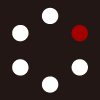
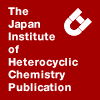
HETEROCYCLES
An International Journal for Reviews and Communications in Heterocyclic ChemistryWeb Edition ISSN: 1881-0942
Published online by The Japan Institute of Heterocyclic Chemistry
e-Journal
Full Text HTML
Received, 28th July, 2014, Accepted, 14th August, 2014, Published online, 20th August, 2014.
DOI: 10.3987/COM-14-S(K)93
■ Total Synthesis of Maremycins A and D1 Using Chiral and Cyclic Nitrone with (E)-3-Ethylidene-1-methylindolin-2-one
Tohru Ueda, Mitsuhide Inada, Nobuyoshi Morita, and Osamu Tamura*
Laboratory of Organic Chemistry, Pharmaceutical Sciences, Showa Pharmaceutical University, 3-3165, Higashi-tamagawagakuen, Machida, Tokyo 194-8543, Japan
Abstract
Total syntheses of maremycin A (4) and maremycin D1 (8) were described, featuring 1,3-dipolar cycloaddition of a chiral cyclic nitrone 15 with (E)-3-ethylidene-1-methylindolin-2-one (13). The cycloaddition was reversible, especially at high temperature in the presence of a Lewis acid or in a solvent possessing a high acceptor number. One of the cycloadducts was efficiently led to maremycin A (4) and maremycin D1 (8). High optical purity of 4 was confirmed by chiral HPLC comparison with ent-4 prepared from ent-15 and 13.INTRODUCTION
β-Methyltryptophan (1) and related structures are important components of many natural products, in which both stereochemistries of the methyl group can be found. For example, indolmycin (2) and FR900452 (3) possess opposite stereochemistry (Figure 1).1
Maremycins A (4) and B (5) are diketopiperazine alkaloids that also include β-methyltryptophan featuring 3-hydroxyindolin-2-one2 structure, and their stereochemistries were tentatively proposed to be as depicted in Figure 2 on the basis of molecular mechanics calculations and spectroscopic data.3 An inseparable 1:1 mixture of maremycins C1 (6) and C2 (7) was obtained from Streptomyces sp. GT 051237, together with an inseparable 3:1 mixture of maremycins D1 (8) and D2 (9).4 The stereochemistries of 6 and 7 were deduced by comparison of the NMR spectroscopic data with those of 5, while the stereostructures of 8 and 9 were assigned by comparison of the spectra with those of 4 and 5. Therefore, confirmation of the stereostructures of maremycins A (4) and B (5) is important to establish the stereochemistries of maremycin family members. Several years ago, we accomplished the first synthesis of maremycins A (4) and D1 (8) and confirmed their stereostructures to be as depicted in Figure 2.5 Since then, several total syntheses of maremycins have been reported.6 We now present a full account of our syntheses of 4 and 8, together with mechanistic considerations, a synthesis of the enantiomer of 4, and determination of the optical purity of the compounds.
RESULTS AND DISCUSSION
The retrosynthetic analysis of maremycins A (4) and D1 (8) is shown in Scheme 1. The exomethylene of 8 can be obtained by elimination of methylsulfide of 4 via its sulfoxide. The diketopiperazine of 4 is constructed from two amino acids, β-methyl amino acid 10 and S-methyl-L-cysteine (11). Connection of oxygen and the amino group in amino acid 10 would afford spiro-isoxazolidine 12, which can be obtained by cycloaddition of 3-ethylidene-indolinone 13 with (E)-C-alkoxycarbonyl nitrone 14 via transition state A.7-9 As an equivalent of 14, we chose chiral cyclic nitrone 15.10-12
The synthesis began with preparation of indolinone 13 according to a known method (Scheme 2).13 Aldol reaction of N-methylisatin (16) with propiophenone (17) in the presence of potassium hydroxide gave a 1:1 diastereomeric mixture of aldol adducts 18. Without separation, the aldol adducts 18 were treated with sulfuric acid to afford (E)-3-ethylidene-1-methylindolin-2-one (13).
The crucial cycloaddition of ethylidene-indolinone 13 with nitrone 15 was next examined. Heating a solution of indolinone 13 (1 equiv) and 15 nitrone (1 equiv) in toluene at 60 °C for two days resulted in cycloaddition reaction to afford a 22:78 mixture of 19 and 20 in 94% yield (Scheme 3).
The structure of 19 was established by X-ray crystallography after recrystallization from hexane-AcOEt, whereas that of 20 was deduced on the basis of detailed NMR studies, including NOE experiments (Figure 3). The crystallography of 19 revealed that the minor product 19 was the desired cycloadduct possessing the required three contiguous stereogenic centers for preparation of non-proteinogenic amino acid 10, and indicated that 19 was formed via transition state B. The NMR studies of 20 showed that the major compound was not the stereoisomer of 19, surprisingly, but a regio isomer of 19 generated via transition state C. It should be noted that both cycloadducts were formed by endo-addition from the less hindered face of nitrone 15.
Unfortunately, the major product was the undesired regio isomer 20. In order to improved the yield of 19, we next examined the use of a Lewis acid such as MgBr2•OEt2,14 ZnBr2,15 Zn(OTf)2,16 Cu(OTf)2,17 or Ti(OiPr)2Cl2.18 However, undesired isomer 20 was always obtained predominantly (19:20 = 33:67 – 4:96), although the total yield of the cycloadducts 19 and 20 was very high (94-100%). For example, in the case of Ti(OiPr)2Cl2, a 4:96 mixture of 19 and 20 was obtained with 100% conversion.
