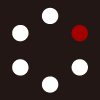
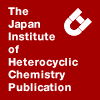
HETEROCYCLES
An International Journal for Reviews and Communications in Heterocyclic ChemistryWeb Edition ISSN: 1881-0942
Published online by The Japan Institute of Heterocyclic Chemistry
e-Journal
Full Text HTML
Received, 30th June, 2014, Accepted, 29th July, 2014, Published online, 7th August, 2014.
DOI: 10.3987/COM-14-S(K)63
■ Enantiodivergent Synthesis of Wieland-Miescher Ketone Analog Mediated by a Chiral Pyridinylmethylamine Derivative
Shota Honda, Kohei Inomata,* and Yasuyuki Endo*
Faculty of Pharmaceutical Sciences, Tohoku Pharmaceutical University, 4-4-1 Komatsushima, Aoba-ku, Sendai 981-8558, Japan
Abstract
A new enantiodivergent route to provide the Wieland-Miescher ketone analog (3b) bearing a 7-membered ring via the intramolecular aldol reaction of the trione (5) mediated by a single chiral pyridinylmethylamine derivative (13e) was established. Although the enantioselectivities of 3b were moderate, the complete inversion of the enantioselectivities was observed based on the amount of additional trifluoroacetic acid (TFA). The basicity of the nitrogen atom on the pyridine ring was very important for this enantiodivergent behavior.INTRODUCTION
Hajos-Parrish (1a), Wieland-Miescher (2a) ketones and their analogs (1b, 2b), which include carbobicyclic enediones, have been highly useful synthons for the total synthesis of a variety of natural products and pharmaceutically important compounds (Figure 1).1-7 These useful enediones have been easily prepared by amino acid-mediated asymmetric intramolecular aldol reactions.8 This asymmetric aldol reaction was first reported by Hajos et al. and has been widely recognized to involve an enamine-based mechanism.2,3 However, there have been few reports on how to prepare 3 bearing a 7-membered ring, in spite of their interesting reaction profiles.1,9 For example, the inversion of enantioselectivities in the asymmetric aldol reactions of the triones 4 and 5, mediated by (S)-phenylalanine [(S)-Phe, 6] in the presence of a Brønsted acid, has been observed to give (S)-2b and (R)-3b, respectively. Equally, different chemoselectivities have also been observed upon the treatment of 2b and 3b with sodium borohydride in EtOH.1a,10 Whereas a hydride stereoselectively attacked at the cyclohexanone carbonyl in 2b from the α-face to afford 7, 3b kinetically and stereoselectively reacted at the conjugated carbonyl to give 8 (Scheme 1). Since many pharmaceutically important natural products containing a 7-membered carbocycle have been isolated,11 the enedione (3) has been significant as a potential chiral synthon to achieve the total synthesis of these products.
We previously reported the preparation of (R)- and (S)-3b via the asymmetric intramolecular aldol reaction of the trione (5), which was easily prepared from 2-methylcycloheptane-1,3-dione,12 mediated by the chiral diamine (9).1c The aldol reaction using a stoichiometric amount of 9 in the presence of 2.0 equivalents of TFA afforded (R)-3b accompanied with 70% ee. However, the inversion of enantioselectivity was observed under the condition of 1.0 equivalent of TFA with a lower ee value. Recently, we established the enantiodivergent preparation of 3b13 using a known chiral triamine (10) which was easily prepared from (S)-Phe (6).14 The enantioselectivities of 3b depended on the amount of additional TFA. The reaction mediated by a stoichiometric amount of 10 in the presence of 1.7 equivalents and 3.5 equivalents of TFA independently afforded (S)- and (R)-3b respectively (Scheme 2). Although the ee values were moderate, we have enantiodivergently achieved the preparation of both enantiomers of 3b using a single chiral mediator (10). Also, we have found that the inversion point of the enantioselectivity existed at around 2.0 equivalents of TFA. We have speculated that this enantiodivergent behavior of 10 was due to a dynamic conformational change in the ethylene diamine moiety. Under less than 2.0 equivalents of TFA, the ethylene diamine moiety coordinated to a proton in a bidentate fashion to form a folding conformation (11). On the other hand, an extended conformation (12) might be preferred under more than 2.0 equivalents of (or an excess amount of) TFA, because of the electrostatic repulsion due to formation of the diammonium structure. Since we are strongly interested in this unique phenomenon concerning 10, we now report the preparation of new chiral mediators (13) installing a pyridine moiety as a basic functionality and their reactivity for the asymmetric aldol reaction of 5 (Scheme 3).
RESULTS AND DISCUSSION
We initially studied the preparation of new chiral amine mediators (13). According to the known procedure,15 the alcohol (15) was obtained from the commercially available (S)-phenylalanine methyl ester (14). The Mitsunobu reaction16 using phthalimide and following deprotection in the presence of hydrazine hydrate afforded the known amine intermediate (17).17 Next, we introduced a variety of pyridinylmethyl groups by the reductive alkylation of 17. Thus, the azeotropic reaction of 17 in the presence of the corresponding commercially available or known aldehyde (18)18,19 to form imines and the following sodium borohydride reduction in MeOH smoothly proceeded to yield 19. Although the direct methylation of 19a using methyl iodide in MeCN afforded 20a in moderate yield, trace amounts of 20b and 20c were obtained under the same reaction conditions. The Eschweiler-Clarke reaction20 succeeded in producing the corresponding methylation products (20b-20e). Finally, the Boc group was removed in the presence of TFA to yield the desired 13 (Scheme 4).
Next, we examined the asymmetric aldol reaction of 5 mediated by 13 in the presence of different amounts of TFA. According to the reaction conditions using 10,13 a stoichiometric amount of 13 was used for the reaction in the presence of 1.7 or 3.5 equivalents of TFA in DMSO at 90 oC. The results are compiled in Table 1. All of the amines (13) mediated the reaction to afford 3b with a moderate enantioselectivity. However, among the tested amines, 13a-13d gave (R)-3b using both amounts of TFA and no enantiodivergent behaviors were observed. In the case of using 13e, (S)- and (R)-3b were obtained using different amounts of TFA. The ee values in each case were almost inverted (Table1, entries 9 and 10).
