λmax for C at 511 nm are grouped as the IV-2-2 type, and 2 flowers belong to this type. It may be considered that the contribution of both intermolecular co-pigmentation and/or self-association is a main factor for the stabilization of flower colors in the anthocyanin pigmentation of this sub-group IV-2, since the major glycoside types of this sub-group are simple 3-glycisides, and reasonably the same phenomena are also recognized in the flowers of sub-group II-4 and III-3 having 3-glycoside type as the major glycoside type. In Charts 4-1-2 and 4-2-2, the distribution of flower colors of this group IV is shown on CIE chromaticity diagrams. The flower colors of the sub-group IV-1 are distributed mainly in red-purple, and those of the sub-group IV-2 distribute mainly in red.
V. The fourth flower group: flowers having metal complex anthocyanins as their principal anthocyanins, and also flowers showing characteristic absorption peaks in the long wavelength region at 650 – 750 nm as their absorption spectra
We discussed above the relationships between flower colors, absorption spectra and structures of pigments in the flowers, particularly by focusing on absorption spectral peaks for the λmax A, B, and C and flower colors according to three structural features of aglycones; such as delphinidin, cyanidin, and pelargonidin types. However, as exceptional cases, flowers of 21 out of 139 species and/or cultivars in this study can’t be classified into the above three groups. Since those flowers exhibit an anomalous λmax at 650 – 750 nm (corresponding to S) in their absorption spectral curves, they are classified as V group. Flowers of this group are further divided into three sub-groups, in which flowers containing metalloanthocyanins (metal complex pigments) as main anthocyanins are grouped as the sub-group V-1. Moreover, the flowers of the sub-group V-2 are based on cyanidin-type pigments but their flowers exhibiting the λmax exceptionally shifted (>50 nm) to longer wavelength region than normal cyanidin type pigments. The flowers belonging to the sub-group V-3 exhibit the λmax at 670 – 706 nm as those of chlorophyll pigments, however, the experiments for confirmation of reproducibility was not attempted here.
V-1: Flowers containing metalloanthocyanins
Table 5 indicates the major anthocyanins, flower colors, λmax of absorption spectra, and their relative absorbance from intact fresh petals of this sub-group (V-1). In these flowers, the presences of metal complex pigments are well known to play an important role for their flower coloration.10,67 In the absorption spectral curves of these flowers, a highly similar common spectral pattern is observed (Charts 5-1-1A ~ 1E) except for that of Hydrangea macrophylla.19,68,69 For example, the flowers of Salvia patens10,67,70 show the λmax at 719 nm (corresponding to S), and in the same manner, those of Nemophila menziesii67 and Centaurea cyanus10,71,72 are found at 715 and 681 nm, respectively. However, the λmax of the flowers of Commelina communis73,74 appears typically at much shorter wavelength, 646 nm. On the contrary, the λmax of the flowers of Hydrangea macrophylla is observed only at 603 – 582 nm as a broaden absorption band peak. Interestingly, this band expands to the long wavelength region of ca. 660 nm without showing a special absorption peak or shoulder corresponding to the λmax S.
The distribution of flower colors of the sub-group V-1 is shown on CIE chromaticity diagram in Chart 5-1-2. On the diagram, their flower colors locate in rather narrow blue regions.
V-2: Flowers containing cyanidin type anthocyanins but exhibiting strong blue colors
The four flowers of this sub-group are based on the cyanidin-type pigments. However, the λmax for A, B, and C of this sub-group are shifted to long wavelength region with 40 – 80 nm compared to those of the ordinary cyanidin-type of the III group. As a representative flower of this sub-group belonging 4 flowers, the absorption spectral curve of Corydalis flexuosa ‘Blue Panda’75 is shown in Chart 5-2-1. In the spectral curves of this sub-group, three absorption peaks for the λmax A, B, and C are observed at 675 – 645, 618 - 599, and 594 – 562 nm, respectively, and those values are obviously shifted to longer wavelength regions than those of bletilla anthocyanin 1 observed at 588, 546, and 510 nm. The similar phenomenon which confers on an additional factor for blueness was scrutinized by Bloor in 1997 as follows.76 When a polyacylated anthocyanin, ceanothus anthocyanin 2, isolated from the flowers of Ceanothus papillosus, was treated with octuple molar concentration of kaempferol 3-xylosylrhamnoside as a co-pigment, the peak of the λmax for A of the original pigment was shifted from 618 nm to 680 nm. Similarly, the λmax for B and C were shifted from 576 to 612 nm, and 536 to 570 nm, respectively. He proposed the reason for this extraordinary wavelength shift by the formation of supramolecular complex between the delphinidin-type anthocyanin and flavonol as a copigment. It was also investigated by Yoshida’s group77 that the blue colors of the flowers of Meconopsis betonicifolia and M. grandis are arising from a formation of metal complex between the cyanidin-type anthocyanins and a copigment, kaempferol glycoside, where Fe3+ and Mg2+ ions are essential and play important roles for the blue color development.