To shorten the reaction time, neat conditions at various reaction temperatures were investigated (Table 1, entries 1-3). Unexpectedly, it was found that higher reaction temperature gave a higher ratio of cycloadduct 20. Thus, reaction of nitrone 15 with 13 at -25 °C afforded a 47:53 mixture of 19 and 20 (entry 1), whereas reaction at 60 °C gave a 22:78 mixture (entry 3). Since a kinetically controlled reaction should exhibit a higher ratio at lower temperature, these results imply that the present cycloaddition at high temperature might involve a thermodynamically controlled equilibrium between 19 and 20 via cycloreversion19 of the cycloadducts 19 and 20 to the starting 13 and 15. The effect of solvent was next examined (entries 4-9). When polar solvents such as methanol and acetonitrile were employed, cycloadduct 20 was predominant (entries 4 and 5). Use of tetrahydrofuran resulted in equal formation of 19 and 20 (entry 6). Since the reactions in entries 4-6 did not go to completion, 3 equiv. of 13 was used for the reaction at 50 °C (entry 7). Interestingly, cycloaddition of 13 and 15 smoothly proceeded in hexane, giving rise to a 1:1 mixture of cycloadducts 19 and 20 (entry 8). In hexane, elevated temperature did not change the ratio of the cycloadducts (entry 9). Since the synthesis of maremycins would require large-scale reaction, neat conditions (entry 2) appeared to be convenient in terms of the distribution of products as well as the solubility of the starting materials in hexane. In fact, reaction of 13 and 15 on a 30 mmol scale under neat conditions furnished a 48:52 mixture of 19 and 20.
Next, the possibility of cycloreversion of cycloadducts 19 and 20 to the starting dipolarophile 13 and nitrone 15 was investigated. We previously found that nitrone 15 reacted with 10 equiv of methyl methacrylate (21) to exclusively afford cycloadduct 22 in quantitative yield (Scheme 4).11 Cycloadduct 19, on heating with methacrylate 21 in toluene at 100 °C for 1 h, released 3-ethylidene-indolin-2-one 13 and afforded cycloadduct 22 of methacrylate 21 in quantitative yield. Similar treatment of cycloadduct 20 with 21 provided 3-ethylidene-indolin-2-one 13 (45%) and cycloadduct 22 (45%), along with recovery of 20 (55%).
Formation of 22 from 19 or 20 would involve regeneration of nitrone 15 and indolinone 13. Thus, heating 19 or 20 would cause cycloreversion to indolinone 13 and nitrone 15, which, in turn, could undergo re-cycloaddition with methacrylate 21 to provide 22 (Scheme 5). The results shown in Scheme 4 also suggest that cycloadduct 20 may be thermodynamically more stable than cycloadduct 19 because cycloadduct 20 exhibited lower cycloreversion reactivity on heating than did 19. In fact, 6-31G** calculation indicated that 20 is more stable by 1.47 kcal/mol than 19. Thus, in the reaction of nitrone 15 and indolinone 13 at low temperature, the reaction rate for the formation of cycloadduct 19 would be as fast as that for 20 (Table 1, entry 1). However, cycloadducts 19 and 20 may exist in equilibrium via the starting nitrone 15 and indolinone 13 at high temperature, and hence formation of more stable 20 is dominant (Table 1, entry 3). We planned to convert regioisomer 20 to the desired cycloadduct 19 via the equilibrium. When a mixture of regioisomer 20 and ethylidene-indolinone 13 was heated in toluene, cycloreversion-re-cycloaddition occurred to give the desired cycloaddition product 19 in 10% yield, accompanied with recovery of regioisomer 20 in 87% yield and ethylidene-indolinone 13 (90%). Thus, the yield of the desired cycloadduct 19 can be improved by recycling this reaction.
Table 1 shows dielectronic constants (ε) and acceptor numbers (AN)20 of solvents used for the cycloaddition. Of the two parameters, AN values seemed to better match the distribution of the two isomers 19 and 20. Thus, reaction in methanol, having a high AN, afforded a high ratio of cycloadduct 20, whereas reaction in hexane, with a low AN (0), gave a 1:1 ratio of cycloadducts 19 and 20. In a solvent of high AN, donating groups of the starting materials 13 and 15 would interact with the solvent to stabilize the left side of the equilibrium, favoring cycloreversion to afford the more stable cycloadduct 20 as the major product through the equilibrium (Scheme 6). Use of a Lewis acid would also stabilize the left side of the equilibrium to yield 20 predominantly.
The next task was hydrogenolysis of cycloadduct 19 with retention of the hydroxyl group at the benzylic position (Scheme 7). Stirring a mixture of 19 and 20%Pd(OH)2 in acetic acid under an atmosphere of hydrogen at room temperature to give amino acid 23 with loss of the benzylic hydroxy group. After experimentation, we finally found that hydrogenolysis in methanol at 50 °C furnished the desired amino acid 10.
To construct the diketopiperazine framework of maremycin A, two routes could be considered (Scheme 8): one is the route via amino acid 24 (route 1) and the other is that via 25 (route 2).
We first examined route 1. Treatment of N-protected amino acid 26 derived from 10 with HATU and S-methylcysteine methyl ester (27)21 in the presence of DIPEA unfortunately resulted in cyclization to gave lactone 28 in place of the desired dipeptide 24 (PG = Boc, R = Me).
Next, route 2 was investigated (Scheme 10). Amino acid was exposed to trimethylsilyldiazomethane to yield ester 29 along with a small amount of lactone 30. Without separation, 29 and 30 were condensed with S-methylcysteine derivative 3122 to afford a mixture 32 and 33. Synthesis of maremycin A (4) was accomplished in 40% yield from amino acid 10 by heating of the mixture of 32 and 33 with silica gel in refluxing xylene via removal of the Boc group, followed by formation of the diketopiperazine ring.
