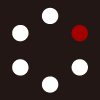
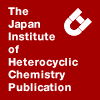
HETEROCYCLES
An International Journal for Reviews and Communications in Heterocyclic ChemistryWeb Edition ISSN: 1881-0942
Published online by The Japan Institute of Heterocyclic Chemistry
e-Journal
Full Text HTML
Received, 14th March, 2014, Accepted, 11th April, 2014, Published online, 15th April, 2014.
DOI: 10.3987/REV-14-795
■ Reductive Cyclization Reactions to Bicyclic Compounds Using Samarium Diiodide
Motoo Tori* and Masakazu Sono
Department of Analytical Chemistry, Faculty of Pharmaceutical Sciences, Tokushima Bunri University, 180 Nishihamabouji, Yamashiro-machi, Tokushima, 770-8514, Japan
Abstract
This review covers reports on the one-electron reductive cyclization reactions affording bicyclic compounds, such as hydrindans, perhydronaphthalenes, perhydroguaianes, and other systems including five-, six-, seven-, and eight-membered carbocycles using SmI2. The substrate is aldehyde, ketone, or ester. The effect of additives, such as H2O, MeOH, HMPA, and NiI2, was studied. The mechanistic aspects recently investigated are also introduced.CONTENTS
1. Introduction
2. Cyclization to hydrindans
3. Cyclization to perhydronaphthalenes
3.1. Enone in the ring
3.2. Enone in the side chain
4. Cyclization to perhydroguaianes
5. Cyclization to other systems
5.1. 6,7-Fused ring systems
5.2. 6,8-Fused ring systems
5.3. Spiro systems
5.4. Esters
5.5. Comparison with electrolysis
5.6. Application to the synthesis of coronafacic acid
5.7. Tricyclic systems
5.8. 5,11-Fused ring systems
6. Mechanistic studies
7. Conclusion
1. INTRODUCTION
Samarium diiodide (SmI2) has been used in organic synthesis because it is easy to use, highly selective, very powerful, and because its power can be changed by the use of additives, such as H2O, MeOH, t-BuOH, HMPA, and NiI2.1 SmI2 has been utilized for carbon-carbon bond-forming reactions such as between ketone and halide (eq. 1, Scheme 1), between ketones (eq. 2), or between ketone and α,β-unsaturated carbonyl to afford lactone (eq. 3).1
We are especially interested in carbon-carbon bond-forming reactions at both the α- and β-positions of α,β-unsaturated carbonyl compounds. The intramolecular versions of these types of reactions (eqs. 4 and 5) are the main topic of this review. Depending on the size of ‘m’ and/or ‘n’, hydrindans, perhydronaphthalenes, perhydroguaianes, and other systems can be established. These reactions can also be applicable to the synthesis of natural products, such as eremophilanes and guaianes. One-electron reduction of a carbonyl group by SmI2 affords a radical anion, which adds to a double bond or a carbonyl group (‘carbonyl-first mechanism’).1 However, in the case of α,β-unsaturated carbonyl compounds, substrates can be recognized as an alkene substituted with an alkoxycarbonyl group. Therefore, it is thought that the carbonyl is first reduced to afford a radical anion and is then added to the double bond of the alkene substituted with an alkoxycarbonyl group at the β-position of the carbonyl group. On the other hand, if the α,β-unsaturated carbonyl compound is first reduced, a radical is formed at the β-position of the carbonyl group. This radical can attack the carbonyl group to form a new carbon-carbon bond. This is called the ‘alkene-first mechanism’ by Procter.1 These points will be discussed later. Special attention should be paid to the stereochemistry of the products in eq. 5. When the newly created hydroxy group (OH) and R2 (H or Me) are in the same direction, this is called a syn relationship, while in the opposite direction, an anti relationship. Please note that this is not due to the relation of a fused-ring, for example, cis- or trans-hydrindan. Most reactions were conducted at rt or 0 ˚C and 3 equivalents of SmI2 were used in general. The amount of SmI2 was increased when the reaction was slow.