They also proposed that this type of metal complex pigment is different from a stoichiometric supramolecular pigment such as commelinin or protocyanin. It is also speculated that the conformation of anthocyanidin and copigment in this group of pigments will be one of the most important factors for bluing of the flowers.10,71,74 In any case, a further progress will be necessary to understand the blue color pigmentation in more detail. On the CIE chromaticity diagram, the flower color distribution of this sub-group V-2 is shown in Chart 5-2-2. Their flower colors are located in most limited blue color regions in this study.
V-3: Other miscellaneous flowers exhibiting absorption peaks or shoulders at 665 - 706 nm
Twelve flowers belong to this sub-group V-3, and the visible absorption spectra of this group exhibit a unique λmax or shoulder of S at 665-706 nm. In Chart 5-3-1, the spectral curve of the flowers of Muscari arumeniacum78 is illustrated. Usually, the similar spectral curves bearing the λmax or a shoulder corresponding to S are observed for the flowers in early spring and/or just started blooming with a lack of reproducible results. This λmax disappear with advancement in aging of the flowers as an example for the λmax of Lechenaultia formosa ‘Red Splash’62 (see Table 3, III-3-2 and Table 5, V-3), and this phenomenon is supposed to be related to discoloration of chlorophyll pigments. However, we did not confirm the presence of chlorophyll pigments in this study. In addition to nine flowers containing common anthocyanidin type pigments, three flowers of genus Alstroemeria containing 6-OH anthocyanidin type pigments79-81 are grouped in this sub-group due to exhibition of the similar λmax S. For the absorption spectral curves of three Alstroemeria flowers, their major λmax for C are characteristically shifted to shorter wavelength region with 10 – 20 nm than those of flowers comprised of common anthocyanin without 6-OH group. The color distribution of sub-group V-3 is shown by plotting CIE color co-ordinates, a* and b* values in the diagram (Chart 5-3-2).
CONCLUSION
We have studied the flower color variations based on the measurement of visible absorption spectra (360 – 750 nm) of fresh petals of 139 species and/or cultivars (40 families). We have also investigated the mechanism of color development and protection for anthocyanins in the flower tissue-cells in relation to their secondary or higher structures.
Number of absorption spectral peaks and shoulders, and their relative absorbance ratios observed in the absorption spectral curves are taken account of the investigation on flower color variations.
Actually, the flowers investigated are categorized into three groups; such as delphinidin, cyanidin, and pelargonidin-types, according to their structural features of aglycones.
As a typical example of delphinidin-type pigments, the absorption spectra of platyconin were measured in the buffer solutions pH 1.68 – 10.1 to obtain the reference values. The λmax values of secondary anthocyanin forms corresponding to negatively ionized quinonoidal form (A), neutral quinonoidal form (B) and flavylium cation form (C) appeared at 618, 570 and 533 nm, respectively, in the spectrum of platyconin. Similarly, those for bletilla anthocyanin 1, as a representative of cyanidin-type pigments, appeared at 588, 546 and 510 nm, and those for senecio pink anthocyanin 1, as a representative of pelargonidin-type pigments, are observed at 564, 530 and 500 nm, respectively.