We next determined the effects of the quantities of TFA on the yield and ee of the aldol reaction of 5 mediated by 13e. All reactions were carried out with 1.0 equivalent of 13e in DMSO at 90 oC in the presence of TFA over the range of 1.0 to 3.5 equivalents (see Table 2 and Figure 2). All of the yields under the tested reaction conditions almost averaged around 80%. However, the absolute configuration of 3b changed from S to R at the point between 1.7 and 2.0 equivalents of TFA. The ee values reached a plateau in the range of 2.5 to 3.5 equivalents of TFA. Based on these results, the inversion point of the enantioselectivity existed at around 1.8 equivalents of TFA.
In the cases of using 13b and 13c, the pyridine unit hardly coordinated to an ammonium proton, like 11, due to a structural restriction (Scheme 5). In spite of their protonation modes (21a ~ d, 22a ~ d), both conformations (21 and 22) seemed to be almost the same at the point of steric hindrance around an ammonium proton which plays a key role in the transition state of the aldol reaction of 5 (vide infra). From this reason, the pyridinylmethyl counterpart in 13b and 13c hardly affected the C-C bond formation process during the aldol reaction to afford (R)-3b using both amounts of TFA.
In the cases of 13a, 13d and 13e bearing a common pyridin-2-yl-methyl substituent tolerating the bidentate coordination to the ammonium proton, a dynamic equilibrium between the coordinated (23) and dissociated (24) conformation might exist in the presence of less than 1.8 equivalents of TFA (Scheme 6). Comparing 23a and 24a (R1 = R2 = H), there were no or only slight steric differences around the ammonium proton. Although the big difference in the steric hindrance due to the direction of the methyl substituent (R1) between 23d and 24d (R1 = Me, R2 = H) existed in the case of 13d, the aldol reaction mediated by 13d afforded (R)-3b accompanied by almost the same ee for both amounts of TFA. This result suggested that the reaction with less than 1.8 equivalents of TFA proceeded through 24 being similar to the diammonium conformer (25) in the presence of an excess amount of TFA.
The increasing basicity of the pyridine nitrogen by installing a 4-dimethylamino substituent [R2 = -N(Me)2] in 13e, 23 was the favored conformer in the equilibrium to invert the enantioselectivity to give (S)-3b. Comparing the well-known pKa values of the conjugated acids of trialkylamine (26), pyridine (27) and 4-dimethylaminopyridine (DMAP, 28), whereas the basicity of the trialkylamine and DMAP are almost the same, pyridine is a much weaker base (Figure 3).21 Since only 13e having DMAP appeared to undergo the same phenomenon as 10, the basicity of the pyridine nitrogen must be a very important factor to exhibit the enatiodivergent fashion in the aldol reaction of 5.
In the aldol reaction of 5, we could not observe the initially formed β-hydroxy ketones under the reaction conditions using 13. Dehydration of the β-hydroxy ketones to afford the corresponding conjugated enone rapidly occured. We previously proposed the possible transition states (TSs) of the aldol reaction of 5 mediated by the diamine (9) via the enamine mechanism.1c According to our proposed model, four TSs concerning the cis/trans and syn/anti (cis, trans: cis- or trans-fused carbocycles, syn, anti: conformation between olefinic and hydrogen bonding sites) were considered (Figure 4). Among the TSs, the trans-anti and cis-anti forming a chair conformation of the 6-membered ring including the hydrogen bonding nitrogen and oxygen atoms are relatively more stable than the trans-syn and cis-syn forming a boat conformation. As already mentioned, under more than 1.8 equivalents of TFA, the hydrogen bond in 23 dissociated and the diammonium ion (25) was formed. This result means that the DMAP moiety in 13e would be oriented away from the C-C bond forming site. In comparison with the two anti-TSs (29 and 31), 31 appears to be not favored due to steric repulsion between the methyl enamine and a 7-membered carbocycle. Consequently, the trans-anti-TS (29) to afford (R)-3b after the dehydration should be relatively more favored than 31 and the other syn-TSs (30 and 32). This behavior was almost the same as the previously reported reaction using 9.1c Since 13a-13d hardly coordinated to form in the bidentate fashion like 23 (vide supra), these mediators afforded (R)-3b via a similar TS like 29 in spite of the different amount of TFA.
For less than 1.8 equivalents of TFA, the pyridine nitrogen atom of the DMAP moiety in 13e coordinated to the ammonium hydrogen which resulted in a change in the energies of the four TSs described above. For the trans-anti-TS (33), this coordination fixed the pyridine ring upon the cycloheptanone to generate a severe steric repulsion between the carbocycle and the pyridine. Also, for the cis-anti TS (35), a similar repulsion between the angular methyl substituent and the coordinated pyridine, accompanied the same repulsion as in TS (31). These facts relatively make the energies of the anti-TSs (33 and 35) higher than those of the syn-TSs (34 and 36). Comparing the two syn-TSs, a steric repulsion between the carbocycle and methyl enamine moiety existed in 36. Based on these reasons, 34 is the relatively favored TS among the four proposed TSs to afford (S)-3b via the rapid dehydration of the corresponding β-hydroxy ketone (Figure 5).
In conclusion, we have established a new enantiodivergent route to provide the Wieland-Miescher ketone analog (3b) bearing a 7-membered ring via the intramolecular aldol reaction of the trione (5) mediated by a single chiral pyridinylmethylamine (13e) in the presence of TFA. Although the enantioselectivities of 3b were moderate, the complete inversion of ee was observed due to the amount of additional TFA. This unique phenomenon was due to the dynamic conformational change of 13e depending on the amount of TFA. The basicity of the nitrogen atom on the pyridine ring was very important for this enantiodivergent behavior. Further work on the details of the reaction mechanism and the developments of a more efficient mediator for the reactions are currently in progress.
