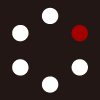
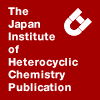
HETEROCYCLES
An International Journal for Reviews and Communications in Heterocyclic ChemistryWeb Edition ISSN: 1881-0942
Published online by The Japan Institute of Heterocyclic Chemistry
e-Journal
Full Text HTML
Received, 4th August, 2014, Accepted, 14th October, 2014, Published online, 20th October, 2014.
DOI: 10.3987/COM-14-S(K)102
■ Synthesis of 2,7-Disubstituted 5,10-Diaryl-5,10-dihydrophenazines via Iron-Catalyzed Intramolecular Ring-Closing C–H Amination
Yuma Aoki, Ryuji Imayoshi, Takuji Hatakeyama, Hikaru Takaya, and Masaharu Nakamura*
International Research Center for Elements Science, Institute for Chemical Research, Kyoto University, Gokasho, Uji, Kyoto, 611-0011, Japan
Abstract
A novel iron-catalyzed intramolecular ring-closing C–H amination reaction of o-phenylenediamines was developed, affording the corresponding 2,7-disubstituted 5,10-diaryl-5,10-dihydrophenazines in acceptable yields. The reaction proceeded via the in-situ generation of the magnesium amides of the starting secondary amines in the presence of a catalytic amount of an iron salt and a stoichiometric amount of 1,2-dibromoethane as the terminal oxidant. The substituted dihydrophenazine derivatives can potentially be used as hole-injection materials in organic electroluminescence (OEL) devices and also offer scaffolds for further synthetic elaborations of OEL materials.Nitrogen-containing polycyclic aromatic compounds are of significant interest because of their versatile redox properties and applications in organic electronic materials,1 and pharmaceuticals as biologically active ingredients.2 5,10-Diaryl-5,10-dihydrophenazines have promising physical properties3,4 and attract considerable attention because of their potential use as hole-injection materials in organic electroluminescence (OEL) devices.3 The synthesis of 5,10-diaryl-5,10-dihydrophenazines has been mainly carried out by the arylation reaction of the parent phenazine or 5,10-dihydrophenazine. In 1957, Gilman and Dietrich reported the synthesis of 5,10-diphenyl-5,10-dihydrophenazine via the copper-catalyzed cross-coupling reaction of iodobenzene with 5-phenyldihydrophenazinyllithium, which was prepared by the addition of phenyllithium to phenazine.4 In 2003, Okamoto et al. developed an efficient synthetic method for 5,10-diaryl-5,10-dihydrophenazines: the palladium-catalyzed double-arylation reactions (Buchwald–Hartwig reaction5) of 5,10-dihydrophenazine afforded symmetrical compounds and the sequential N-arylation with aryllithium addition and the Buchwald–Hartwig reaction of the resulting 5-aryl-5,10-dihydrophenazine provided unsymmetrical compounds possessing different aryl groups on the nitrogen atoms.6
Although diverse aryl groups can be introduced on the nitrogen atoms using these N-arylation methods, the regioselective functionalization of the core aromatic units of 5,10-diaryl-5,10-dihydrophenazines has been an arduous task: The classical Wohl–Aue reaction needs harsh conditions (high temperature and strong base) and usually results in a low yield of the desired substituted phenazines.7 The thermal decomposition of tetraarylhydrazine provides the core-substituted 5,10-diaryl-5,10-dihydrophenazines as a mixture of the target compounds, the corresponding diarylamines, and the oligomers of the diarylamines.8 Phenazine and dihydrophenazine substrates bearing core substituents can be synthesized via the transition-metal-catalyzed cross-coupling reactions of aniline derivatives.9 However, these syntheses also require the multistep and regioselective preparation of polysubstituted aniline precursors.