In the fresh flowers, the λmax of A for 67 delphinidin-type flowers appear at 642 – 611 nm, and those of B and C are found at 590 – 556 nm and 553 – 531 nm, respectively. Similarly, the λmax of A for 39 cyainidin-type flowers appear at 605 – 570 nm, and those of B and C are found at 564 – 520 nm and 532 – 510 nm, respectively. Moreover, the λmax of A for 13 pelargonidin-type flowers are observed at 577 – 558 nm, and those of B and C appear at 542 – 520 nm and 515 – 486 nm, respectively.
The observed λmax values for these flowers investigated above are closely related to those of the reference pigments; such as platyconin, bletilla anthocyanin 1, and and senecio pink anthocyanin 1, within ±20 nm.
However, other 21 flowers out of 139 flowers were unable to belong into above three groups due to the appearance of the unusual λmax corresponding to S at > 645 nm. Thus, these flowers were classified as another group for the sake of convenience.
In common, the anthocyanin pigmentations of the flowers of the above three groups having different types of anthocyanidin pigments; such as delphinidin, cyanidin, and pelargonidin, are mainly produced by the formation of intra- and/or inter-molecular co-pigmentations in their flower tissue-cells, and their absorption spectra are highly susceptible to the secondary structures of the anthocyanins in the cell sap. Thus, the above three anthocyanidin type groups are further classified into sub-groups according to the occurrence types of absorption spectral peaks for the λmax of A, B, and C, especially on their three relative absorbance ratios. In general, the tendency toward the appearance of the λmax A, B, and C depends on the intensity of co-pigmentation in the flower tissue-cells, pH values, type of glycosides, the presence or absence of acylated sugars with aromatic acids etc. By this investigation, it is concluded that the anthocyanin pigmentation of the flowers whose tissue-cells inclined toward somewhat acidic, is consistent with the formation of self-association in addition to intra- and inter-molecular co-pigmentations because only one strong absorption spectral peak for the λmax B or C is observed in these flowers.
On the other hand, the flowers showing the λmax corresponding to S at > 645 nm are classified as different group from the above three groups. Among them, 5 flowers comprised of the metal complex anthocyanins are grouped as the sub-group V-1. Again, the characteristic spectral feature of the flowers of this sub-group is the occurrence of the λmax corresponding to S at > 645 nm, except for the flowers of Hydrangea macrophylla. Although the flowers of V-2 sub-group comprise of cyanidin-type anthocyanins as same as the flowers of III group, the λmax for A, B, and C are larger than those of III group, and shifted to long wavelength side with ca. 60 nm. In this sub-group, the flowers of genus Meconopsis and Corydalis demonstrating characteristic blue color pigmentations are included.
The flowers exhibiting a weak λmax or shoulders at 665 - 706 nm in their absorption spectral curves are included in the sub-group V-3, and 3 flowers of genus Alstroemeria possessing 6-hydroxy group in anthocyanidins are classified into this sub-group. The observed λmax or shoulders appear in almost the same region of those of chlorophyll pigments. These λmax are usually not found in the spectra of the aged flowers. Moreover, the appearance of the λmax is depending on the seasons.
ACKNOWLEDGEMENTS
This work was supported in part by a Grant-in-Aid for Scientific Research (C) [No.25450037, to F.T.] from the Japan Society for the Promotion of Science (JSPS).
References
1. J. B. Harborne and H. Baxter, The Handbook of Natural Flavonoids, Vol. 2, 1999, Wiley, Chichester.
2. Ø. M. Andersen and M. Jordheim, The Anthocyanins. In “Flavonoids, Chemistry, Biochemistry and Applications” eds. by Ø. M. Andersen and K. R. Markham, pp. 471-551, 2006, Chapman & Hall, London.
3. N. C. Veitch and R. J. Grayer, Nat. Prod. Rep., 2008, 25, 555. CrossRef
4. N. C. Veitch and R. J. Grayer, Nat. Prod. Rep., 2011, 28, 1626. CrossRef
5. R. Brouillard, Chemical Structure of Anthocyanin. In “Anthocyanins as Food Colors” ed. by P. Markakis, pp. 1-40, 1982, Academic Press, New York.
6. R. Brouillard, Flavonoids and Flower Colour. In “The Flavonoids, Advances in Research since 1980”, ed. by J. B. Harborne, pp. 525-538, 1980, Chapman & Hall, London.
