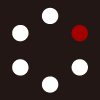
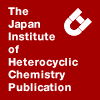
HETEROCYCLES
An International Journal for Reviews and Communications in Heterocyclic ChemistryWeb Edition ISSN: 1881-0942
Published online by The Japan Institute of Heterocyclic Chemistry
e-Journal
Full Text HTML
Received, 7th August, 2014, Accepted, 14th October, 2014, Published online, 29th October, 2014.
DOI: 10.3987/COM-14-S(K)104
■ Efficient Desulfinative Cross-Coupling of Heteroaromatic Sulfinates with Aryl Triflate in Environmentally Friendly Protic Solvents
Daniel Mangel, Cindy Buonomano, Stéphane Sévigny, Gianna Di Censo, Gowsic Thevendran, and Pat Forgione*
Department of Chemistry and Biochemistry, Concordia University, 7141 Sherbrooke Street West, SP 201.00, Montreal, Quebec, H4B 1R6, Canada
Abstract
Aryl-substituted heteroaromatics were synthesized via desulfinative cross-coupling reactions using aryl triflate and heteroaromatic sulfinate coupling partners. This method uses synthetically versatile aryl triflates to access aryl-substituted heteroaromatics in good yields employing aqueous and alcoholic media without the use of base, additives or co-catalysts.INTRODUCTION
Aryl-substituted heteroaromatics are a key motif in a variety of applications including medicinal chemistry,1 natural products,2 advanced materials,3 and the agrochemical industry.4 As a consequence of this versatility, these structures have attracted much attention of the scientific community in developing novel, efficient, methods for accessing these scaffolds. One method towards these structures is via desulfinative cross-couplings. This relatively new cross-coupling was shown to be an attractive alternative to the popular decarboxylative cross-couplings5 and direct arylations6 due to its more facile gas extrusion, chemoselectivity, and environmentally benign nature. Sulfinates can be readily synthesized from recycling the large amounts of SO2 waste, that is generated industrially.7 The facile extrusion of SO2 does not require a co-catalyst or extensive reaction times thus making the desulfinative cross-coupling8a-c of aryl sulfinates8d an interesting alternative to classical methods. There are a few methods involving aryl sulfinates as nucleophilic coupling partners for palladium cross-coupling reactions.9 Billard’s group demonstrated desulfinative cross-couplings of haloquinolines with aryl-sufinates9a and Duan’s group developed the desulfinative coupling of sodium aryl sulfinates with aryl bromides and chlorides (Scheme 1, eq. 1).9b Recently, we reported a cross-coupling protocol for the synthesis of aryl-substituted heteroaromatics utilizing heteroaromatic sulfinate salts and aryl bromides.10 The cross-coupling was shown to proceed in good yields without the need of additives or base in H2O/DMF (3:1) with PdCl2 and PPh3 (Scheme 1, eq. 2). Recently, Duan has developed conditions for the arylation of aryl sulfinates using aryl triflates in good yields (Scheme 1, eq. 3).11 Similarly, many other groups have shown the successful use of aryl triflates in arylation processes,12 however, there are only limited examples in aqueous solvents.13 Replacing organic solvents by aqueous solutions is an asset for economic and environmental reasons. Nevertheless, most organic compounds and especially organometallic catalysts are not soluble in water. To further diversify our method, aryl triflates have been applied as the electrophilic desulfinative cross-coupling partner, an alternative to the aryl halide counterpart (Scheme 1, eq. 4). These aryl triflates are attractive coupling partners due to their availability, low cost, and high yielding production. The phenol group is very common in organic synthesis and can be used to insert a new functional group on the aromatic ring as well as be converted into a new carbon-carbon bond. Due to the high abundance of the phenol functionality and the ease for its transformation into an aryl triflate, the versatility of the desulfinative palladium-catalyzed cross-coupling is increased tremendously.