Kagan,2-5 Curran,6-8 Molander,9-12 Little,13 Flowers, II,14,15 and Procter1,16,17 have published reviews on similar subjects. These authors concentrated on a narrow area, cyclization to bicyclic systems, covering reports published in approximately the past ten years, and touched on the mechanism obtained recently by DMPO and ESR methods. The ring-opening fragmentation reaction was not included.18
2. CYCLIZATION TO HYDRINDANS
Hydrindans are synthesized by coupling between enone and aldehyde moieties, as shown in Table 1.19 Three chiral centers are created from 1 by this reaction in one step to afford four kinds of products. When the reaction was conducted without additives, syn compound 2, the configurations of which were all β-oriented, was the predominant product. When MeOH was added as the additive, the ratio of compound 2 slightly decreased. However, the product ratio was almost the same as without the additive. Addition of HMPA decreased the yield of these products, presumably due to the isolation procedure. In any case the ratio of syn (2 and 3):anti (4 and 5) was ca. 9:1.
Two methyl groups were added to the cyclopentanone ring and similar reactions were carried out (Table 2).19 The stereochemistries of the products were almost the same as those in Table 1. Without an additive, syn compounds 7 and 8 predominated, and with MeOH as the additive, the ratio of compound 9 increased. The tendency was for the major product to have a syn configuration involving the hydroxy group and the hydrogen at the juncture position, while in the case of compound 9, the relationship was anti. Compounds 7 and 8 belong to the same group because the juncture position next to the carbonyl group is created on the quenching of samarium enolate. cis-Fused hydrindans are usually produced rapidly due to their stability. The ratio of syn:anti was ca. 7:3–9:1.
One methyl group was introduced at the side chain because the substrate was chosen aiming at the synthesis of botrydial.19 Compound 10 was subjected to the reaction with SmI2 (Table 3). The major product was compound 11, which again had a syn relationship involving the hydroxy group and the hydrogen at the juncture position. When MeOH was added, the ratio of compound 11 decreased and that of compound 12 increased. Compounds 11 and 12 have different stereochemistry of the hydrogen adjacent to the carbonyl group. It is interesting to note that over reduction to product 14 also occurred in this case. This means that SmI2 can reduce ketone to alcohol under these conditions. The ratio of syn:anti was ca. 4:1.
Two substrates, 15 and 21, bearing a vinyl group on the side chain were next studied (Tables 4 and 5).19 In these examples the products are complicated because they have many chiral centers. The products were separated carefully using HPLC and the ratio was determined by GC. In the case of compound 15, the situation was much more complex. The major products, 16 and 17, had a syn configuration and compound 20 arose from the coupling between ketone and aldehyde (Table 4). The product ratio of syn:anti was ca. 1:1–4:1. For compound 21, it was slightly simpler. These results depended on the difference of the substitution pattern of both vinyl and methyl groups. Only syn products were formed in entries 1 and 3, while with MeOH as an additive, the ratio of syn:anti was ca. 1:2 (Table 5). The transition state affording these isomers was considered (Scheme 2).
Conformations B and C must be more stable than A and D because samarium is outside the molecule. It was not clear why the product from conformer C was not produced.
The intramolecular reaction between six-membered enone and aldehyde or ketone was next investigated. Compound 27 had a methyl group at the β-position of the α,β-unsaturated carbonyl group (Table 6). The major product was syn compound 28 and the ratio of syn;anti was ca. 7:3–8:2. The yield was good without an additive or with NiI2. However, in the presence of MeOH or HMPA, yields were not high.20
In the reaction between the ketone and enone in compound 30, yields were also very high in most cases (Table 7).20 The major product was syn compound 31. With HMPA as an additive, the α-position of the α,β-unsaturated carbonyl group reacted to give 33. It was quite surprising that the yields were very high in spite of the coupling between the ketone and the quaternary position. This is presumably because the conformation at the transition state benefited the bond formation in this substrate. The substrates described in Tables 1–5 had no functional group at the juncture position of the products, while compounds in Tables 6 and 7 had methyl groups there.