Fortunately, transformation from maremycin A (4) to maremycin D1 (8) could be conducted without difficulty (Scheme 11). The sulfide group of 4 was oxidized with sodium periodate to give sulfoxide 34 as a mixture of diastereomers, which, on heating in toluene with calcium carbonate, underwent syn-elimination to afford maremycin D1 (8).
The final task was confirmation of the optical purity of the synthesized maremycin. For this purpose, we synthesized ent-maremycin A (ent-4) from nitrone ent-15, indolinone 13, and S-methyl-D-cysteine according to the method used for the synthesis of natural 4 (Scheme 12). With both enantiomers of maremycin A in hand, chiral HPLC analysis was conducted. The analyses of racemates prepared from both enantiomers, compound 4, and ent-4 clearly showed that both enantiomers had an optical purity of >99 %ee.
In conclusion, we have accomplished the first synthesis of maremycins A (4) and D1 (8), featuring cycloaddition of cyclic nitrone 15 with (E)-3-ethylidene-1-methylindolin-2-one (13). This also conclusively determined the stereochemistries of the natural products 4 and 8. In addition, 8 was fully characterized for the first time. Mechanistically, it was demonstrated that cycloreversion of the cycloadducts 19 and 20 influenced the distribution of 19 and 20, as did the acceptor number of the solvent used for the cycloaddition. This short-step synthesis clearly shows the usefulness of nitrone cycloaddition of 15 in the synthesis of natural products containing unusual amino acids.
EXPERIMENTAL
General: Melting points are uncorrected. Infrared (IR) spectra were recorded with a Shimadzu FTIR-8200A spectrometer, and 1H NMR and 13C NMR spectra were recorded with JEOL JNM-AL300 spectrometer, with tetramethylsilane as an internal standard (CDCl3 solution). Chemical shifts are recorded in ppm, and coupling constants (J) in Hz. Mass spectra were recorded on JEOL JMS-D300 and HX110 spectrometers. Elemental analyses were performed on an Amco Flash EA 1112 instrument. Merck silica gel 60 (1.09385) and Merck silica gel 60 F254 were used for column chromatography and thin layer chromatography (TLC), respectively.
3-Hydroxy-1-methyl-3-(1-methyl-2-oxo-2-phenylethyl)-1,3-dihydro-2H-indol-2-one (18)
Potassium hydroxide (372 mg, 6.6 mmol) was dissolved in H2O-EtOH (2:3, 200 mL). N-Methylisatin (16) (9.88 g, 59.5 mmol) and propiophenone (17) (12.0 mL, 90.2 mmol) were added to the KOH solution (140 mL, 4.6 mmol) prepared above. The mixture was stirred at room temperature for 6 h, and then the mixture was concentrated under reduced pressure. The residue was chromatographed on silica gel with CH2Cl2-AcOEt (5:1) to give 18 (14.5 g, 81%) as a ca 3:1 mixture of diastereomers. This material was used for the next step without further purification.
Major diastereomer: 1H NMR (300 MHz, CDCl3) δ 1.26 (3H, d, J = 7.1 Hz), 3.20 (3H, s), 4.02 (1H, q, J = 7.1 Hz), 4.34 (1H, s), 6.84 (1H, br d, J = 7.9 Hz), 7.09 (1H, td, J = 7.7, 0.9 Hz), 7.34 (1H, td, J = 7.7, 1.3 Hz), 7.42-7.51 (2H, m), 7.54-7.62 (2H, m), 7.93-7.99 (2H, m).
Minor diastereomer: 1H NMR (300 MHz, CDCl3) δ 1.60 (3H, d, J = 6.8 Hz), 3.13 (3H, s), 3.93 (1H, q, J = 7.2 Hz), 4.15 (1H, s), 6.82 (1H, dd, J = 8.2, 0.5 Hz), 6.98 (1H, td, J = 7.5, 0.9 Hz), 7.26-7.34 (2H, m), 7.38-7.46 (2H, m), 7.55 (1H, tt, J = 7.3 1.5 Hz), 7.77-7.83 (2H, m).
(3E)-3-Ethylidene-1-methyl-1,3-dihydro-2H-indol-2-one (13)
Compound 18 (14.5 g, 49.2 mmol) was added to concentrated H2SO4 (400 mL) at 0 °C, and then the mixture was stirred at room temperature for 10 min. The mixture was poured into ice water, and the whole was extracted with CHCl3. The combined organic phases were washed with a saturated aqueous solution of NaHCO3 and brine, and dried over MgSO4. The solvent was removed by rotary evaporation and the crude product was purified by column chromatography on silica gel with hexane-AcOEt (3:1) to give 13 (7.97 g, 94%) as a yellow solid. 1H NMR (300 MHz, CDCl3) δ 2.28 (3H, d, J = 7.6 Hz), 3.24 (3H, s), 6.83 (1H, br d, J = 7.8 Hz), 7.05 (1H, td, J = 7.6, 1.0 Hz), 7.13 (1H, q, J = 7.6 Hz), 7.28 (1H, td, J = 7.8, 1.0 Hz), 7.58 (1H, br d, J = 7.6 Hz). Spectral data were identical with the literature values.13
(3S,3'R,3a'S,7'S)-1,3'-Dimethyl-7'-phenyl-6',7'-dihydro-3'H-spiro[indole-3,2'-isoxazolo[3,2-c][1,4]-oxazine]-2,4'(1H,3a'H)-dione (19),
(2'R,3S,3a'S,7'S)-1,2'-dimethyl-7'-phenyl-6',7'-dihydrospiro[indole-3,3'-isoxazolo[3,2-c][1,4]oxazine]-2,4'(1H,3a'H)-dione (20) and their enantiomers ent-19 and ent-20
(a) A solution of nitrone 15 (99.5 mg, 0.521 mmol) and 13 (90.0 mg, 0.520 mmol) in toluene (5 mL) was heated at 60 °C for 49 h and then concentrated under reduced pressure to give a 22:78 mixture (181 mg, 94%). Chromatography on silica gel (CH2Cl2-MeCN, 20 : 1) gave 19 and 20.