EXPERIMENTAL
The 1H NMR spectra and 13C NMR spectra were recorded using a JEOL-AX-400 (1H 400 MHz, 13C 100 MHz) spectrometer and calibrated using tetramethylsilane or CDCl3 as the internal standard. The mass spectra were recorded by a JEOL-DX-303 or JEOL JMS-MS700 spectrometer. The enantiomeric excesses were determined by a HITACHI HPLC LaChrom instrument equipped with a chiral stationary phase column. Optical rotations were measured using a JASCO DIP 370 digital polarimeter.
Typical procedure for reductive pyridinylmethylation of 17.22
A mixture of 17 (2.00 g, 7.99 mmol) and 2-pyridinecarboxaldehyde (18a) (910 mg, 9.59 mmol) in toluene (20 mL) was heated to reflux under azeotropic reaction conditions using a Dean-Stark apparatus for 2 h. After cooling, the toluene was removed under reduced pressure. The residue was dissolved in MeOH (20 mL), then sodium borohydride (360 mg, 9.59 mmol) was added in small portions over 10 min in the ice bath. After removing an ice bath, the mixture was stirred at rt for 4 h. Acetone (10 mL) was then added to the mixture to quench the reaction and the solvent was removed under reduced pressure. The residue was dissolved in AcOEt and washed with saturated aqueous NaHCO3 and brine. After drying the mixture (Na2SO4), the solvent was removed under reduced pressure and the residue was chromatographed (AcOEt/hexane/NH4OH = 20/1/0.2, v/v) to afford 19a 2.53 g (93%) as a pale yellow oil.
(S)-tert-Butyl {1-phenyl-3-[(pyridin-2-ylmethyl)amino]propan-2-yl}carbamate (19a)
[α]D22 +8.4 (c 1.00, CHCl3); 1H-NMR (CDCl3) δ 1.41 (s, 9H), 1.98 (brs, 1H), 2.62 (dd, J = 6.3 Hz, 12.1 Hz, 1H), 2.67 (dd, J = 4.8 Hz, 12.1 Hz, 1H), 2.77 (dd, J = 7.7 Hz, 13.5 Hz, 1H), 2.86-3.00 (brm, 1H), 3.82-3.90 (brm, 1H), 3.84 (d, J = 14.0 Hz, 1H), 3.91 (d, J = 14.0 Hz, 1H), 4.97 (brs, 1H), 7.13-7.30 (m, 7H), 7.62 (dt, J = 1.9 Hz, 7.7 Hz, 1H), 8.55 (d, J = 4.8 Hz, 1H); 13C-NMR (CDCl3) δ 28.2, 38.8, 51.2, 54.9, 78.8, 121.8, 122.1, 126.1, 128.2, 129.2, 136.2, 138.0, 149.1, 155.5, 159.5; EIMS (m/z) 341 (M+), 284, 121 (100%), 92, 57; HRMS calcd for C20H27N3O2 341.2103. Found 341.2098.
(S)-tert-Butyl {1-phenyl-3-[(pyridin-3-ylmethyl)amino]propan-2-yl}carbamate (19b)
Yield: 62% (as a yellow oil); [α]D22 +8.8 (c 1.00, CHCl3); 1H-NMR (CDCl3) δ 1.41 (s, 9H), 1.72 (brs, 1H, 2.59 (dd, J = 6.8 Hz, 12.1 Hz, 1H), 2.67 (dd, J = 4.8 Hz, 12.1 Hz, 1H), 2.76 (dd, J = 7.2 Hz, 12.6 Hz, 1H), 2.80-2.91 (brm, 1H), 3.72 (d, J = 13.5 Hz, 1H), 3.80 (d, J = 13.5 Hz, 1H) 3.96 (brs, 1H), 4.68 (brs, 1H), 7.16-7.24 (m, 6H), 7.63 (d, J = 7.7 Hz, 1H), 8.49 (d, J = 4.8 Hz, 1H), 8.52 (s, 1H); 13C-NMR (CDCl3) δ 28.2, 38.9, 50.7, 51.0, 51.4, 79.0, 123.1, 126.1, 128.2, 128.4, 129.1, 135.6, 137.8, 148.2, 149.4, 155.5; EIMS (m/z) 341 (M+), 284, 121 (100%), 92, 57; HRMS calcd for C20H27N3O2 341.2103. Found 341.2110.
(S)-tert-Butyl {1-phenyl-3-[(pyridin-4-ylmethyl)amino]propan-2-yl}carbamate (19c)
Yield: 63% (as a yellow oil); [α]D23 +3.1 (c 1.00, CHCl3); 1H-NMR (CDCl3) δ 1.41 (s, 9H), 1.60-1.80 (brm, 1H), 2.59 (dd, J = 6.8 Hz, 12.1 Hz, 1H), 2.66 (dd, J = 4.8 Hz, 12.1 Hz, 1H), 2.77 (dd, J = 6.8 Hz, 13.0 Hz, 1H), 2.82-2.93 (brm, 1H), 3.74 (d, J = 14.5 Hz, 1H), 3.80 (d, J = 14.5 Hz, 1H), 3.97 (brs, 1H), 4.67 (brs, 1H), 7.10-7.40 (m, 7H), 8.52 (d, J = 6.3 Hz, 2H); 13C-NMR (CDCl3) δ 28.2, 28.3, 39.2, 51.7, 52.4, 79.4, 121.5, 122.9, 126.5, 128.5, 128.6, 129.3, 137.8, 138.0, 149.8, 149.9, 155.7; EIMS (m/z) 341 (M+), 284, 121 (100%), 92, 57; HRMS calcd for C20H27N3O2 341.2103. Found 341.2106.