Another system for constructing hydrindans was reported (Scheme 3).21,22 The intramolecular carbon-carbon bond formation between aldehyde and α,β-unsaturated ester only produced cis-hydrindans, 35, 37, 39, 41, and 43. In most cases, a methoxycarbonyl group was oriented on the same side of the juncture hydrogen, presumably due to the requirement of samarium enolate quenching. The proton can easily be taken from the α-equatorial side. Therefore, compounds bearing β-substituents at the adjacent position more easily took the proton from the α-equatorial side, while in the case of 36, the methyl group on the α-side interfered with the α-attack, and finally a compound bearing a thermodynamically stable α-methoxycarbonyl group was formed. Compound 44 cyclized to hydrindan 45, making two carbon-carbon bonds, one being Dieckmann-type condensation.22 In all cases, sec-hydroxy groups had a syn configuration involving the juncture hydrogen.
3. CYCLIZATION TO PERHYDRONAPHTHALENES
3.1. Enone in the ring
The intramolecular reaction between the six-membered enone and aldehyde or ketone affords perhydronaphthalenes.23 The reaction between aldehyde and the enone system was carried out to form both syn and anti products involving the newly created hydroxy group and the hydrogen at the juncture position (Table 8). Compound 48 was always the major product. The ratio of the product hardly changed depending on the conditions employed. The ratio of syn:anti was ca. 9:1. The fourth isomer 50 was not produced. This tendency was almost the same as that of hydrindans because the predominant transition state was quite similar.
Compound 51 had a methyl group at the β-position of the enone system which was expected to become a juncture substitution in the product.23 The yield depended on the conditions shown in Table 9 and varied markedly. Compound 52 was the sole product without an additive. anti Products, 54 and 55, involving the hydroxy and methyl groups at the juncture were hardly formed in these cases.
Compound 56 produced syn products 57 and 58 as major isomers with additives (Table 10).
Finally, substrate 61 was treated similarly with SmI2 (Table 11).23 Compound 62 was the major product without an additive. However, when MeOH was added, compound 63 was formed predominantly. The transition state must be considered individually to explain these phenomena.
3.2. Enone in the side chain
Matsuda and Shirahama reported SmI2-induced cyclization (with MeOH as an additive) to perhydronaphthalenes.24 They used diastereoisomers with E and Z isomers, 66, 67, 69, and 72, and compared their results (Scheme 4). Although the product from compounds 66 and 67 was only one isomer, their diastereoisomers 69 and 72 afforded different results. Compound 69 produced a mixture of isomers, 70 and 71. Compound 72 afforded 73, as well as 70 and 71. These differences were attributed to the different configurations of the hydroxy group, because SmI2 coordinated both the carbonyl and hydroxy groups in the transition state. This is a marked difference from other cases discussed in this review, especially the compound discussed in Section 6.
4. PERHYDROGUAIANES
Four methylenes were introduced in the side chain to obtain a seven-membered carbocycle. Compound 74 afforded compound 78 as the major product without an additive (Table 12).25 Even when MeOH was added, compound 78 was the major product, which was anti involving the hydroxy group and methyl group at the ring junction. The ratio of syn:anti was ca. 1:1–1:4.
When the carbonyl group was changed to a ketone from an aldehyde, compound 79 did not cyclize, but instead various reduction products were formed (Scheme 5).25
5. OTHER SYSTEMS
5.1. 6,7-Fused ring systems
When compound 81, bearing a ketone and enone, was subjected to the reaction with SmI2, compounds with the ring system 6/7 (82 and 83) were produced in 74% yield (6 eq. of SmI2) (Table 13).25 Unfortunately, the yield did not increase when MeOH was added. When the methyl group was substituted at the β-position of the substrate, five products were formed depending on with or without an additive (Table 14).25 The major product was 86 without an additive or with MeOH. When HMPA was added, a spiro ketol 90 was also formed. This is the product of an anionic aldol-type reaction.