19: mp 142.5-143.5 °C (hexane-AcOEt); [α]D27 +125.2 (c 1.00, CHCl3); IR (KBr) 3444, 1746, 1736, 1614, 1497, 1472, 1458, 1375, 1352, 1298, 1227, 1043, 768, 760, 696 cm-1; 1H NMR (300 MHz, CDCl3) δ 1.07 (3H, d, J = 7.0 Hz), 3.13 (3H, s), 3.44 (1H, dq, J = 11.7, 7.0 Hz), 3.89-4.26 (3H, m), 4.97 (1H, dd, J = 9.7, 5.1 Hz), 6.76 (1H, d, J = 7.9 Hz), 7.06 (1H, td J = 7.6, 0.9 Hz), 7.24-7.39 (5H, m), 7.55 (2H, d, J = 6.8 Hz), 1H NMR (300 MHz, C6D6) δ 0.91 (3H, d, J = 6.9 Hz), 2.26 (3H, s), 3.38 (1H, dq, J = 11.6, 6.8 Hz), 3.75 (1H, dd, J = 11.6, 4.1 Hz), 3.83 (1H, dd, J = 11.5, 10.8 Hz), 4.14 (1H, d, J = 11.9 Hz), 4.97 (1H, dd, J = 10.8, 4.0 Hz), 6.00 (1H, d, J = 7.7 Hz), 6.72 (1H, td, J = 7.5, 1.0 Hz), 6.85 (1H, d, J = 7.2 Hz), 6.90 (1H, dd, J = 7.7, 1.4 Hz), 7.03 (1H, dt, J = 6.1, 1.4 Hz) 7.58-7.64 (2H, m); 13C NMR (75 MHz, CDCl3) δ 13.3, 26.7, 49.0, 62.5, 70.2, 70.6, 87.7, 108.7, 123.0, 125.5, 125.8, 128.3, 128.5, 128.7, 130.4, 135.3, 143.8, 167.6, 175.8; HRMS m/z calcd for C21H20N2O4, 364.1423; found, 364.1415. Anal. Calcd for C21H20N2O4: C, 69.22; H, 5.53; N, 7.69. Found: C, 69.49; H, 5.50; N, 7.58.
20: mp 136.0-140.0 °C (hexane-AcOEt); [α]D24 +160.2 (c 1.00, CHCl3); IR (KBr) 3442, 1751, 1713, 1612, 1495, 1472, 1458, 1375, 1231, 1094, 756 cm-1; 1H NMR (300 MHz, CDCl3) δ 0.92 (3H, d, J = 6.2 Hz), 3.25 (3H, s), 4.27 (1H, dd, J = 11.4, 10.6 Hz), 4.43 (1H, dd, J = 11.5, 3.4 Hz), 4.71 (1H, q, J = 6.2 Hz), 4.82 (1H, s), 5.19 (1H, dd, J = 10.5, 3.3 Hz), 6.91 (1H, dd, J = 8.3, 1.0 Hz), 7.15 (1H, td, J = 7.6, 1.0 Hz), 7.32-7.47 (5H, m), 7.51-7.59 (2H, m), 13C NMR (75 MHz, CDCl3) δ 14.3, 26.7, 58.7, 67.5, 71.1, 74.7, 79.9, 109.0, 123.0, 124.4, 127.9, 128.2, 128.7, 128.9, 129.2, 134.9, 143.6, 167.1, 176.0; HRMS m/z calcd for C21H20N2O4, 364.1423; found, 364.1423.
(b) Nitrone 15 (6.07 g, 31.8 mmol) and 13 (5.50 g, 31.8 mmol) were dissolved in CH2Cl2 (10 mL). The mixture was concentrated under reduced pressure below 10 °C, and the residue was kept room temperature for 33 h. The 1H NMR spectrum of the mixture showed a 48:52 mixture of 19 and 20. Flash chromatography (CH2Cl2-MeCN, 20:1) afforded pure 19 and 20, whose 1H NMR spectra were identical with those obtained in (a).
(c) A solution of nitrone 15 (3.0 mg, 0.016 mmol) and 13 (8.2 mg, 0.047 mmol) in hexane (0.5 mL) was heated at 50 °C for 2.5 h, then concentrated. The 1H NMR spectrum of the residue showed completion of the reaction and formation of a 1:1 mixture of 19 and 20.
(d) According to procedure (b), reaction of ent-15 (507 mg, 2.66 mmol) with 13 (503 mg, 2.90 mg) afforded a 47:53 mixture of ent-19 and ent-20 (901 mg, 93%). Pure samples were obtained by chromatography.
ent-19: mp 141.5-143.0 °C (hexane-AcOEt); [α]D27 = -127.7 (c 1.0, CHCl3).
ent-20: mp 135.0-139.0 °C (hexane-AcOEt); [α]D27 = -156.5 (c 1.0, CHCl3).
Transformation of 20 to 19
A solution of 20 (25.0 mg, 0.0686 mmol) and 13 (35.7 mg, 0.206 mmol) in toluene (1.0 mL) was heated at 100 °C for 1 h. After concentration, the mixture was chromatographed to give 19 (2.6 mg, 10%) along with recovery of 20 (21.8 mg, 87%) and 13 (32.2 mg, 90%).