(S)- tert-Butyl {1-({[6-methylpyridin-2-yl)methyl]amino}-3-phenylpropan-2-yl}carbamate (19d)
Yield: 52% (as a yellow oil); [α]D22 +2.7 (c 1.00, CHCl3); 1H-NMR (CDCl3) δ 1.42 (s, 9H), 1.95 (brs, 1H), 2.55 (s, 3H), 2.60 (dd, J = 6.3 Hz, 12.6 Hz, 1H), 2.66 (dd, J = 4.8 Hz, 12.0 Hz, 1H), 2.75 (dd, J = 8.2 Hz, 14.0 Hz, 1H), 2.83-3.00 (brm, 1H), 3.79 (d, J = 14.0 Hz, 1H), 3.87 (d, J = 14.0 Hz, 1H), 3.76-3.98 (brm, 1H), 5.03 (s, 1H), 7.02 (t, J = 8.7 Hz, 2H), 7.12-7.32 (m, 5H), 7.51 (t, J = 7.7 Hz, 1H); 13C-NMR (CDCl3) δ 24.5, 28.4, 39.0, 51.2, 51.7, 55.0, 79.1, 119.2, 121.5, 126.3, 128.3, 129.4, 136.7, 138.2, 155.7, 158.0, 158.9; EIMS (m/z) 355 (M+), 135 (100%), 106, 57; HRMS calcd for C21H28N3O2 355.2260. Found 355.2263.
(S)-tert-Butyl {1-{{[4-(dimethylamino)pyridin-2-yl]methyl}amino}-3-phenylpropan-2-yl}carbamate (19e)
Yield: 68% (as an orange colored oil); [α]D21 +1.7 (c 1.00, CHCl3); 1H-NMR (CDCl3) δ 1.41 (s, 9H), 2.05 (brs, 1H), 2.61 (dd, J = 6.3 Hz, 12.5 Hz, 1H), 2.65 (dd, J = 4.8 Hz, 12.5 Hz, 1H), 2.76 (dd, J = 7.7 Hz, 13.0 Hz, 1H), 2.83-3.04 (brm, 1H), 2.99 (s, 6H), 3.72 (d, J = 14.0 Hz, 1H), 3.79 (d, J = 14.0 Hz, 1H), 3.89 (brs, 1H), 5.10 (brs, 1H), 6.38 (dd, J = 2.4 Hz, 5.9 Hz, 1H), 6.46 (d, J = 2.4 Hz, 1H), 7.12-7.31 (m, 5H), 8.17 (d, J = 5.9 Hz, 1H); 13C-NMR (CDCl3) δ 28.3, 28.4, 39.1, 51.1, 51.7, 55.5, 78.9, 104.7, 104.8, 105.11, 105.13, 126.2, 128.3, 129.4, 138.3, 149.3, 154.8, 155.6, 159.4; EIMS (m/z) 384 (M+), 311, 164 (100%), 135, 91, 57; HRMS calcd for C22H32N4O2 384.2525. Found 384.2513.
Direct methylation of 19a using MeI.22
To a stirred solution of 19a (2.50 g, 7.33 mmol) in MeCN (27 mL) was added MeI (1.36 mL, 22.0 mmol) at rt. After stirring at the same temperature for 12 h, the solvent was removed under reduced pressure. The residue was dissolved in AcOEt and the mixture was washed with saturated aqueous NaHCO3 and brine. After drying the mixture (Na2SO4), the solvent was removed under reduced pressure and the residue was chromatographed (AcOEt/hexane/NH4OH = 10/10/0.03, v/v) to afford 20a 1.00 g (39%) as a pale yellow oil.
(S)-tert-Butyl {1-[methyl(pyridin-2-ylmethyl)amino]-3-phenylpropan-2-yl}carbamate (20a)
[α]D21 +1.7 (c 1.00, CHCl3); 1H-NMR (CDCl3) δ 1.43 (s, 9H), 2.27 (s, 3H), 2.40 (d, J = 7.2 Hz, 2H), 2.79 (dd, J = 6.3 Hz, 13.5 Hz, 1H), 2.84-3.00 (m, 1H), 3.62 (d, J = 14.5 Hz, 1H), 3.72 (d, J = 14.5 Hz, 1H), 3.94 (brs, 1H), 4.97 (brs, 1H), 7.14-7.28 (m, 6H), 7.38 (d, J = 7.7 Hz, 1H), 7.64 (dt, J = 1.9 Hz, 7.7 Hz, 1H), 8.54 (d, J = 4.8 Hz, 1H); 13C-NMR (CDCl3) δ 28.3, 38.9, 42.7, 49.6, 59.9, 63.7, 78.9, 121.9, 122.9, 126.1, 128.1, 129.4, 136.3, 138.0, 148.9, 155.7, 159.1; EIMS (m/z) 355 (M+), 135 (100%), 92, 57; HRMS calcd for C21H29N3O2 355.2260. Found 355.2211.
Typical procedure for Eschweiler-Clarke reaction of 19.22
To a stirred solution of 19b (3.51 g, 10.3 mmol) and formalin (37 w/v %, 3.10 mL, 30.8 mmol) in MeOH (25 ml) was added NaBH4 (776 mg, 20.5 mmol) in an ice bath. After stirring at the same temperature for 30 min, the same amounts of formalin and NaBH4 were added every 30 min for three times. The mixture was stirred at rt for 12 h after the final addition and the solvent was removed under reduced pressure. The residue was dissolved in AcOEt and the mixture was washed with saturated aqueous NaHCO3 and brine, and then dried (Na2SO4). The solvent was removed under reduced pressure and the residue was chromatographed (AcOEt/hexane/NH4OH = 50/50/0.4, v/v) to afford 20b 3.08 g (85%) as a pale yellow oil.