Because of the lack of a suitable direct core-functionalization method, an alternative approach to core-substituted dihydrophenazine derivatives can involve the intramolecular C–H amination of o-phenylenediamines bearing aryl groups at appropriate positions (Scheme 1). Despite the recent remarkable advances in intramolecular C–H amination reactions,10,11 no such catalytic C–H amination reaction for 5,10-diaryl-5,10-dihydrophenazines has been reported to the best of our knowledge, probably because of the instability of the densely arylated diamine precursors and overoxidation of the dihydrophenazine products under conventional oxidative conditions.12 In this communication, we report our preliminary findings on the first catalytic intramolecular C–H amination of o-phenylenediamines that affords 2,7-disubstituted 5,10-diaryl-5,10-dihydrophenazines. The key to the success of the reaction is the conversion of o-phenylenediamines to the corresponding magnesium amides and the use of an iron catalyst in the presence of 1,2-dibromoethane as an oxidant.
The study commenced with a screening of reaction conditions for the conversion of N1,N1,N2-triphenyl- o-phenylenediamine (1a) to 5,10-diphenyl-5,10-dihydrophenazine (2a). Both the compounds were obtained as by-products during our previous study on iron-catalyzed amination reaction,13 and we envisaged that iron would catalyze the ring-closing C–H amination reaction of 1a. The reaction was thus investigated, and the screening of reaction conditions and reagents identified two different procedures as shown in Table 1. In procedure A, a solution of BuMgBr in Bu2O was added to a solution of 1a in Bu2O at 0 °C to generate the magnesium amide of the secondary amine (NH). After stirring the mixture at 100 °C for 1 h to complete the deprotonation, a catalytic amount of an iron salt and 2.0 equiv of 1,2-dibromoethane were added to the resulting magnesium amide. The C–H amination reactions were found to proceed at 80 °C with a reasonable rate of conversion although the reactions ceased when 33–50% of 1a was consumed. In procedure B, a mixture of 1a, lithium bis(trimethylsilyl)amide LiN(SiMe3)2, MgBr2, an iron salt, and 1,2-dibromoethane in cyclopentyl methyl ether, hereafter abbreviated as CPME, was heated at 100 °C for 15 to 21 h to obtain 2a up to 39% yield. The reactions were monitored by GC and quenched when the reaction stopped.
Table 1 summarizes the results of the ring-closing C–H amination reactions of 1a. Although the reactions using ferrous halides, FeCl2 or FeBr2, as the catalyst did not give the desired dihydrophenazine at all (data not shown), the ferric halide catalysts, FeCl3 and FeBr3, promoted the reaction to afford 2a in 15% and 19% yields, respectively (entries 1 and 2). The yields are almost the same for FeCl3 and FeBr3, while a slightly improved material balance was achieved with the latter catalyst. An increase in catalyst loading from 5 mol% to 20 mol% did not improve the yield of 2a significantly (entry 3). Because the conversion of 1a to 2a requires the elimination of hydrogen atoms, probably as protons, protonation of the starting magnesium amide may compete during the reaction to decrease the yield of 2a. Therefore, an additional base was employed in procedure B, in which an excess amount of the magnesium amide base was generated using 2.0 equiv of LiN(SiMe3)2 and MgBr2. In this procedure, 5 mol% of FeBr3 afforded the desired product in 12% yield (entry 4). Notably, FeCl2 was found to work well in procedure B, affording 2a in 11% yield with an improved material balance (entry 5). Finally, the yield of 2a was improved to 39% with an increased amount of FeCl2 (20 mol%) (entry 6). Some unidentified by-products were observed, particularly in the low material balance cases, and these may be oligomers of 1a, as inferred by the GPC analyses of the crude products (data not shown).