(2S,3R)-2-Amino-3-[(3S)-3-hydroxy-1-methyl-2-oxo-2,3-dihydro-1H-indol-3-yl]butanoic acid (10) and its enantiomer ent-10
(a) A mixture of 19 (1.00 g, 2.74 mmol) and 20% Pd(OH)2/C (50% wet, 1.00 g) in MeOH (200 mL) was stirred at 50 °C under an atmosphere of hydrogen for 1 h. The mixture was filtered and the filtrate was concentrated under reduced pressure to give a residue, which was triturated with CH2Cl2 to afford 10 (580 mg, 80%) as a colorless powder. mp 159.5-160.0 °C; [α]D28 -32.8 (c 0.20, MeOH); IR (KBr) 3446, 3128, 3059, 2975, 2935, 2890, 2361, 1709, 1614, 1500, 1495, 1420, 1377, 1354, 1323, 1261, 1219, 1124, 1097, 1086, 758, 692, 540 cm-1; 1H NMR (300 MHz, CD3OD) δ 0.91 (3H, d, J = 7.1 Hz), 2.25 (1H, quin, J = 7.0 Hz), 3.20 (3H, s), 4.41 (1H, d, J = 6.0 Hz), 7.03 (1H, d, J = 7.7 Hz), 7.15 (1H, td J = 7.5, 0.7 Hz), 7.40 (1H, td, J = 7.7, 1.1 Hz), 7.51 (1H, d, J = 7.5 Hz), 13C NMR (75 MHz, CD3OD) δ 11.2, 26.5, 41.4, 56.5, 79.7, 110.1, 124.1, 126.3, 130.6, 131.1, 144.4, 173.1, 179.9, HRMS m/z calcd for C13H16N2O4, 264.1110, found, 264.1093.
(b) According to procedure (a), hydrogenolysis of ent-19 (300 mg, 0.824 mmol) with 20% Pd(OH)2/C (50% wet, 301 mg) in MeOH (100 mL) afforded ent-10 (184 mg, 84%). mp 160.0-160.5 °C; [α]D23 -33.0 (c 0.20, MeOH).
Methyl (2S,3R)-2-Amino-3-[(3S)-3-hydroxy-1-methyl-2-oxo-2,3-dihydro-1H-indol-3-yl]butanoate (29) and lactone 30, their enantiomers ent-29 and ent-30
(a) To a stirred solution of 10 (400 mg, 1.52 mmol) in benzene-MeOH (10:1, 15 mL) was added a 2.0 M solution of TMSCHN2 in hexane (7.5 mL, 15 mmol) at 0 °C, and the mixture was stirred at the same temperature for 2 h. The mixture was concentrated under reduced pressure, and then the residue was diluted with CH2Cl2, washed successively with water and brine, dried (Na2SO4), and concentrated to give 29 (420 mg, quant) that contained a small amount of 30.
29: 1H NMR (300 MHz, CDCl3) δ 0.51 (3H, d, J = 7.0 Hz), 2.52 (1H, dq, J = 10.3, 6.6 Hz), 3.18 (3H, s), 3.72 (3H, s), 3.89 (1H, d, J = 10.3 Hz), 6.83 (1H, d, J = 7.7 Hz), 7.10 (1H, t, J = 7.3 Hz), 7.2-7.4 (2H, m).
30: 1H NMR (300 MHz, CDCl3) δ 1.13 (3H, d, J = 7.3 Hz), 2.87 (1H, quin, J = 7.7 Hz), 3.20 (3H, s), 4.87 (1H, d, J = 7.7 Hz), 6.87 (1H, dd, J = 8.3, 0.9 Hz), 7.11 (1H, td, J = 7.6, 0.7 Hz), 7.37-7.45 (2H, m).
This material was used for the next step without further purification.
(b) According to a procedure similar to that described in (a), ent-29 (166 mg, 98%) containing a small amount of ent-30 was obtained from ent-10 (162 mg, 0.613 mg).
This material was used for the next step without further purification.
(2S,3R)-2-{[N-(tert-Butoxycarbonyl)-S-methyl-L-cysteinyl]amino}-3-[(3S)-3-hydroxy-1-methyl-2-oxo-2,3-dihydro-1H-indol-3-yl] butanoate 32 and lactone 33, their enantiomers ent-32 and ent-33
(a) 2-(1H-7-Azabenzotriazol-1-yl)-1,1,3,3-tetramethyluronium hexafluorophosphate methanaminium (HATU, 651 mg, 1.66 mmol) was added to a stirred solution of 29 prepared above (418 mg, 1.50 mmol), 31 (394 mg, 1.65 mmol), and diisopropylethylamine (0.30 mL, 1.74 mmol) in DMF (15 mL) at 0 °C, and the mixture was stirred at room temperature for 10 min. After concentration, the residue was diluted with CH2Cl2, washed with 10% aqueous HCl, a saturated aqueous solution of NaHCO3 and brine, dried (Na2SO4), and concentrated to give 32 (691 mg, 92%) containing a small amount of 33 as a syrup.
32: 1H NMR (300 MHz, CDCl3) δ 0.87 (3H, d, J = 7.0 Hz), 1.43 (9H, s), 2.17 (3H, s), 2.70 (1H, quin, J = 7.2 Hz), 3.19 (3H, s), 3.69 (3H, s), 3.99 (1H, br s), 4.25 (1H, q, J = 7.2 Hz), 4.67 (1H, br s), 5.39 (1H, d, J = 7.3 Hz), 6.84 (1H, d, J = 7.9 Hz), 7.10 (1H, td, J = 7.2 Hz), 7.35 (1H, td, J = 7.8, 1.2 Hz), 7.47 (1H, br d, J = 7.5 Hz).