(S)-tert-Butyl {1-[methyl(pyridin-3-ylmethyl)amino]-3-phenylpropan-2-yl}carbamate (20b)
[α]D24 +12.1 (c 1.00, CHCl3); 1H-NMR (CDCl3) δ 1.43 (s, 9H), 2.18 (s, 3H), 2.34 (dd, J = 7.2 Hz, 12.6 Hz, 1H), 2.38 (dd, J = 7.7 Hz, 12.6 Hz, 1H), 2.80-2.92 (m, 2H), 3.44 (d, J = 13.5 Hz, 1H), 3.54 (d, J = 13.5 Hz, 1H), 3.99 (brs, 1H), 4.59 (brs, 1H), 7.15-7.30 (m, 6H), 7.63 (d, J = 7.7 Hz, 1H), 8.48-8.54 (m, 2H); 13C-NMR (CDCl3) δ 28.4, 28.5, 39.0, 42.2, 55.0, 59.3, 79.2, 78.0, 123.3, 126.3, 128.3, 129.5, 134.3, 136.1, 136.5, 137.8, 138.4, 148.6, 150.3, 155.6; EIMS (m/z) 355 (M+), 135 (100%), 92, 57; HRMS calcd for C21H29N3O2 355.2260. Found 355.2263.
(S)-tert-Butyl {1-[methyl(pyridin-4-ylmethyl)amino]-3-phenylpropan-2-yl}carbamate (20c)
Yield: 61% (as a pale yellow oil); [α]D25 +32.0 (c 1.01, CHCl3); 1H-NMR (CDCl3) δ 1.42 (s, 9H), 2.19 (s, 3H), 2.32-2.42 (m, 2H), 2.82-2.92 (brm, 2H), 3.44 (d, J = 14.0 Hz, 1H), 3.54 (d, J = 14.0 Hz, 1H), 3.40-4.05 (brm, 1H), 4.69 (brs, 1H), 7.16-7.30 (m, 7H), 8.52 (dd, J = 1.4 Hz, 4.3 Hz, 2H); 13C-NMR (CDCl3) δ 28.3, 28.4, 39.0, 42.5, 49.3, 56.4, 60.8, 79.1, 121.4, 123.6, 126.3, 128.2, 129.2, 129.4, 137.8, 148.2, 149.6, 155.6; EIMS (m/z) 355 (M+), 135 (100%), 92, 57; HRMS calcd for C21H29N3O2 355.2260. Found 355.2258.
(S)-tert-Butyl {1-{methyl[(6-methylpyridin-2-yl)methyl]amino}-3-phenylpropan-2-yl}carbamate (20d)
Yield: 82% (as a pale yellow oil); [α]D25 +11.8 (c 1.00, CHCl3); 1H-NMR (CDCl3) δ 1.44 (s, 9H), 2.28 (s, 3H), 2.29-2.43 (m, 2H), 2.55 (s, 3H), 2.76 (dd, J = 6.8 Hz, 13.7 Hz, 1H), 2.88-3.02 (brm, 1H), 3.57 (d, J = 14.1 Hz, 1H), 3.72 (d, J = 14.1 Hz, 1H), 3.40-4.00 (brm, 0.5H), 5.29 (brs, 0.5H), 7.00 (d, J = 7.8 Hz, 1H), 7.12-7.28 (m, 6H), 7.52 (t, J = 7.8 Hz, 1H); 13C-NMR (CDCl3) δ 24.4, 28.4, 39.1, 42.9, 50.0, 59.6, 63.7, 79.0, 119.9, 121.5, 126.1, 128.2, 129.6, 136.6, 138.2, 155.9, 157.9, 158.5; EIMS (m/z) 369 (M+), 149 (100%), 106, 57; HRMS calcd for C21H30N3O2 369.2416. Found 369.2429.
(S)-tert-Butyl{1-{{[4-(dimethylamino)pyridin-2-yl]methyl}(methyl)amino}-3-phenylpropan-2-yl}carbamate (20e)
Yield: 79% (as a pale yellow oil); [α]D21 +10.6 (c 1.00, CHCl3); 1H-NMR (CDCl3) δ 1.42 (s, 9H), 2.28 (s, 3H), 2.33 (dd, J = 6.3 Hz, 13.0 Hz, 1H), 2.39 (dd, J = 8.2 Hz, 13.0 Hz, 1H), 2.75 (dd, J = 7.2 Hz, 13.5 Hz, 1H), 2.90-3.10 (m, 1H), 3.00 (s, 6H), 3.48 (d, J = 14.0 Hz, 1H), 3.62 (d, J = 14.0 Hz, 1H), 3.89 (brs, 1H), 5.25 (brs, 1H), 6.40 (dd, J = 2.9 Hz, 6.3 Hz, 1H), 6.62 (brs, 1H), 7.15-7.27 (m, 5H), 8.16 (d, J = 6.3 Hz, 1H); 13C-NMR (CDCl3) δ 28.3, 39.0, 42.8, 49.8, 59.4, 64.1, 78.7, 105.1, 105.2, 126.0, 128.0, 129.4, 138.1, 148.9, 154.8, 155.7, 158.9; EIMS (m/z) 398 (M+), 325, 178 (100%), 135, 91, 57; HRMS calcd for C23H34N4O2 398.2682. Found 398.2698.
Typical procedure for deprotection of Boc group in 20.
To a stirred solution of 20b (3.00 g, 8.40 mmol) in CH2Cl2 (25 mL) was added TFA (8.70 mL, 113 mmol) in an ice bath. After removing the ice bath, the mixture was stirred at rt for 34 h, then extracted with H2O. The combined aqueous layer was basified by granular NaOH and extracted with CH2Cl2. The combined organic layer was washed with saturated aqueous NaHCO3 and brine, then dried (Na2SO4). The solvent was removed under reduced pressure and the residue was chromatographed (MeOH/CHCl3/NH4OH = 10/20/0.1, v/v) to afford 13b 1.60 g (74%) as a pale yellow oil.