Diverse o-phenylenediamine substrates 1b–1e were subjected to the ring-closing amination conditions (procedure A or B), and the results are summarized in Table 2. Under conditions of procedure B, the reaction of methyl substrate 1b afforded 2,7-dimethyl-5,10-di-p-tolyl-5,10-dihydrophenazine (2b) in 39% yield (entry 1). Fluoro substrate 1c showed high reactivity: the ring-closing reaction proceeded at 70 °C to afford a new compound, 2,7-difluoro-5,10-bis(4-fluorophenyl)-5,10-dihydrophenazine (2c), albeit in a low yield (20%) because the oligomerization of 1c competed with the desired reaction (entry 2). The reaction of chloro substrate 1d afforded 2,7-dichloro-5,10-bis(4-chlorophenyl)-5,10-dihydrophenazine (2d), in 27% yield under conditions of procedure B (entry 3). Under the reaction conditions, the dechlorination reaction was observed to compete with the C–H amination to some extent, making the isolation of the pure product difficult. Therefore, procedure A was applied to this reaction, and 2d was obtained in 35% yield in an analytically pure form (entry 4).14 Although procedure B did not work well with bromo substrate 1e because of the undesired debromination reaction, 2,7-dibromo-5,10-bis(4-bromophenyl)-5,10-dihydrophenazine (2e) was obtained in 22% yield using procedure A (entry 5).
Although the mechanism of the C–H amination reaction is unclear at present, presumably this reaction proceeds via the formation of an iron-amide species,15 followed by C–H bond cleavage and C–N bond formation reactions. Scheme 2 shows two possible reaction pathways: (i) electrophilic amination pathway and (ii) C–H activation/reductive elimination pathway. In the former pathway, the electrophilic addition of the nitrogen of the iron-amide species to the neighboring arene ring (electrophilic amination)16 generates a cationic intermediate A, which provides the dihydrophenazine product upon the release of the iron species and a proton. In the latter pathway, a C–H bond of the arene ring is activated by the iron catalyst to generate a ferracycle17 by oxidative addition, or more probably by a concerted metalation–deprotonation mechanism,18 followed by reductive elimination to afford the dihydrophenazine. The reduced iron species may undergo two-electron oxidation by 1,2-dibromoethane to regenerate the catalytically active high-valent iron species.
In summary, we developed a novel iron-catalyzed intramolecular ring-closing C–H amination of o-phenylenediamines. This reaction provides a facile access to diverse 5,10-diaryl-5,10-dihydrophenazines with the regioselective installation of methyl, fluoro, chloro, and bromo substituents at the 2,7-positions of phenazine core. Some of these products are amenable to further synthetic elaborations such as the extension of π-conjugation and introduction of additional amino groups or heteroatoms by standard cross-coupling reactions. Further study is underway to elucidate the mechanism of the reaction and to improve the reaction efficiency.
ACKNOWLEDGEMENTS
This research was supported by the Japan Society for the Promotion of Science (JSPS) through the “Funding Program for Next-Generation World-Leading Researchers (NEXT Program),” initiated by the Council for Science and Technology Policy (CSTP) and also supported in part by the Japan Science and Technology Agency (JST), the Core Research for Evolutional Science and Technology (CREST 1102545) Program, and MEXT program “Elements Strategy Initiative to Form Core Research Center.” We are grateful to Tosoh Organic Chemical Co., Ltd., Nissan Chemical Industry Ltd., and JX Nippon Oil & Energy Corporation for financial support. The generous gift of CPME from Zeon Corporation is acknowledged. We also thank RIKEN for the provision of beam time in SPring-8 (BL14B2: 2013A1798; 2013B1855, BL27Su: 2013A1685; 2013B1115, and BL40XU: 2013B1736; 2014A1717). 1H and 13C NMR measurements (800 and 201 MHz, respectively) were supported by JURC at ICR, Kyoto University.