33: 1H NMR (300 MHz, CDCl3) δ 1.03 (3H, d, J = 7.3 Hz), 1.46 (9H, s), 2.18 (3H, s), 2.87 (1H, dd, J = 13.8, 6.7 Hz), 2.97 (1H, dd, J = 13.9, 6.2 Hz), 3.20 (3H, s), 3.14-3.27 (1H, m), 4.31 (1H, q, J = 6.7 Hz), 5.33 (1H, br s), 5.77 (1H, dd, J = 7.8, 5.6 Hz), 6.88 (1H, d, J = 7.7 Hz), 6.99 (1H, br d, J = 5.0 Hz), 7.31 (1H, dq, J = 7.5, 0.6 Hz), 7.42 (1H, td, J = 7.8, 1.3 Hz).
This material was used for the next step without further purification.
(b) According to a procedure similar to that described in (a), ent-32 (262 mg, quant.) containing a small amount of ent-33 was obtained from ent-29 (157 mg, 0.566 mg) and ent-31 (124 mg, 0.528 mmol).
This material was used for the next step without further purification.
(3S,6R)-3-{(1R)-1-[(3S)-3-Hydroxy-1-methyl-2-oxo-2,3-dihydro-1H-indol-3-yl]ethyl}-6-[(methylsulfanyl)methyl]piperazine-2,5-dione, maremycin A (4) and its enantiomer ent-4
(a) A mixture of 32 prepared above (688 mg, 1.39 mmol) and silica gel (3.45 g) in xylene (20 mL) was heated at reflux for 30 min. The solvent was evaporated, and the residue was subjected to column chromatography on silica gel (CHCl3 then CHCl3-MeOH, 40:1) to give maremycin A (4) (220 mg, 40% from 10) as a colorless solid. mp 229.0-229.5 °C (lit.3 229 °C); [α]D25 -110.2 (c 0.15, MeOH), [lit.3 [α]D25 -120.95 (c 0.21, MeOH); IR (KBr) 3433, 2920, 1707, 1670, 1614, 1472, 1458, 1375, 1261, 1213, 1126, 1097, 976, 758, 692, 540 cm-1; 1H NMR (300 MHz, DMSO-d6) δ 1.11 (3H, d, J = 7.3 Hz), 2.04 (1H, dd, J = 7.3, 2.2 Hz), 2.08 (3H, s), 2.79 (1H, dd, J = 13.9, 4.0 Hz), 2.97 (1H, dd, J = 13.9, 3.7 Hz), 3.09 (3H, s), 4.27 (1H, m), 4.88 (1H, br s), 7.00 (1H, d, J = 7.5Hz), 7.04 (1H, ddd, J = 7.5, 7.5, 1.0 Hz), 7.29-7.40 (2H, m), 7.59 (1H, br s), 7.92 (1H, br s), 8.64 (1H, br s), 13C NMR (75 MHz, DMSO-d6) δ 8.3, 16.4, 26.0, 36.4, 43.1, 53.6, 54.3, 76.4, 108.6, 121.9, 125.1, 129.2, 130.7, 143.1, 165.7, 168.1, 187.1, HRMS m/z calcd for C17H21N3O4S, 363.1253, found, 363.1258. These data were identical with reported values.3
(b) According to a procedure similar to that described in (a), ent-4 (70 mg, 31% from ent-10) was obtained from ent-32 (252 mg, 0.566 mg) and silica gel (1.26 g).
ent-4: mp 225.0-226.0 °C; [α]D22 +99.4 (c 0.10, MeOH).
(c) HPLC analysis. Analytical conditions were as follows: column, DAICEL Chiralcel OJ-H; mobile phase, hexane-EtOH (1:5); flow rate, 0.25 mL/min; detector, UV (254 nm). Retention times for racemic 4: 18.2 min (50%, 4), 21.7 min (50%, ent-4). Retention time for 4: 18.4 min (100%). Retention time for ent-4: 21.7 min (100%).
(3S,6R)-3-{(1R)-1-[(3S)-3-Hydroxy-1-methyl-2-oxo-2,3-dihydro-1H-indol-3-yl]ethyl}-6-[(methylsulfinyl)methyl]piperazine-2,5-dione (34)
To a solution of maremycin A (4, 46.0 mg, 0.126 mmol) in MeOH (20 mL) was added a solution of NaIO4 (41.3 mg, 0.193 mmol) in water (4.0 mL) at room temperature, and the mixture was stirred at the same temperature for 17 h. To the mixture was added an additional solution of NaIO4 (20.3 mg, 0.093 mmol) in water (2.0 mL), and stirring was continued for 20 h. After concentration under reduced pressure, the crude material was chromatographed on silica gel (CH2Cl2-MeOH, 10:1) to give 34 (43.2 mg, 90%) as a 1:1 mixture of diastereomers.
1H NMR (300 MHz, DMSO-d6) δ 0.95 (3H, d, J = 7.0 Hz), 1.00 (3H, d, J = 7.1 Hz), 2.17-2.32 (2H, m), 2.62 (6H, s), 2.97-3.29 (5H, m), 3.09 (3H, s), 3.10 (3H, s), 4.42-4.50 (2H, m), 4.56 (1H, br s), 4.61 (1H, br s), 7.00 (2H, br d, J = 7.9 Hz), 7.05 (2H, br t, J = 8.0 Hz), 7.11 (1H, s), 7.15 (1H, s), 8.09 (1H, s), 8.20 (1H, s), 8.33 (1H, s), 8.56 (1H, s). This material was used for the next step without further purification.