RESULTS AND DISCUSSION
Optimization of the desulfinative cross-coupling reaction began with the screening of different catalytic systems. The palladium source was first screened under the optimal conditions previously reported, H2O:DMF (3:1) at 170 °C for 8 min under microwave irradiation.10a Lithium thiophene-2-sulfinate (1a) and 4-cyanophenyl triflate (2b) were chosen as coupling partners for the optimization of the reaction conditions (Table 1).
The highly reactive Pd[P(tBu)3]2 only yielded a trace amount of the expected product (Table 1, entry 1). Promisingly, the generation of this catalyst in situ employing PdCl2 and HP(tBu)3BF4 (entry 2) showed improved yield of the desired product that led us to screen a range of phosphine ligands (entry 3-14). The optimal yield was obtained using the inexpensive and readily available PPh3 as the ligand (entry 14).
Having determined the optimal ligand, a range of Pd sources were also evaluated. Although slight variations in yield were observed (entries 14-19), Pd(acac)2 and Pd(TFA)2 were found to be the optimal palladium sources when used in conjunction with PPh3. Further optimization would be carried out using the less expensive Pd(acac)2.
Although the H2O/DMF yielded the desired product in good yield (Table 2, entry 1), the presence of significant amounts of DMF makes the water more difficult to purify and consequently recycle.14 Unfortunately, the coupling in pure water occurs in moderate yield (entry 2), which indicates that DMF may play a role in solubilizing all reagents. The use of alcoholic solvents (entries 3-4) is attractive, because they are biodegradable15 and readily available from biomass.16 There are a variety of applications of alcoholic solvents in synthetic organic chemistry,17 many involving cross-coupling reactions.18 Despite their potential attractiveness as a solvent, no significant work has been carried out on decarboxylative or desulfinative palladium-catalyzed cross-coupling reactions. In order to develop the desulfinative cross-coupling employing a green solvent system, that may improve the solubility of reagents when compared to pure water, protic solvents EtOH and iPrOH were selected for evaluation.15 The preliminary results employing EtOH (entry 3) and iPrOH (entry 4), although lower than those obtained in the H2O/DMF solvent mixture, provided encouraging yields and the benefit of being single solvent systems that would be easier to subsequently purify. As such, further optimizations were carried out with both these solvents.
Employing Pd(acac)2 in conjunction with PPh3 in EtOH or iPrOH, the temperature, time, and heating method were optimized. Although increasing reaction times to 170 ºC in the microwave did not prove beneficial (Table 3, entries 1-4), when the reaction temperature was reduced to 150 ºC (entries 5-7), an important improvement in cross-coupling yield to 89% was observed (entry 6). Further reducing the reaction temperature to 120 ºC while increasing the reaction time to 4 h did not prove beneficial when performed in the microwave (entry 8), however when performed thermally and extending the reaction time
to 19 h, an excellent yield of 89% was obtained (entry 9). Other attempts at thermal conditions (entries 10-11) using Pd(TFA)2 did not show any increased yield. Having determined the optimal heating conditions in EtOH using the microwave at 150 ºC for 1 h, and using H2O/DMF (3:1) at 170 °C for 8 min, these two conditions were applied in a study of heteroaromatic sulfinate substrate scope.
While employing the H2O/DMF solvent system, good to excellent yields were obtained (Table 4), however, in all cases, equal or better yields were obtained when using ethanol as the solvent. As demonstrated in the table, benzo[b]thiophene-2-sulfinate (1b, entry 2) and benzo[b]furan-2-sulfinate (1g, entry 7) provided the corresponding product in the highest yield. Interestingly, the unsubstituted sulfinate (1a, entry 1) led to a reduced yield. Methyl substituted thiophenes (1c-e, entries 3-5) provided the corresponding cross-coupling product in good yields in EtOH, however, 2-methylthiophene (entry 5) provided much better yields in EtOH in comparison with the H2O/DMF mixture, highlighting the significant advantage of EtOH in certain cases. Furan-2-sulfinate (1f, entry 6) also provided the corresponding product in very good yields, but the pyridine-2-sulfinate gave only moderate yield (1g, entry 8). Although EtOH generated the cross-coupling products with consistently higher yields, using the H2O/DMF system also proved to be efficient, highlighting the complementary nature of these solvents systems. Other aryl triflate coupling partners bearing electron donating groups were investigated to emphasize the versatility of this methodology. Electrophilic coupling partners with electron donating groups have been known to undergo palladium-mediated cross-couplings less efficiently.10 This was also observed under our modified conditions, especially when considering the most challenging 4-methoxyphenyl triflate (2d, entries 9 and 10). However, thiophene-2-sulfinate (1a) underwent cross-coupling with 3-methoxyphenyl triflate (2c, entry 11) in a very good yield of the corresponding product. In both cases, the yields observed were moderate to very good, further demonstrating that the method can be efficient with both electron donating groups and electron withdrawing groups on the aromatic ring of the triflate coupling partner.