(S)-N1-Methyl-3-phenyl-N1-(pyridin-3-ylmethyl)propane-1,2-diamine (13b)
[α]D25 +21.6 (c 1.01, CHCl3); 1H-NMR (CDCl3) δ 1.65 (brs, 2H), 2.18 (s, 3H), 2.32-2.44 (m, 2H), 2.47 (dd, J = 8.7 Hz, 13.5 Hz, 1H), 2.75 (dd, J = 4.8 Hz, 13.5 Hz, 1H), 3.18-3.25 (m, 1H), 3.48 (d, J = 13.0 Hz, 1H), 3.57 (d, J = 13.5 Hz, 1H), 7.19-7.32 (m, 6H), 7.64 (d, J = 7.7 Hz, 1H), 8.50 (dd, J = 1.4 Hz, 4.3 Hz, 1H), 8.54 (d, J = 1.5Hz, 1H); 13C-NMR (CDCl3) δ 42.20, 42.23, 50.1, 60.1, 64.3, 123.3, 126.2, 128.4, 129.2, 134.5, 136.5, 139.2, 148.5, 150.3; EIMS (m/z) 255 (M+), 164, 135, 120, 93 (100%), 92; HRMS calcd for C16H21N3 255.1735. Found 255.1730.
(S)- N1-Methyl-3-phenyl-N1-(pyridin-2-ylmethyl)propane-1,2-diamine (13a)
Yield: 71% (as pale yellow oil); [α]D25 +28.7 (c 1.00, CHCl3); 1H-NMR (CDCl3) δ 1.61 (brs, 2H), 2.28 (s, 3H), 2.40-2.50 (m, 3H), 2.76 (dd, J = 4.3 Hz, 13.5 Hz, 1H), 3.18-3.26 (m, 1H), 3.63 (d, J = 14.0 Hz, 1H), 3.76 (d, J = 15.0 Hz, 1H), 7.13-7.33 (m, 4H), 7.27-7.32 (m, 2H), 7.44 (d, J = 8.2 Hz, 1H), 7.65 (dt, J = 1.4 Hz, 7.2 Hz, 1H), 8.53 (d, J = 4.8 Hz, 1H); 13C-NMR (CDCl3) δ 42.1, 42.9, 50.1, 64.37, 64.40, 121.9, 122.9, 126.1, 128.4, 129.2, 136.4, 139.2, 149.0, 159.4; EIMS (m/z) 255 (M+), 164, 135 (100%), 120, 92; HRMS calcd for C16H21N3 255.1735. Found 255.1745.
(S)-N1-Methyl-3-phenyl-N1-(pyridin-4-ylmethyl)propane-1,2-diamine (13c)
Yield: 73% (as pale yellow oil); [α]D25 +24.0 (c 1.01, CHCl3); 1H-NMR (CDCl3) δ 1.62 (brs, 2H), 2.20 (s, 3H), 2.37 (d, J = 6.3 Hz, 2H), 2.48 (dd, J = 8.8 Hz, 13.7 Hz, 1H), 2.76 (dd, J = 4.9 Hz, 13.7 Hz, 1H), 3.16-3.26 (m, 1H), 3.47 (d, J = 14.1 Hz, 1H), 3.58 (d, J = 14.1 Hz, 1H), 7.19-7.33 (m, 7H), 8.53 (dd, J = 1.5 Hz, 4.4 Hz, 2H); 13C-NMR (CDCl3) δ 42.2, 42.5, 50.1, 61.7, 64.5, 123.7, 126.3, 128.4, 129.2, 139.1, 148.4, 149.8; EIMS (m/z) 255 (M+), 164, 135, 120, 93 (100%), 92; HRMS calcd for C16H21N3 255.1735. Found 255.1722.
(S)-N1-Methyl-N1-[(6-methylpyridin-2-yl)methyl]-3-phenylpropane-1,2-diamine (13d)
Yield: 77% (as a pale yellow oil); [α]D25 +35.3 (c 1.00, CHCl3); 1H-NMR (CDCl3) δ 1.70 (brs, 2H), 2.28 (s, 3H), 2.40 (d, J = 6.0 Hz, 2H), 2.46 (dd, J = 8.8 Hz, 13.2 Hz, 1H), 2.53 (s, 3H), 2.76 (dd, J = 4.5 Hz, 13.2 Hz, 1H), 3.17-3.25 (m, 1H), 3.59 (d, J = 14.1 Hz, 1H), 3.72 (d, J = 14.1 Hz, 1H), 7.01 (d, J = 7.3 Hz, 1H), 7.19-7.29 (m, 6H), 7.54 (t, J = 7.4 Hz, 1H); 13C-NMR (CDCl3) δ 24.2, 42.1, 42.8, 50.0, 64.26, 64.31, 119.5, 121.2, 125.9, 128.2, 129.0, 136.4, 139.2, 157.3, 158.7; EIMS (m/z) 269 (M+), 149 (100%), 106; HRMS calcd for C17H23N3 269.1892. Found 269.1900.
(S)-N1-{[4-(Dimethylamino)pyridin-2-yl]methyl}-N1-methyl-3-phenylpropane-1,2-diamine (13e)
Yield: 51% (as a pale yellow oil); [α]D25 +17.7 (c 1.00, CHCl3); 1H-NMR (CDCl3) δ 1.60 (brs, 2H), 2.30 (s, 3H), 2.40 (d, J = 6.8 Hz, 2H), 2.46 (dd, J = 8.7 Hz, 13.5 Hz, 1H), 2.77 (dd, J = 4.8 Hz, 13.5 Hz, 1H), 2.99 (s, 6H), 3.16-3.25 (m, 1H), 3.51 (d, J = 14.0 Hz, 1H), 3.64 (d, J = 14.0 Hz, 1H), 6.39 (dd, J = 2.4 Hz, 5.8 Hz, 1H), 6.70 (d, J = 2.4 Hz, 1H), 7.19-7.22 (m, 3H), 7.26-7.31 (m, 2H), 8.15 (d, J = 5.8 Hz, 1H); 13C-NMR (CDCl3) δ 39.0, 42.2, 43.1, 50.1, 64.5, 64.7, 105.20, 105.22, 126.0, 128.3, 129.2, 139.4, 149.0, 154.8, 159.3; EIMS (m/z) 298 (M+), 254, 178, 136 (100%), 120, 91, 44; HRMS calcd for C18H26N4 298.2157. Found 298.2158.