7. R. Brouillard and O. Dongles, Flavonoids and Flower Colour. In “The Flavonoids, Advances in Research since 1980”, ed. by J. B. Harborne, pp. 565-588, 1988, Chapman & Hall, London.
8. T. Goto and T. Kondo, Angew. Chem., Int. Ed. Engl., 1991, 30, 17. CrossRef
9. T. Honda and N. Saito, Heterocycles, 2002, 56, 633. CrossRef
10. K. Takeda, Proc. Japan. Acad., 2006, 82, 142. CrossRef
11. K. Yoshida, M. Mori, and T. Kondo, Nat. Prod. Rep., 2009, 26, 884. CrossRef
12. R. Willstätter and A. E. Everest, Justus Liebig Ann. Chem., 1913, 401, 189. CrossRef
13. R. Willstätter and H. Mallison, Justus Liebig Ann. Chem., 1915, 408, 147. CrossRef
14. G. M. Robinson and R. Robinson, Biochem. J., 1931, 25, 1687.
15. K. Shibata, Y. Shibata, and I. Kashiwagi, J. Am. Chem. Soc., 1919, 41, 208. CrossRef
16. N. Saito, Y. Osawa, and K. Hayashi, Bot. Mag. Tokyo, 1972, 87, 33.
17. S. Asen, R. N. Stewart, and K. H. Norris, Phytochemistry, 1970, 9, 619. CrossRef
18. S. Asen, R. N. Stewart, and K. H. Norris, Phytochemistry, 1972, 11, 1139. CrossRef
19. N. Saito, Phytochemistry, 1967, 6, 1013. CrossRef
20. T. O. M. Nakayama and J. J. Powers, Absorption Spectra of Anthocyanin in Vivo. In “The Chemistry of Plant Pigments” ed. by C. O. Chichester, pp. 193-199, 1972, Academic Press, New York.
21. M. Yokoi and N. Saito, Phytochemistry, 1973, 12, 1783. CrossRef
22. R. N. Stewart, S. Asen, K. H. Norris, and D. R. Massie, Amer. J. Bot., 1969, 56, 227. CrossRef
23. N. Ishikura, Plant Cell Physiol., 1978, 19, 887.
24. K. Shibata, Zoku-Seibutsu-Butsurigaku-koza, 1968, Vol. 1, pp. 77-141.
25. T. Goto, T. Kondo, H. Tamura, K. Kawahori, and H. Hattori, Tetrahedron Lett., 1983, 24, 2181. CrossRef
26. N. Saito, M. Ku, F. Tatsuzawa, T. S. Lu, M. Yokoi, A. Shigihara, and T. Honda, Phytochemistry, 1995, 40, 1523. CrossRef
27. K. Toki, N. Saito, H. Kuwano, A. Shigihara, and T. Honda, Phytochemistry, 1995, 38, 1523.
28. N. Saito, F. Tatsuzawa, M. Yokoi, K. Kasahara, S. Iida, A. Shigihara, and T. Honda, Phytochemistry, 1996, 43, 1365. CrossRef
29. N. Saito, F. Tatsuzawa, K. Toki, K. Shinoda, A. Shigihara, and T. Honda, Phytochemistry, 2011, 72, 2219. CrossRef
30. K. Yoshitama, K. Hayashi, K. Abe, and H. Kakisawa, Bot. Mag. Tokyo, 1975, 88, 213. CrossRef
31. T. Goto, T. Kondo, T. Kawai, and H. Tamura, Tetrahedron Lett., 1984, 25, 6021. CrossRef
32. N. Saito, F. Tatsuzawa, Y. Yazaki, A. Shigihara, and T. Honda, Phytochemistry, 2007, 68, 673. CrossRef
33. K. Takeda, S. Sato, H. Kobayashi, Y. Kanaitsuka, M. Ueno, T. Kinoshita, H. Tazaki, and T. Fujimori, Phytochemistry, 1994, 36, 613. CrossRef
34. K. Toki, N. Saito, and T. Honda, Phytochemistry, 1998, 48, 729. CrossRef
35. N. Terahara, K. Toki, N. Saito, T. Honda, I. Isono, H. Furumoto, and Y. Kontani, J. Chem. Soc., Perkin Trans. 1, 1990, 3327. CrossRef
36. K. Toki, N. Saito, H. Nishi, F. Tatsuzawa, A. Shigihara, and T. Honda, Heterocycles, 2009, 77, 401. CrossRef
37. N. Saito, M. Nakamura, K. Shinoda, N. Murata, T. Kanazawa, K. Kato, K. Toki, H. Kasai, T. Honda, and F. Tatsuzawa, Phytochemistry, 2012, 80, 99. CrossRef