To summarize, the reported conditions generate industrially interesting aryl-susbsituted heteroaromatic products using versatile aryl triflates as a coupling partners in alcoholic and aqueous media. The use of biodegradable EtOH as the solvent proved to be efficient by providing a range of heteroaromatic bi-aryls in moderate to excellent yields. This green solvent system provided improved results overall than the H2O/DMF mixture. This process provides an economical and green alternative to other available methods using aryl triflates for palladium catalyzed cross-coupling reactions of heteroaryl sulfinates with aryl triflates and has potential for further development.
EXPERIMENTAL
All anhydrous flasks were flame-dried while under high-vacuum and purged with argon unless otherwise stated. Solids were weighed on a balance open to air and added to a round bottom flask or microwave vial unless otherwise noted. Liquids were transferred using a glass syringe with a stainless steel needle or a micropipette for µL volumes unless noted otherwise. Manual flash chromatography columns were carried out using 40-63 µm silica gel from Silicycle. All reagents purchased are from Sigma-Aldrich or Alfa Aesar and used without further purification unless otherwise noted. All solvents were purchased as ACS grade from Fischer Scientific or JT Baker unless otherwise noted. Anhydrous solvents were dried and stored in a flame-dried Schlenk flask using 3 Å molecular sieves, which were activated by heating at 150 ºC under high vacuum overnight. Distilled water was obtained from an in-house distillery. Unless otherwise noted, reactions were performed using a Biotage Initiator 2.3 build 6250 microwave. Purifications by flash column chromatography were performed using a Teledyne Isco CombiFlash® Rf unless mentioned otherwise. Proton nuclear magnetic resonance spectra (1H NMR) were measured at 500 MHz using a Varian VNMRS-500 in CDCl3 unless stated otherwise. Carbon nuclear magnetic resonance spectra (13C NMR) were measured at 125 MHz using the Varian VNMRS-500 in CDCl3 unless stated otherwise. The chemical shifts are reported in parts per million (ppm) and referenced from either residual solvent or the tetramethylsilane (TMS) signal. The multiplicity is represented as; s = singlet, d = doublet, t = triplet, q = quartet and m = multiplet which is indicated in parentheses along with the number of protons and coupling constants (in Hz). Gas chromatograph-mass spectral analyses (GC-MS) were obtained using an Agilent 7890A GC system and Agilent 5975C VL MSD with Triple-Axis MS Detector with a HP-588 column coated with (5%-phenyl)-methylpolysiloxane.
General procedure for the synthesis of heteroaromatic lithium sulfinates (A).
To a dried, rubber septum capped flask, under an argon stream, equipped with a magnetic stir bar and cooled to -78 ºC was added the heteroaromatic (1.0 equiv.) with anhydrous Et2O (0.3 M). After 20 min, with stirring, tert-butyllithium (0.9 equiv.) was added slowly with a glass syringe over 5 min. The reaction was stirred for 2 h while maintaining a temperature of -78 ºC. The reaction was then quenched by bubbling SO2 produced from general procedure (B) for the generation of anhydrous sulfur dioxide for 1 h, while warming to 23 ºC, precipitating the sulfinate salt. The salt was isolated via vacuum filtration, washed thoroughly with Et2O followed by acetone, and dried under vacuum. The solid was then ground to a fine powder, to which Et2O was added, and sonicated for 10 min, followed by vacuum filtration and drying under high vacuum. Heteroaromatic lithium sulfinates 1a-1g10 and 1h22 correspond to what was reported previously in the literature.