References
1. (a) Y. Shirota, J. Mater. Chem., 2000, 10, 1; CrossRef (b) Y. Shirota, J. Mater. Chem., 2005, 15, 75; CrossRef (c) Z. Ning and H. Tian, Chem. Commun., 2009, 5483; CrossRef (d) L. Duan, L. Hou, T.-W. Lee, J. Qiao, D. Zhang, G. Dong, L. Wang, and Y. Qiu, J. Mater. Chem., 2010, 20, 6392. CrossRef
2. (a) A. W. Schmidt, K. R. Reddy, and H.-J. Knölker, Chem. Rev., 2012, 112, 3193; CrossRef (b) C. Sánchez, C. Méndez, and J. A. Salas, Nat. Prod. Rep., 2006, 23, 1007. CrossRef
3. E. Terada, T. Okamoto, K. Sato, T. Takui, and K. Okada, J. Org. Chem., 2005, 70, 10073. CrossRef
4. H. Gilman and J. J. Dietrich, J. Am. Chem. Soc., 1957, 79, 6178. CrossRef
5. (a) J. Paradies, in Metal-Catalyzed Cross-Coupling Reactions and More, ed. by A. de Meijere, S. Bräse, and M. Oestreich, Wiley-VCH, Weinheim, 2014, pp. 995–1066; (b) D. S. Surry and S. L. Buchwald, Chem. Sci., 2011, 2, 27; CrossRef (c) J. Hartwig, Acc. Chem. Res., 2008, 41, 1534. CrossRef
6. T. Okamoto, E. Terada, M. Kozaki, M. Uchida, S. Kikukawa, and K. Okada, Org. Lett., 2003, 5, 373. CrossRef
7. (a) A. Wohl and A. Aue, Chem. Ber., 1901, 34, 2442; CrossRef (b) U. Bahnmüller, W. Keller-Schierlein, M. Brandl, H. Zähner, and H. Diddens, J. Antibiot., 1988, 41, 1552; CrossRef (c) Z. Wong, in Comprehensive Organic Name Reactions and Reagents, John Willey & Sons, Inc. 2009, pp. 3060–3063.
8. F. A. Neugebauer and H. Fischer, Chem. Ber., 1971, 104, 886 and references cited therein. CrossRef
9. For synthesis of phenazines, see: (a) T. Emoto, N. Kubozaki, Y. Yamagiwa, and T. Kamikawa, Tetrahedron Lett., 2000, 41, 355; CrossRef (b) M. Tietze, A. Iglesias, E. Merisor, J. Conrad, I. Klaiber, and U. Beifuss, Org. Lett., 2005, 7, 1549; CrossRef (c) L. Yu, Z. Zhou, D. Wu, and H. Xiang, J. Organomet. Chem., 2012, 705, 75; CrossRef For synthesis of 5,10-dihydrophenazine, see: (d) Z. Hu, W. Ye, H. Zou, and Y. Yu, Synth. Commun., 2010, 40, 222. CrossRef
10. For reviews, see: (a) M.-L. Louillat and F. W. Patureau, Chem. Soc. Rev., 2014, 43, 901; CrossRef (b) B. J. Stokes and T. G. Driver, Eur. J. Org. Chem., 2011, 4071. CrossRef
11. For carbazoles, see: (a) W. C. P. Tsang, N. Zheng, and S. L. Buchwald, J. Am. Chem. Soc., 2005, 127, 14560; CrossRef (b) G. Brasche and S. L. Buchwald, Angew. Chem. Int. Ed., 2008, 47, 1932; CrossRef (c) W. C. P. Tsang, R. H. Munday, G. Brasche, N. Zheng, and S. L. Buchwald, J. Org. Chem., 2008, 73, 7603; CrossRef (d) J. A. Jordan-Hore, C. C. C. Johansson, M. Gulias, E. M. Beck, and M. J. Gaunt, J. Am. Chem. Soc., 2008, 130, 16184; CrossRef (e) S. H. Cho, J. Yoon, and S. Chang, J. Am. Chem. Soc., 2011, 133, 5996; CrossRef (f) K. Takamatsu, K. Hirano, T. Satoh, and M. Miura, Org. Lett., 2014, 16, 2892; CrossRef For quinolinones, see: (g) K. Inamoto, T. Saito, K. Hiroya, and T. Doi, J. Org. Chem., 2010, 75, 3900; CrossRef For imidazoles, see: (h) H. Wang, Y. Wang, C. Peng, J. Zhang, and Q. Zhu, J. Am. Chem. Soc., 2010, 132, 13217; CrossRef (i) X. Wang, Y. Jin, Y. Zhao, L. Zhu, and H. Fu, Org. Lett., 2012, 14, 452; CrossRef For indoles, see: (j) S. Jana, M. D. Clements, B. K. Sharp, and N. Zheng, Org. Lett., 2010, 12, 3736; CrossRef (k) Y. Tan and J. F. Hartwig, J. Am. Chem. Soc., 2010, 132, 3676. CrossRef
12. The oxidation of electron-rich N1,N1,N2-triaryl-o-phenylenediamines by a stoichiometric amount of FeCl3 was reported to afford the corresponding 5,10-diaryl-5,10-dihydrophenazines via the formation of the overoxidized radical cation of the product. See ref 8.