(3S)-3-{(1R)-1-[(3S)-3-Hydroxy-1-methyl-2-oxo-2,3-dihydro-1H-indol-3-yl]ethyl}-6-methylidenepiperazine-2,5-dione, maremycin D1 (8)
A mixture of 34 (40.8 mg, 0.107 mmol) and CaCO3 (11.9 mg, 0.119 mmol) in toluene (2.0 mL) was stirred at 100 °C for 24 h, and then concentrated under reduced pressure. The crude material was chromatographed on silica gel (CH2Cl2-MeOH, 10:1) to give recovered maremycin A S-oxide (22.3 mg, 55%) and maremycin D1 (5) [10.9 mg, 33.7% (71% based on recovered starting material)], mp 224.0-226.0 °C (decomp.). [α]D22 -40.8 (c 0.1, MeOH); IR (KBr) 3310, 3209, 2920, 1708 cm-1; 1H NMR (300 MHz, DMSO-d6) δ 1.00 (3H, d, J = 7.3 Hz), 2.57 (1H, dq, J = 7.7, 2.2 Hz), 3.04 (3H, s), 4.14 (1H, br s), 4.65 (1H, s), 5.12 (1H, s), 6.24 (1H, br s), 6.91 (1H, d, J = 7.7 Hz), 7.00 (1H, td, J = 7.7, 0.7 Hz), 7.29, (1H, td, J = 7.7, 1.5 Hz), 7.35 (1H, dt, J = 7.4, 0.6 Hz), 8.27 (1H, br s), 10.40 (1H, br s); 13C NMR (75 MHz, DMSO-d6) δ 10.0, 25.8, 44.4, 56.9, 75.9, 98.8, 108.2, 121.7, 124.9, 129.1, 129.7, 134.4, 143.5, 158.0, 165.2, 176.5; HRMS m/z calcd for C16H17N3O4, 315.1219, found 315.1217. The 1H and 13C NMR data were identical reported values.4
ACKNOWLEDGEMENTS
This work was supported in part by “Platform for Drug Discovery, Informatics, and Structural Life Science from the Ministry of Education, Culture, Sports, Science and Technology, Japan,” “the Takeda Science Foundation,” “Research Foundation for Pharmaceutical Sciences, the Houan-sha,” “MEXT-Supported Program for the Strategic Research Foundation at Private Universities (2013-2017),” and “Grants-in-Aid for Scientific Research (C) from JSPS.”
References
1. Y. Zou, Q. Fang, H. Yin, Z. Liang, D. Kong, L. Bai, Z. Deng, and S. Lin, Angew. Chem. Int. Ed., 2013, 52, 12951. CrossRef
2. For a review on 3-substituted-3-hydroxy-2-oxindoles, see: S. Peddibhotla, Curr. Bioact. Compd., 2009, 5, 20. CrossRef
3. W. Balk-Bindseil, E. Helmke, H. Weyland, and H. Laatsch, Liebigs Ann. Chem., 1995, 1291. CrossRef
4. Y. Tang, I. Sattler, R. Thiericke, and S. Grabley, Eur. J. Org. Chem., 2001, 261. CrossRef
5. T. Ueda, M. Inada, I. Okamoto, N. Morita, and O. Tamura, Org. Lett., 2008, 10, 2043. CrossRef
6. G. Bergonzini and P. Melchiorre, Angew. Chem. Int. Ed., 2012, 51, 971; CrossRef Y. Liu, L. Zhang, and Y. Jia, Tetrahedron Lett., 2012, 53, 684; CrossRef J. Dugal-Tessier, E. A. O’Bryan, T. B. H. Schroeder, D. T. Cohen, and K. A. Scheidt, Angew. Chem. Int. Ed., 2012, 51, 4963. CrossRef
7. Unlike azomethine ylides or nitrile oxides, a nitrone rarely undergoes cycloaddition with a 2-oxoindolin-3-ylidene. For known examples, see: R. Raunak, V. Kumar, S. Mukherjee, P. Poonam, A. K. Prasad, C. E. Olsen, S. J. C. Schäffer, S. K. Sharma, A. C. Watterson, W. Erringtone, and V. S. Parmar, Tetrahedron, 2005, 61, 5687; CrossRef S. Malhotra, S. Balwani, A. Dhawan, Raunak, Y. Kumar, B. K. Singh, C. E. Olsen, A. K. Prasad, V. S. Parmar, and B. Ghosh, Med. Chem. Commun., 2012, 3, 1536; Y. Shi, A. Lin, H. Mao, Z. Mao, W. Li, H. Hu, C. Zhu, and Y. Cheng, Chem. Eur. J. 2013, 19, 1914. CrossRef
8. For recent examples of cycloaddition of azomethine ylides with 2-oxoindolin-3-ylidenes, see: L. Shu, Z. Li, C. Gu, and D. Fishlock, Org. Process Res. Dev., 2013, 17, 247; CrossRef A. Dandia, A. K. Jain, A. K. Laxkar, and D. S. Bhati, Tetrahedron, 2013, 69, 2062; CrossRef Y. Arun, G. Bhaskar, C. Balachandran, S. Ignacimuthu, and P. T. Perumal, Bioorg. Med. Chem. Lett., 2013, 23, 1839; CrossRef L. Wang, X.-M. Shi, W.-P. Dong, L.-P. Zhu, and R. Wang, Chem. Commun., 2013, 49, 3458; CrossRef W. Sun, G. Zhu, C. Wu, G. Li, L. Hong, and R. Wang, Angew. Chem. Int. Ed., 2013, 52, 8633; CrossRef J. Day, M. Uroos, R. A. Castledine, W. Lewis, B. McKeever-Abbas, and J. Dowden, Org. Biomol. Chem., 2013, 11, 6502; CrossRef J.-A. Xiao, H.-G. Zhang, S. Liang, J.-W. Ren, H. Yang, and X.-Q. Chen, J. Org. Chem., 2013, 78, 11577; CrossRef S. Lanka, S. Thennarasu, and P. T. Perumal, Tetrahedron Lett., 2014, 55, 2585. CrossRef