38. S. J. Bloor and R. Falshaw, Phytochemistry, 2000, 53, 575. CrossRef
39. N. Saito, K. Yoda, H. Haruyama, H. Kuwano, and T. Honda, Heterocycles, 1990, 30, 759. CrossRef
40. N. Saito, K. Abe, T. Honda, C. F. Timberlake, and P. Bridle, Phytochemistry, 1985, 24, 1583.
41. F. Tatsuzawa, N. Saito, K. Maeyama, M. Yokoi, A. Shigihara, and T. Honda, Heterocycles, 2010, 81, 2257. CrossRef
42. T. Endo, Jap. J. Genet., 1962, 37, 284. CrossRef
43. Y. Fukui, Y. Tanaka, T. Kasumi, T. Iwashita, and K. Nomoto, Phytochemistry, 2003, 63, 15. CrossRef
44. F. Tatsuzawa, unpublished results.
45. T. Yamaguchi, S. Fukuda-Tanaka, Y. Tanagaki, N. Saito, K. Yonekura-Sakakibara, Y. Tanaka, T. Kusumi, and S. Iida, Plant Cell Physiol., 2001, 42, 451. CrossRef
46. K. Yoshida, K. Kondo, Y. Okazaki, and K. Kato, Nature, 1995, 373, 291. CrossRef
47. K. Takeda, A. Fujii, Y. Senda, and T. Iwashina, Biochem. Syst. Ecol., 2010, 38, 630. CrossRef
48. P. Figueiredo, F. George, F. Tatsuzawa, K. Toki, N. Saito, and R. Brouillard, Phytochemistry, 1999, 51, 125. CrossRef
49. F. Tatsuzawa, Y. Aiba, T. Morino, N. Saito, K. Shinoda, K. Kato, K. Toki, and T. Honda, J. Japan. Soc. Hort. Sci., 2012, 81, 275. CrossRef
50. N. Saito, K. Toki, H. Kuwano, H. Moriyama, A. Shigihara, and T. Honda, Phytochemistry, 1995, 39, 423. CrossRef
51. K. Toki, N. Saito, A. Shigihara, and T. Honda, Heterocycles, 2003, 60, 345. CrossRef
52. T. Honda, F. Tatsuzawa, N. Kobayashi, H. kasai, S. Nagumo, A. Shigihara, and N. Saito, Phytochemistry, 2005, 66, 1844. CrossRef
53. F. Tatsuzawa, N. Saito, K. Shinoda, A. Shigihara, and T. Honda, Phytochemistry, 2006, 67, 1287. CrossRef
54. F. Tatsuzawa, N. Saito, M. Yokoi, A. Shigihara, and T. Honda, Phytochemistry, 1998, 49, 869. CrossRef
55. F. Tatsuzawa, N. Saito, T. Yukawa, T. Honda, K. Shinoda, K. Kato, and K. Miyoshi, J. Japan. Soc. Hort. Sci., 2014, 83, 64. CrossRef
56. T. Kondo, T. Kawai, H. Tamura, and T. Goto, Tetrahedron Lett., 1987, 28, 2273. CrossRef
57. T. S. Lu, N. Saito, M. Yokoi, A. Shigihara, and T. Honda, Phytochemistry, 1992, 31, 659. CrossRef
58. N. Saito, F. Tstsuzawa, K. Yoda, K. Kasahara, S. Iida, A. Shigihara, and T. Honda, Phytochemistry, 1995, 40, 1283. CrossRef