Typical procedure for the aldol reaction of 5.
A stirred mixture of 5 (100 mg, 0.476 mmol), 13a (121 mg, 0.476 mmol) and TFA (92 mg, 0.809 mmol) in DMSO (1 mL) was heated at 90 oC for 60 h. After cooling, saturated aqueous NaHCO3 was added to the mixture, then extracted with AcOEt. The combined organic layer was washed with brine and dried (Na2SO4). The solvent was removed under reduced pressure and the residue was chromatographed (AcOEt/hexane = 1/3, v/v) to afford (R)-3b 58 mg (59%) as pale yellow crystals. All of the spectroscopic data were identical to those of (R)-3b previously reported.1a According to the known method,1a the optical purity of the obtained (R)-3b was determined to be 72% ee by HPLC equipped with a chiral stationary phase column. HPLC conditions; column: Chiralpak AS-H, mobile phase: EtOH/hexane = 10/90 (v/v), detection: UV 254 nm, flow rate: 1.0 mL/min, Rt = 9.09 min for (R)-3b and 10.1 min for (S)-3b.
ACKNOWLEDGEMENTS
This work was supported by a Grant-in-Aid for Scientific Research from the Ministry of Education, Culture, Sports, Science and Technology of the Japanese Government.
References
1. For a collection of relevant references consult: (a) T. Nagamine, K. Inomata, Y. Endo, and L. A. Paquette, J. Org. Chem., 2007, 72, 123; CrossRef (b) T. Nagamine, K. Inomata, and Y. Endo, Chem. Pharm. Bull., 2007, 55, 1710; CrossRef (c) T. Nagamine, K. Inomata, and Y. Endo, Heterocycles, 2008, 76, 1191. CrossRef
2. For a recent review of 2a: B. Bradshaw and J. Bonjoch, Synlett, 2012, 23, 337. CrossRef
3. Some selected recent preparations of 1 and/or 2: (a) D. A. Lanfranchi, N. Baldovini, and G. Hanquet, Synthesis, 2008, 3775; (b) D. Almasi, D. A. Alonso, A.-N. Balaguer, and C. Nájera, Adv. Synth. Catal., 2009, 351, 1123; CrossRef (c) K. Mori, T. Katoh, T. Suzuki, T. Noji, M. Yamanaka, and T. Akiyama, Angew. Chem. Int. Ed., 2009, 48, 9652; CrossRef (d) A. Monge-Marcet, R. Pleixats, X. Cattoën, M. W. C. Man, D. A. Alonso, and C. Nájera, New J. Chem., 2011, 35, 2766; CrossRef (e) F. Peng, M. Dai, A. R. Angeles, and S. J. Danishefsky, Chem. Sci., 2012, 3, 3076; CrossRef (f) P. Zhou, L. Zhang, S. Luo, and J.-P. Cheng, J. Org. Chem., 2012, 77, 2526; CrossRef (g) G. Tang, U. Gün, and H.-J. Altenbach, Tetrahedron, 2012, 68, 10230; CrossRef (h) A. Monge-Marcet, X. Cattoën, D. A. Alonso, C. Nájera, M. W. C. Man, and R. Pleixats, Green Chem., 2012, 14, 1601; CrossRef (i) A. Bañón-Caballero, G. Guillena, C. Nájera, E. Faggi, R. M. Sebastián, and A. Vallribera, Tetrahedron, 2013, 69, 1307; (j) R. Pedrosa, J. M. Andres, R. Manzano, and C. Pérez-López, Tetrahedron Lett., 2013, 54, 3101; CrossRef (k) C. Xu, L. Zhang, P. Zhou, S. Luo, and J.-P. Cheng, Synthesis, 2013, 45, 1939. CrossRef
4. Some selected recent applications of 1a: (a) S. Yamashita, K. Iso, K. Kitajima, M. Himuro, and M. Hirama, J. Org. Chem., 2011, 76, 2408; CrossRef (b) S. Qian and G. Zhao, Synlett, 2011, 722; (c) K. Michalak and J. Wicha, J. Org. Chem., 2011, 76, 6906; CrossRef (d) M. Adachi, T. Komada, and T. Nishikawa, J. Org. Chem., 2011, 76, 6942; CrossRef (e) C. J. Bungard, G. D. Hartman, J. J. Manikowski, J. J. Perkins, C. Bai, P. E. Brandish, D. H. Euler, J. C. Hershey, A. Schmidt, Y. Fang, R. T. Norcross, T. H. Rushmore, C. D. Thompson, and R. S. Meissner, Bioorg. Med. Chem., 2011, 19, 7374; CrossRef (f) T. Komada, M. Adachi, and T. Nishikawa, Chem. Lett., 2012, 41, 287; CrossRef (g) N. Kotoku, Y. Sumii, T. Hayashi, S. Tamura, T. Kawachi, S. Shiomura, M. Arai, and M. Kobayashi, ACS Med. Chem. Lett., 2012, 3, 673; (h) K.-W. Tsao, C.-Y. Cheng, and M. Isobe, Org. Lett., 2012, 14, 5274; CrossRef (i) G. Mehta and S. Yaragorla, Tetrahedron Lett., 2013, 54, 549; CrossRef (j) D. Minato, B. Li, D. Zhou, Y. Shigeta, N. Toyooka, H. Sakurai, K. Sugimoto, H. Nemoto, and Y. Matsuya, Tetrahedron, 2013, 69, 8019. CrossRef
5. Some selected recent applications of 1b: (a) T. P. Brady, S. H. Kim, K. Wen, C. Kim, and E. A. Theodorakis, Chem. Eur. J., 2005, 11, 7175; CrossRef (b) N. Arai, H. Ui, S. Omura, and I. Kuwajima, Synlett, 2005, 1691.