When the substrate was changed to compound 91, cyclization product 92 was obtained in very low yield (Table 15).25 The structure was elucidated by X-ray crystallography. Other products were various reduction products.
5.2. 6,8-Fused ring systems
Challenges to eight-membered compounds were conducted using substrate 96.25 Although the yield was quite low, cyclized compounds 97 and 98 were produced (Table 16). Otherwise, various reduction products were formed.
5.3. Spiro systems
When the side chain is substituted at the β-position of the carbonyl group, such as 103, the product may be a spiro cyclic compound (Scheme 6).26 Compound 103 afforded 104 in 70% yield with diastereoselectivity of 5:1. The substrate with one carbon less substance 105 gave 106 in 75% yield in the ratio of 3:2. The configurations of each hydroxy group were determined by NMR spectroscopy. Several other systems were also described in this paper.
The substrate similar to compounds 15 and 21, but bearing an epoxide instead of enone system, 107, gave three kinds of products, 108, 109, and 110 (Scheme 7).27 These spiro compounds were apparently derived from two-electron reduction followed by the intramolecular aldol reaction. Compound 107 was a mixture of two diastereoisomers and thus two products, 108 and 109, were obtained. The hydroxy group oriented to the carbonyl side, presumably due to the coordination of samarium with both oxygen atoms.
5.4. Esters
Esters are usually not reduced by SmI2; however, they can work as acceptors of Claisen-type or Dieckmann-type condensations.28 When compound 111 was subjected to reduction with SmI2, a simple reduction product 115 was mostly produced with recovery of the starting material (Table 17). Instead, dimerization of the cyclohexenone moiety occurred at the β-position, and then aldol-type carbon-carbon bond formation followed to give compounds 112 and 113.
When the ester was changed to trichloroethyl ester 116, the products were bicyclic diones 118 and 119, on the addition of H2O as an additive, although the yield was 18% (Table 18).28 These results indicated that the radical formed at the β-position of the cyclohexanone moiety was further reduced by SmI2 and the resulting anion attacked the ester part.
MOM ester 117 gave a complex mixture without an additive.28 However, with water as an additive, 118 and 119 were formed in 11% yield (Table 18). The addition of TBDMSCl afforded a better yield of compound 118.
5.5. Comparison with electrolysis
One-electron reduction using SmI2 was compared with electrolysis.29 When compound 120 was treated under CCE conditions (Zn-Pt electrode), compound 121 was produced in 39% yield (Scheme 8). However, SmI2 can give compound 121 in 80% yield as the sole product. In the case of compound 122, electrolysis gave the cyclized product 123 in 65% yield, which was better than SmI2 reduction (57%). Other interesting examples are described in this report.
In the case of compound 124, compound 126 predominated in electrolysis (Scheme 9).29 The yield of SmI2-mediated cyclization was 50% without an additive (125:126 = 11:89), and 100% with MeOH (20 eq) (125:126 = 55:45).
In the complicated example of compound 127, electrolysis afforded three products, 128, 129, and 130, in 31% yield (Scheme 10).29 However, with SmI2, the total yield was either 64 or 69%, depending on whether there was an additive or not (see Table 19). SmI2-mediated cyclization mainly afforded syn products involving the hydroxy group and hydrogen at the juncture position, while electrolysis gave anti products as the major products.
In the case of perhydronaphthalenes, SmI2-mediated cyclization of 46 gave syn products 47 and 48 as the major products (see Table 8),29 while electrolysis afforded anti product 49 as the major product (Scheme 11). The yield from electrolysis was less than from SmI2-mediated cyclization.