59. N. Saito, K. Hirata, R. Hotta, and K. Hayashi, Proc. Japan Acad., 1964, 40, 516.
60. K. Takeda, N. Saito, and K. Hayashi, Proc. Japan Acad., 1968, 44, 352.
61. Y. Sakaguchi and T. Takamura, Hort. Res. (Japan), 2008, 7 (Suppl-1), 206.
62. F. Tatsuzawa, K. Kato, Y. Nagamatsu, H. Shono, K. Toki, and N. Saito, Hort. Res. (Japan), 2013, 12, 23. CrossRef
63. F. Tatsuzawa, K. Miyoshi, T. Yukawa, K. Shinoda, K. Toki, N. Saito, A. Shigihara, and T. Honda, Biochem. Syst. Ecol., 2011, 39, 220. CrossRef
64. F. Tatsuzawa, Y. Toya, H. Watanabe, Y. Hirayama, K. Shinoda, R. Hara, H. Seki, and T. Ando, Heterocycles, 2004, 63, 509. CrossRef
65. F. Tatsuzawa, N. Saito, K. Toki, K. Shinoda, A. Shigihara, and T. Honda, Phytochemistry, 2008, 69, 1029. CrossRef
66. K. Toki, N. Saito, A. Shigihara, and T. Honda, Phytochemistry, 2001, 56, 711. CrossRef
67. K. Yoshida, M. Mori, and T. Kondo, Nat. Prod. Rep., 2004, 26, 884. CrossRef
68. S. Asen and H. W. Siegelman, Proc. Am. Soc. Hort. Sci., 1957, 70, 478.
69. K. Takeda, T. Yamashita, A. Takahashi, and C. F. Timberlake, Phytochemistry, 1990, 29, 1089. CrossRef
70. K. Takeda, M. Yanagisawa, T. Kifune, T. Kinoshita, and C. F. Timberlake, Phytochemistry, 1994, 35, 1165.
71. M. Shiono, N. Matsugaki, and K. Takeda, Nature, 2005, 436, 791. CrossRef
72. K. Takeda, A. Osakabe, S. Saito, D. Furuyama, A. Tomita, Y. Kojima, M. Yamada, and M. Sakuta, Phytochemistry, 2005, 66, 1607. CrossRef
73. K. Hayashi, Y. Abe, and S. Mitsui, Proc. Japan Acad., 1958, 34, 373. CrossRef
74. T. Kondo, K. Yoshida, A. Nakagawa, T. Kawai, H. Tamura, and T. Goto, Nature, 1992, 358, 515. CrossRef
75. F. Tatsuzawa, Y. Mikanagi, N. Saito, K. Shinoda, A. Shigihara, and T. Honda, Biochem. Syst. Ecol., 2005, 33, 789. CrossRef
76. S. J. Bloor, Phytochemistry, 1997, 45, 1399. CrossRef
77. K. Yoshida, S. Kitahara, D. Ito, and T. Kondo, Phytochemistry, 2006, 67, 992. CrossRef
78. K. Yoshida, H. Aoki, K. Kaneda, and T. Kondo, ITE Letters on Batteries New Technologies & Medicine, 2002, 3, 35.
79. N. Saito, M. Yokoi, M. Yamaji, and T. Honda, Phytochemistry, 1985, 24, 2125. CrossRef
80. N. Saito, M. Yokoi, M. Ogawa, M. Kamijo, and T. Honda, Phytochemistry, 1988, 27, 1399. CrossRef
81. F. Tatsuzawa, N. Saito, N. Murata, K. Shinoda, A. Shigihara, and T. Honda, Phytochemistry, 2003, 62, 1239. CrossRef
82. I. Skaar, M. Jordheim, R. Byamukana, A. Mbabazi, S. G. Wubshet, B. Kiremire, and O. M. Andersen, J. Agric. Food Chem., 2012, 60, 1510. CrossRef