9. For selected examples of cycloaddition of azomethine ylides with 2-oxoindolin-3-ylidenes, see: A. Singh and G. P. Roth, Org. Lett., 2011, 13, 2118; CrossRef A. Singh and G. P. Roth, Tetrahedron Lett., 2012, 53, 4889; CrossRef A. V. Velikorodov, V. A. Ionova, O. V. Degtyarev, and L. T. Sukhenko1, Pharm. Chem. J., 2013, 46, 715; CrossRef C. J. A. Ribeiro, J. D. Amaral, C. M. P. Rodrigues, R. Moreira, and M. M. M. Santos, Bioorg. Med. Chem., 2014, 22, 577. CrossRef
10. O. Tamura, K. Gotanda, R. Terashima, M. Kikuchi, T. Miyawaki, and M. Sakamoto, Chem. Commun., 1996, 1861; O. Tamura, T. Kuroki, Y. Sakai, J. Takizawa, J. Yoshino, Y. Morita, N. Mita, K. Gotanda, and M. Sakamoto, Tetrahedron Lett., 1999, 40, 895; CrossRef O. Tamura, S. Yoshida, H. Sugita, N. Mita, Y. Uyama, N. Morita, M. Ishiguro, T. K Kawasaki, H. Ishibashi, and M. Sakamoto, Synlett, 2000, 1553; O. Tamura, K. Gotanda, J. Yoshino, Y. Morita, R. Terashima, M. Kikuchi, T. Miyawaki, N. Mita, M. Yamashita, H. Ishibashi, and M. Sakamoto, J. Org. Chem., 2000, 65, 8544; CrossRef O. Tamura, T. Shiro, A. Toyao, and H. Ishibashi, Chem. Commun., 2003, 2678. CrossRef
11. O. Tamura, T. Shiro, M. Ogasawara, A. Toyao, and H. Ishibashi, J. Org. Chem., 2005, 70, 4569. CrossRef
12. For reviews, see: O. Tamura, Synth. Org. Chem. Jpn., 2010, 68, 1272; CrossRef O. Tamura, 'Methods and Applications of Cycloaddition Reactions in Organic Synthesis: Geometry-Controlled Cycloaddition of C-Alkoxycarbonyl Nitrones: Synthetic Studies on Nonproteinogenic Amino Acids,' ed. by N. Nishiwaki, John Wiley & Sons, Inc., Hoboken, 2014, pp. 151-174. CrossRef
13. P. López-Alvarado and C. Avendaño, Synthesis, 2002, 104. CrossRef
14. S. Kanemasa, T. Tsuruoka, and E. Wada, Tetrahedron Lett., 1993, 34, 87; CrossRef S. Kanemasa and T. Tsuruoka, Chem. Lett., 1995, 49; CrossRef R. Hanselmann, J. Zhou, P. Ma, and P. N. Confalone, J. Org. Chem., 2003, 68, 8739. CrossRef
15. S. Kanemasa, T. Tsuruoka, and E. Wada, Tetrahedron Lett., 1993, 34, 87; CrossRef T. Saito, T. Yamada, S. Miyazaki, and T. Otani, Tetrahedron Lett., 2004, 45, 9581; CrossRef B. Dugovic, L. Fisera, M. K. Cyranski, C. Hametner, N. Pronayova, and M. Obranec, Helv. Chim. Acta, 2005, 88, 1432. CrossRef
16. P. Merino, T. Tejero, M. Laguna, E. Cerrada, A. Moreno, and J. A. Lopez, Org. Biomol. Chem., 2003, 1, 2336; CrossRef Q. Zhao, F. Han, and D. L. Romero, J. Org. Chem., 2002, 67, 3317. CrossRef
17. K. B. Jensen, R. G. Hazell, and K. A. Jørgensen, J. Org. Chem., 1999, 64, 2353. CrossRef
18. P. Bayon, P. De March, M. Figueredo, and J. Font, Tetrahedron, 1998, 54, 15691. CrossRef
19. For examples of cycloreversion of nitrone-cycloadducts, see: G. M. Williams, S. D. Roughley, J. E. Davies, and A. B. Holmes, J. Am. Chem. Soc., 1999, 121, 4900; CrossRef F. Pisaneschi, F. M. Cordero, and A. Brandi, Synlett, 2003, 1889; J. L. Garcia Ruano, A. Fraile, A. M. Martin Castro, and M. R. Martin, J. Org. Chem., 2005, 70, 8825; CrossRef J. Garcia Ruano, J. F. Soriano, A. Fraile, M. R. Martin, and A. Nunez, J. Sulfur Chem., 2013, 34, 17; CrossRef A. J. Hodges, J. P. Adams, A. D. Bond, A. B. Holmes, N. J. Press, S. D. Roughley, J. H. Ryan, S. Saubern, C. J. Smith, M. D. Turnbullf, and A. F. Newton, Org. Biomol. Chem., 2012, 10, 8963. CrossRef
20. Gutmann et al. defined “Acceptor Number,” a quantitative empirical parameter for the Lewis acidities of solvents, based on the 31P-NMR chemical shift of Et3P=O in the solvent, see: U. Mayer, V. Gutmann, and W. Gerger, Monatsh. Chem., 1975, 106, 1235; CrossRef V. Gutmann, ‘The Donor-Acceptor Approach to Molecular Interactions,’ Plenum Press, New York, 1978. CrossRef
21. C. C. G. Scully and P. J. Rutledge, Tetrahedron, 2010, 66, 5653. CrossRef
22. H. Ikeuchi, M. E. Meyer, Y. Ding, J. Hiratake, and N. G. J. Richards, Bioorg. Med. Chem., 2009, 17, 6641. CrossRef