13. T. Hatakeyama, R. Imayoshi, Y. Yoshimoto, S. K. Ghorai, M. Jin, H. Takaya, K. Norisuye, Y. Sohrin, and M. Nakamura, J. Am. Chem. Soc., 2012, 134, 20262. CrossRef
14. A representative procedure (Method A): A solution of BuMgBr in Bu2O (275 µL, 0.799 M, 0.220 mmol) was added to a solution of 1d (95.3 mg, 0.202 mmol) in Bu2O (1.0 mL) at 0 °C. After stirring the mixture at 100 °C for 1 h, FeBr3 (3.0 mg, 10 µmol) and 1,2-dibromoethane (75.8 mg, 0.403 mmol) were added to the resulting solution of magnesium amide at room temperature. The mixture was stirred at 90 °C for 3 h and quenched with 1 N aqueous HCl (0.4 mL) at room temperature. The aqueous layer was extracted with toluene four times. The combined organic extracts were filtered with a pad of Florisil, and then the solvent was removed in vacuo to obtain the crude product (104 mg). A part of the crude product (96.2 mg) was purified by recycling GPC affording 2d as a yellow powder (31.3 mg, 35% yield based on the amount of the purified crude product, 65% yield based on the consumption of 1d, >98% purity on GC analysis). IR (neat): ν cm–1 1481, 1416, 1356, 1323, 1285, 1270, 1258, 1089, 965, 940, 842, 828, 795, 786, 724; mp 303.4–304.5 °C; 1H NMR (C6D6, 392 MHz) δ ppm 5.34 (d, J = 8.5, 2H), 5.79 (d, J = 2.2 Hz, 2H), 6.28 (dd, J = 2.2 Hz, 8.5 Hz, 2H), 6.60 (m, 4H), 6.97 (m, 4H); 13C NMR (C6D6, 98.5 MHz) δ ppm 113.04 (2C), 114.01 (2C), 121.08 (2C), 127.38 (2C), 132.13 (4C), 132.21 (4C), 134.72 (2C), 134.73 (2C), 137.45 (2C), 137.49 (2C); HRMS (FAB) Calcd for C24H14Cl4N2 [M]+: 469.9911. Found: 469.9911; Anal. Calcd for C24H14Cl4N2: C, 61.05; H, 2.99; N, 5.93. Found: C, 61.15; H, 3.04; N, 5.85.
15. M. M. Olmstead, P. P. Power, and S. C. Shoner, Inorg. Chem., 1991, 30, 2547. See also ref. 13. CrossRef
16. L. A. Paquette, J. Am. Chem. Soc., 1963, 85, 3288. CrossRef
17. T. Matsubara, S. Asako, L. Ilies, and E. Nakamura, J. Am. Chem. Soc., 2014, 136, 646. CrossRef
18. D. Lapointe and K. Fagnou, Chem. Lett., 2010, 39, 1118. CrossRef