83. N. Ishikura and K. Minegishi, Bot. Mag. Tokyo, 1978, 91, 181. CrossRef
84. J. B. Harborne, Phytochemistry, 1967, 6, 1415. CrossRef
85. T. Kondo, K. Suzuki, K. Yoshida, K. Oki, M. Ueda, M. Isobe, and T. Goto, Tetrahedron Lett., 1991, 33, 6375. CrossRef
86. N. Ishikura, Kumamoto J. Sci. Biol., 1974, 12, 17.
87. N. Ishikura and M. Shimizu, Kumamoto J. Sci. Biol., 1972, 11, 19.
88. K. Toki, N. Saito, H. Kuwano, T. S. Lu, A. Shigihara, and T. Honda, Phytochemistry, 1994, 36, 609. CrossRef
89. K. Toki, N. Saito, A. Nogami, F. Tatsuzawa, A. Shigihara, and T. Honda, Heterocycles, 2009, 78, 2287. CrossRef
90. N. Terahara, A. Callebaut, R. Ohba, T. Nagata, M. Ohnishi-Kameyama, and M. Suzuki, Phytochemistry, 1996, 42, 199. CrossRef
91. T. Kondo, J. Yamashiki, K. Kawahori, and T. Goto, Tetrahedron Lett., 1989, 30, 6055. CrossRef
92. F. Tatsuzawa, N. Saito, H. Seki, M. Morita, T. Yukawa, K. Shinoda, and T. Honda, Biochem. Syst. Ecol., 2004, 32, 651. CrossRef
93. N. Saito, K. Toki, H. Moriyama, A. Shigihara, and T. Honda, Phytochemistry, 2002, 60, 365. CrossRef
94. F. Tatsuzawa, M. Hosokawa, N. Saito, and T. Honda, South African J. Bot., 2012, 79, 71. CrossRef
95. S. Asen, R. J. Griesbach, K. H. Norries, and B. A. Leonhardt, Phytochemistry, 1986, 25, 2509. CrossRef
96. N. Terahara, H. Suzuki, K. Toki, H. Kuwano, N. Saito, and T. Honda, J. Nat. Prod., 1993, 56, 335. CrossRef
97. S. J. Bloor, Phytochemistry, 1999, 50, 1395. CrossRef
98. N. Ishikura, Plant Cell Physiol., 1978, 19, 887.
99. N. Akavia, D. Strack, and A. Cohen, Z. Naturforsch., 1981, 36C, 378.
100. N. Saito and J. B. Harborne, Phytochemistry, 1992, 31, 3009. CrossRef
101. K. Takeda, J. B. Harborne, and P. G. Watermann, Phytochemistry, 1993, 34, 421. CrossRef
102. K. Toki, N. Terahara, N. Saito, T. Honda, and T. Shioji, Phytochemistry, 1991, 30, 671. CrossRef
103. K. Takeda and K. Hayashi, Proc. Japan Acad., 1965, 41, 449.
104. R. J. Griesbach, S. Asen, and B. A. Leonnarat, Phytochemistry, 1991, 30, 1729. CrossRef
105. K. Toki, N. Saito, M. Kitamura, F. Tatsuzawa, A. Shigihara, and T. Honda, Heterocycles, 2011, 83, 2011. CrossRef
106. N. Saito, S. Mitsui, and K. Hayashi, Proc. Japan Acad., 1960, 36, 340.
107. K. Toki, M. Takeuchi, N. Saito, and T. Honda, Phytochemistry, 1996, 42, 1055. CrossRef
108. K. Toki, N. Saito, K. Iinuma, and T. Honda, Phytochemistry, 1994, 36, 1181. CrossRef
109. K. Toki and N. Katsuyama, J. Japan Hort. Sci., 1995, 64, 853. CrossRef
110. F. Tatsuzawa, N. Saito, Y. Mikanagi, K. Shinoda, K. Toki, A. Shigihara, and T. Honda, Phytochemistry, 2009, 70, 672. CrossRef