A compound bearing a ketone and an enone, 61, gave cyclization products, 62 and 63, by electrolysis in the ratio of 43:57 in total 100% yield. Although this is the coupling of an enone and a ketone, perhydronaphthalene was achieved in better yield by electrolysis (Scheme 12).29 These products both had syn configurations involving the hydroxy group and methyl group at the juncture position. In the case of SmI2, two more diastereoisomers were produced, although the ratio was not very high (see Table 11).
Substrate 39 was subjected to electrolysis (Scheme 13).29 The syn product 40 always predominated with both electrolysis and SmI2 (see Table 6).
Ketone 42 gave only 43 with electrolysis (Scheme 14).29 Cyclization mediated by SmI2 with or without MeOH gave 43 as a major product (see Table 7). For compounds 39 and 42, the transition state affording the syn product might be advantageous both in aqueous and THF solutions.
5.6. Application to the synthesis of coronafacic acid
The SmI2-mediated cyclization reaction was applied to the synthesis of coronafacic acid (132) (Scheme 15).30 Substrate 127 had one chiral center, and therefore the products were very complicated (Table 19). In most cases, three or four products were obtained depending on the conditions. However, the authors treated the whole mixture under isomerization conditions with alkaline, and thermodynamically stable products were separated. This method can be used for synthesis if one stable isomer predominates over the other.
5.7. Tricyclic systems
Hagiwara reported SmI2-induced cyclization of the bicyclic enone 135 (Scheme 16).31 The reaction without an additive gave a retro-aldol product. However, successful cyclization to 136 was accomplished with t-BuOH and HMPA at low temperature in 76% yield (exo:endo = 52:24). The compound without TBDMSO group 137 afforded 138 under similar conditions. This cyclization can be considered 5-Exo Trig cyclization. They proposed the ‘carbonyl-first’ mechanism, because the exo product predominated.
Two groups reported the cyclization to seven-membered carbocycles, making tricyclic compounds. Arimoto conducted SmI2-induced cyclization of compound 139 with t-BuOH as an additive and the desired tricyclic compound 140 was obtained in 86% yield as the sole isomer (Scheme 17).32 Interestingly, its diastereoisomer 141 did not afford a cyclized product, but only reduction products were isolated. This is a marked difference in conformation at the transition state of both compounds. Lee reported synthesis toward guanacastepene (Scheme 17).33 Compound 143 successfully afforded compound 144 in 70% yield as the sole product. Again, in this system the transition state highly predominated for cyclization, affording only one product.
5.8. 5,11-Fused ring systems
Five-membered carbocycles fused with the large ring can also be formed. A natural product 145 could be correlated with another natural product.34 Compound 145 was treated with SmI2 (with a large excess) without an additive, but no reaction occurred (Table 20). Water was added to the mixture and compound 146 was isolated as a major product with a small amount of 147 and 148. This tendency was almost the same as with HMPA. With DBU, only 146 was produced. The stereochemistry of the juncture in the major product was trans because of the strain of the large ring.
6. MECHANISTIC STUDIES
Cyclization between a ketone and an α,β-unsaturated system was next investigated (such as 149 and 150).35 This system resembles Matsuda and Shirahama's experiments (see Scheme 4), but lacks a hydroxy group. anti Hydroxy ester 152 was produced starting from E-149 by treating with SmI2 without an additive (Table 21). The same result was obtained on the addition of t-BuOH. However, in the presence of HMPA, both 151 and 152 were obtained. This phenomenon was completely reversed when Z-150 was used as the starting material. The major product was syn lactone 151 under most of the conditions, except with HMPA. The mechanism will be discussed later (vide infra).
One methylene in the side chain of α,β-unsaturated ester was elongated. When E-153 was treated with SmI2, anti lactone 156 was produced predominantly under most of the conditions employed (Table 22). Z-154 afforded syn lactone 155 in high yield. When 10 equivalents of t-BuOH were used for 153, compound 157 was produced (156:157 = 70:13) in 13% yield. The mechanism will be discussed later.