6. Some selected recent applications of 2a: (a) S. Hanessian, N. Boyer, G. J. Reddy, and B. Deschênes-Simard, Org. Lett., 2009, 11, 4640; CrossRef (b) K. Ma, C. Zhang, M. Liu, Y. Chu, L. Zhou, C. Hu, and D. Ye, Tetrahedron Lett., 2010, 51, 1870; CrossRef (c) V. M. T. Carneiro, H. M. C. Ferraz, T. O. Vieira, E. E. Ishikawa, and L. F. Silva, Jr., J. Org. Chem., 2010, 75, 2877; CrossRef (d) Y.-S. Lu and X.-S. Peng, Org. Lett., 2011, 13, 2940; CrossRef (e) J.-A. Richard and D. Y.-K. Chen, Eur. J. Org. Chem., 2012, 484; CrossRef (f) D. B. Ushakov, A. Raja, R. Franke, F. Sasse, and M. E. Maier, Synlett, 2012, 23, 1358; CrossRef (g) K. Viswanathan, S. N. Ononye, H. D. Cooper, M. K. Hadden, A. C. Anderson, and D. L. Wright, Bioorg. Med. Chem. Lett., 2012, 22, 6919; CrossRef (h) M. Enomoto, A. Morita, and S. Kuwahara, Angew. Chem. Int. Ed., 2012, 51, 12833; CrossRef (i) M. E. Jung and M. Guzaev, J. Org. Chem., 2013, 78, 7518. CrossRef
7. Some selected recent applications of 2b: (a) M. Scheck, M. A. Koch, and H. Waldmann, Tetrahedron, 2008, 64, 4792; CrossRef (b) T. Ling, J. Xu, R. Smith, A. Ali, C. L. Cantrell, and E. A. Theodorakis, Tetrahedron, 2011, 67, 3023; CrossRef (c) H. Hagiwara, T. Nakamura, T. Hoshi, and T. Suzuki, Green Chem., 2011, 13, 1133; CrossRef (d) D. J. Burns, S. Mommer, P. O’Brien, R. J. K. Taylor, A. C. Whitwood, and S. Hachisu, Org. Lett., 2013, 15, 394; CrossRef (e) H. Hagiwara, N. Honma, K. Kinugawa, S. Sato, T. Hoshi, and T. Suzuki, Nat. Prod. Commun., 2013, 8, 873.
8. Some selected reviews of the organocatalytic aldol reactions: (a) B. M. Trost and C. S. Brindle, Chem. Soc. Rev., 2010, 39, 1600; CrossRef (b) A. Moyano and R. Rios, Chem. Rev., 2011, 111, 4703. CrossRef
9. (a) R. Selvarajan, J. P. John, K. V. Narayanan, and S. Swaminathan, Tetrahedron, 1966, 22, 949; CrossRef (b) D. Rajagopal, R. Narayanan, and S. Swaminathan, Proc. Indian Acad. Sci., 2001, 113, 19; CrossRef (c) R. Malathi, D. Rajagopal, Z. G. Hajos, and S. Swaminathan, J. Chem. Sci., 2004, 116, 159. CrossRef
10. (a) J. S. Dutcher, J. G. Macmillan, and C. H. Heathcock, J. Org. Chem., 1976, 41, 2663; CrossRef (b) H. Hagiwara and H. Uda, J. Org. Chem., 1988, 53, 2308; CrossRef (c) X. Wang, S. C. Butler, J. C. Gallucci, and L. A. Paquette, J. Org. Chem., 2009, 74, 6825. CrossRef
11. (a) S. F. Brady, M. P. Singh, J. E. Janso, and J. Clardy, J. Am. Chem. Soc., 2000, 122, 2116; CrossRef (b) S. F. Brady, S. M. Bondi, and J. Clardy, J. Am. Chem. Soc., 2001, 123, 9900; CrossRef (c) F. Marion, D. E. Williams, B. O. Patrick, I. Hollander, R. Mallon, S. C. Kim, D. M. Roll, L. Feldberg, R. V. Soest, and R. J. Andersen, Org. Lett., 2006, 8, 321. CrossRef
12. K. Inomata and Y. Endo, Heterocycles, 2014, 88, 997. CrossRef
13. T. Nagamine, K. Inomata, and Y. Endo, to be submitted.
14. K. Ishihara and K. Nakano, J. Am. Chem. Soc., 2005, 127, 10504. CrossRef
15. A. S. Kumar, B. Haritha, and B. V. Rao, Tetrahedron Lett., 2003, 44, 4261. CrossRef
16. O. Mitsunobu, Synthesis, 1981, 1. CrossRef
17. T. Hunt, H. C. Atherton-Watson, S. P. Collingwood, K. J. Coote, S. Czarnecki, H. Danahay, C. Howsham, P. Hunt, D. Paisley, and A. Young, Bioorg. Med. Chem. Lett., 2012, 22, 2877. CrossRef
18. Aldehydes (18a-18d) were commercially available.
19. Preparation of 18e: R. López-Rodríguez, A. Ros, R. Fernández, and J. M. Lassaletta, J. Org. Chem., 2012, 77, 9915. CrossRef
20. (a) W. Eschweiler, Ber., 1905, 38, 880; CrossRef (b) H. T. Clarke, H. B. Gillespie, and S. Z. Weisshaus, J. Am. Chem. Soc., 1933, 55, 4571; CrossRef (c) A. Gopin, C. Rader, and D. Shabat, Bioorg. Med. Chem., 2004, 12, 1853. CrossRef
21. (a) D. R. Lide ‘CRC Handbook of Chemistry and Physics, 75th ed.’, CRC Press, London, 1994; (b) L. A. Paquette ‘Encyclopedia of Reagents for Organic Synthesis’, John Wiley & Sons, New York, 1995.
22. Some peaks of the new compounds (19-20) in 1H-NMR spectra were observed as the very broad signals. Also, there were some slightly different signals due to the isomers in 13C-NMR spectra. Since no other signals due to the isomers in 13 were observed after the deprotection of Boc group, these results suggested that we detected the corresponding rotamers based on the Boc group in 19-20.