111. K. Yoshitama, K. Ishii, and H. Yasuda, J. Fac. Sci. Shinshu Univ., 1980, 15, 19.
112. M. Shibata and N. Ishikura, Jap. J. Bot., 1960, 17, 230.
113. K. Hayashi and Y. Abe, Misc. Rep. Inst. Nat. Resour. Tokyo, 1953, 29, 1.
114. F. Tatsuzawa, N. Saito, M. Yokoi, A. Shigihara, and T. Honda, Phytochemistry, 1996, 41, 635. CrossRef
115. K. Yoshitama and K. Abe, Phytochemistry, 1976, 16, 591. CrossRef
116. N. Saito, K. Toki, K. Uesato, A. Shigihara, and T. Honda, Phytochemistry, 1994, 37, 245. CrossRef
117. F. Tatsuzawa, N. Takahashi, K. Kato, K. Shinoda, N. Saito, and T. Honda, Phytochemistry Lett., 2014, 7, 69. CrossRef
118. F. Tatsuzawa, N. Saito, A. Shigihara, T. Honda, K. Toki, K. Shinoda, T. Yukawa, and K. Miyoshi, J. Japan. Soc. Hort. Sci., 2010, 79, 215. CrossRef
119. F. Tatsuzawa, N. Saito, K. Toki, K. Shinoda, and T. Honda, J. Japan. Soc. Hort. Sci., 2012, 81, 91. CrossRef
120. N. Saito, F. Tatsuzawa, E. Suenaga, K. Toki, K. Shinoda, A. Shigihara, and T. Honda, Phytochemistry, 2008, 69, 3139. CrossRef
121. F. Tatsuzawa, Biochem. Syst. Ecol., 2012, 44, 374. CrossRef
122. J. B. Harborne, Phytochemistry, 1963, 2, 85. CrossRef
123. K. Toki, N. Saito, H. Kuwano, N. Terahara, and T. Honda, Phytochemistry, 1995, 38, 515. CrossRef
124. K. Toki, J. Ichikawa, and M. Kisue, Bull. Facul. Hort. Minamikyushu Univ., 1996, 26, 13.
125. T. Endo, Bot. Mag. Tokyo, 1959, 72, 10. CrossRef
126. S. Ootani, Rep. Inst. Breeding Res. Tokyo Univ. of Agric., 1973, 1.
127. J. B. Harborne, Experientia, 1961, 17, 72. CrossRef
128. F. Tatsuzawa and T. Ando, J. Japan. Hort. Sci., 2005, 74, 482. CrossRef
129. T. Kondo, M. Yoshikane, K. Yoshida, and T. Goto, Tetrahedron Lett., 1989, 30, 6729. CrossRef
130. N. Terahara, T. Shioji, K. Toki, N. Saito, and T. Honda, Phytochemistry, 1984, 28, 1507. CrossRef
131. K. Toki, T. Yamamoto, N. Terahara, N. Saito, T. Honda, H. Inoue, and H. Mizutani, Phytochemistry, 1991, 30, 3828. CrossRef
132. J. B. Harborne, Archs. Biochem. Biophys., 1962, 96, 171. CrossRef
133. N. Terahara and M. Yamaguchi, Phytochemistry, 1986, 25, 2906. CrossRef
134. K. Toki, N. Saito, Y. Irie, F. Tatsuzawa, A. Shigihara, and T. Honda, Phytochemistry, 2008, 69, 1215. CrossRef
135. N. Saito, K. Toki, T. Honda, and F. Tatsuzawa, Heterocycles, 2012, 85, 1427. CrossRef
136. M. Tanaka, T. Fujimori, I. Uchida, S. Yamaguchi, and K. Takeda, Phytochemistry, 2001, 56, 373. CrossRef
137. T. Goto, T. Kondo, H. Tamura, H. Imagawa, A. Iino, and K. Takeda, Tetrahedron Lett., 1980, 23, 3695. CrossRef
138. K. Toki, N. Saito, and T. Honda, Phytochemistry, 1998, 48, 729. CrossRef
139. K. Hosokawa, Y. Fukunaga, E. Fukushi, and J. Kawabata, Phytochemistry, 1995, 38, 1293. CrossRef
140. N. Saito, F. Tatsuzawa, K. Miyoshi, A. Shigihara, and T. Honda, Tetrahedron Lett., 2003, 44, 6821. CrossRef
141. F. Tatsuzawa, N. Saito, and M. Yokoi, Lindleyana, 1996, 11, 214.
142. T. Fossen, R. Slimestad, D. O. Øvstendal, and Ø. M. Andersen, Phytochemistry, 2000, 54, 317. CrossRef
143. F. Tatsuzawa, N. Saito, N. Murata, K. Shinoda, A. Shigihara, and T. Honda, Heterocycles, 2002, 57, 1787. CrossRef
144. F. Tatsuzawa, N. Murata, K. Shinoda, N. Saito, A. Shigihara, and T. Honda, Heterocycles, 2001, 55, 1195. CrossRef