The mechanisms of SmI2-induced reactions have long been investigated by many scientists.1 Direct evidence to identify the radical using ESR has been sought and applications to nitroxyl radicals have been reported.36,37 Sono and Tori used a radical trapping method with DMPO and ESR.31 Compound 158 was treated with SmI2 in the presence of DMPO, and radical 159 was shown by ESR signals (Scheme 18). In this case, the only possible radical should be 159; therefore, the signals should be due to this radical 159, whose splitting pattern was reasonable. Therefore, this experiment indicates that the α,β-unsaturated carbonyl system can be reduced by SmI2 to afford a radical, 159.
Compound 149 was similarly treated with SmI2 in the presence of DMPO. The resulting ESR spectrum was derived from the sum of radicals 161 and 163 in the ratio of 100:12, demonstrated by simulation of the ESR spectra. Therefore, these results indicated that the α,β-unsaturated carbonyl system could be reduced faster than the isolated ketone. The mechanisms shown in Scheme 19 (‘alkene-first mechanism’) are possible, although they depend on the functionality in the molecule, because the difference between E and Z double bonds can also affect the results.
The finding that products from 149 and 150 were different could be explained by the conformation in the transition state, shown in Scheme 20. Samarium can coordinate both the carbonyl groups and hence the difference of the geometry of the double bond can change the shape of the transition state.
7. CONCLUSION
This review covers the cyclization reactions to bicyclic systems through either one- or two-electron reduction by SmI2. As demonstrated in the final section, samarium can pass one electron to the carbonyl group of the α,β-unsaturated system.38 This is presumably because the reduction potential of α,β−unsaturated carbonyl should be lower. In other words, it is reduced faster than the isolated ketone. Of course, this depends on the functionality and it is not always the fastest route. Procter described that the ‘alkene-first mechanism’ may also be considered, as well as the ‘carbonyl-first mechanism,’ which is widely accepted.1 Thus two-electron pathways sometimes work and cyclization due to this mechanism occurs frequently. SmI2 are easy to use and not toxic, but are sensitive to oxygen. As it can be coordinated with the carbonyl groups near the reaction site, the reaction using SmI2 can be controlled by the addition of various additives. The use of the SmI2 reagent will be further widened by developing synthetic methods in the future.
ACKNOWLEDGEMENTS
The authors express their sincere thanks to the students in their laboratory engaged in these projects and also to Dr. Katsuyuki Nakashima for his help. They are also grateful for the financial support from Tokushima Bunri University as well as a Grant-in-Aid for Scientific Research from JSPS.
References
1. D. J. Procter, R. A. Flowers, II, and T. Skrydstrup, 'Organic Synthesis Using Samarium Diiodide: A Practical Guide,' Royal Society of Chemistry Publishing, UK, 2010.
2. H. B. Kagan, L. J. Namy, and P. Girard, Tetrahedron, Suppl. 1, 1981, 37, 175.
3. L. J. Namy, J. Collon, C. Bied, and H. B. Kagan, Synlett, 1992, 733. CrossRef
4. H. B. Kagan, Tetrahedron, 2003, 59, 10351. CrossRef
5. P. Girard, L. J. Namy, and H. B. Kagan, J. Am. Chem. Soc., 1980, 102, 2693. CrossRef
6. D. P. Curran, T. L. Fevig, and M. J. Totleben, Synlett, 1990, 773. CrossRef
7. D. P. Curran, T. L. Fevig, C. P. Jasperse, and M. J. Totleben, Synlett, 1992, 943. CrossRef
8. D. P. Curran, X. Gu, W. Zhang, and P. Dowd, Tetrahedron, 1997, 53, 9023. CrossRef
9. G. A. Molander, Chem. Rev., 1992, 92, 29. CrossRef
10. G. A. Molander and C. R. Harris, Chem. Rev., 1996, 96, 307. CrossRef
11. G. A. Molander, Acc. Chem. Res., 1998, 31, 603. CrossRef
12. G. A. Molander and C. R. Haris, Tetrahedron, 1998, 54, 3321. CrossRef
13. R. D. Little, Chem. Rev., 1996, 96, 93. CrossRef
14. R. S. Miller, J. M. Sealy, M. Shabangi, M. L. Kuhlman, J. R. Fuchs, and R. A. Flowers, II, J. Am. Chem. Soc., 2000, 122, 7718. CrossRef
15. E. Prasad and R. A. Flowers, II, J. Am. Chem. Soc., 2002, 124, 6895. CrossRef
16. M. Szostak and D. J. Procter, Angew. Chem. Int. Ed., 2012, 51, 9238. CrossRef
17. M. Szostak, M. Spain, D. Parmer, and D. J. Procter, Chem. Comm., 2012, 48, 330. CrossRef
18. (a) T. Honda, Heterocycles, 2010, 81, 2719; CrossRef T. Honda, Heterocycles, 2011, 83, 1. CrossRef
19. M. Sono, Y. Nakashiba, K. Nakashima, and M. Tori, J. Org. Chem., 2000, 65, 3099. CrossRef
20. M. Sono, N. Ise, T. Shoji, and M. Tori, Molecules, 2012, 17, 11079. CrossRef
21. T. J. K. Findley, D. Sucunza, L. C. Miller, D. T. Davies, and D. J. Procter, Chem. Eur. J., 2008, 14, 6862. CrossRef
22. A. Kishida and H. Nagaoka, Tetrahedron Lett., 2008, 49, 6393. CrossRef
23. M. Sono, S. Onishi, and M. Tori, Tetrahedron, 2003, 59, 3385. CrossRef
24. M. Kito, T. Sakai, K. Yamada, F. Matsuda, and H. Shirahama, Synlett, 1993, 158. CrossRef
25. M. Sono, Y. Sugimoto, H. Tatara, N. Ise, S. Takaoka, and M. Tori, Tetrahedron, 2008, 64, 11096. CrossRef
26. D. S. Hsu and C. W. Hsu, Tetrahedron Lett., 2012, 53, 2185. CrossRef
27. M. Sono, Y. Nakashiba, K. Nakashima, S. Takaoka, and M. Tori, Heterocycles, 2001, 54, 101. CrossRef
28. M. Sono, T. Mizutani, M. Nozaki, S. Takaoka, and M. Tori, Heterocycles, 2008, 76, 851. CrossRef
29. M. Sono, T. Shoji, T. Tamaki, S. Kishi, and M. Tori, Heterocycles, 2007, 72, 517. CrossRef
30. M. Sono, A. Hashimoto, K. Nakashima, and M. Tori, Tetrahderon Lett., 2000, 41, 5115. CrossRef
31. H. Hagiwara, H. Sakai, T. Uchiyama, Y. Ito, N. Morita, T. Hoshi, T. Suzuki, and M. Ando, J. Chem. Soc., Perkin Trans. 1, 2002, 583. CrossRef
32. A. Sato, T. Masuda, H. Arimoto, and D. Uemura, Org. Biomol. Chem., 2005, 3, 2231. CrossRef
33. T. M. Nguyen, R. J. Seifert, D. R. Mowrey, and D. Lee, Org. Lett., 2002, 4, 3959. CrossRef
34. M. Sono, M. Hanaoka, T. Hashimoto, A. Asakawa, and M. Tori, Synlett, 2009, 469. CrossRef
35. M. Sono, S. Hanamura, M. Furumaki, H. Murai, and M. Tori, Org. Lett., 2011, 13, 5720. CrossRef
36. A. R. Katritzky, H. Y. He, and G. Qiu, Org. Lett., 1999, 1, 1755. CrossRef
37. A. Alberti, M. Benaglia, and D. Macciantelli, Org. Lett., 2000, 2, 1553. CrossRef
38. J. H. Wagenknecht, L. Eberson, and J. H. P. Utley, 'Organic Electrochemistry,' Third Edition, ed. by H. Lund and M. M. Baizer, Marcel Dekker, Inc., New York, 1991, 453.