General procedure for the generation of anhydrous sulfur dioxide (B).
To a three-neck flask equipped with a magnetic stir bar, sodium sulfite or sodium metabisulfite (1.0 equiv.) and water were added. Concentrated sulfuric acid (1.0 equiv.) was added drop-wise, with stirring, from a capped pressure-equalized addition funnel. The gas generated was then scrubbed twice via diffusion through concentrated sulfuric acid.
General procedure for the arylation of heteroaromatic lithium sulfinates with aryl triflate (C).
To a 5 mL conical microwave vial equipped with a spin-vein was added heteroaromatic sulfinate (0.30 mmol, 1.5 equiv.), aryl triflate (0.20 mmol, 1.0 equiv.), Pd(acac)2 (0.01 mmol, 0.05 equiv.) and PPh3 (0.05 mmol, 0.25 equiv.). 2 mL of either EtOH or 3:1 H2O:DMF were added and the vial was pre-stirred for 30 sec at 23 ºC followed by appropriate heating (see Table 4). The crude cross-coupling solution was diluted with EtOAc (5 mL). The organic layer was washed with a saturated NaCl aqueous solution (2x 5 mL), saturated NaHCO3 aqueous solution (2x 5 mL), distilled H2O (1x 5 mL), and saturated NaCl aqueous solution (1x 5 mL). The combined aqueous phases were washed with EtOAc (3x 5 mL). The combined organic phases were dried over Na2SO4 and after filtration the solvent evaporated under reduced pressure and the solid residue was purified by flash column chromatography.
4-(Thiophen-2-yl)benzonitrile (3a). The above compound was prepared from general procedure (C) on a 0.20 mmol (37.05 mg) scale. 1H NMR (500 MHz, CDCl3) δ 7.69 (d, J = 8.4 Hz, 2H), 7.65 (d, J = 8.4 Hz, 2H), 7.42 (dd, J = 3.5, 0.9 Hz, 1H), 7.40 (dd, J = 5.1, 1.0 Hz, 1H), 7.13 (dd, J = 5.0, 3.7 Hz, 1H) ppm. 13C NMR (126 MHz, CDCl3) δ 142.2, 138.8, 132.9 (2C), 128.7, 127.2, 126.2 (2C), 125.2, 119.0, 110.7 ppm.
4-(Benzo[b]thiophen-2-yl)benzonitrile (3b). The above compound was prepared from general procedure (C) on a 0.20 mmol (47.06 mg) scale. 1H NMR (500 MHz, CDCl3) δ 7.84 (dd, J = 6.4, 2.1 Hz, 1H), 7.81 (dd, J = 6.6, 2.4 Hz, 1H), 7.77 (d, J = 8.2 Hz, 2H), 7.64 (s, 1H), 7.42 – 7.35 (m, 2H) ppm. 13C NMR (126 MHz, CDCl3) δ 141.8, 140.4, 140.1, 138.7, 132.8 (2C), 126.8 (2C), 125.5, 125.1, 124.3, 122.5, 121.9, 118.7, 111.5 ppm.
4-(5-Methylthiophen-2-yl)benzonitrile (3c). The above compound was prepared from general procedure (C) on a 0.20 mmol (39.85 mg) scale. 1H NMR (300 MHz, CDCl3) δ 7.61 (s, 4H), 7.22 (d, J = 3.6 Hz, 1H), 6.78 (dd, J = 3.6, 1.1 Hz, 1H), 2.53 (d, J = 0.8 Hz, 3H) ppm. 13C NMR (126 MHz, CDCl3) δ 142.3, 139.7, 139.0, 132.8 (2C), 126.9, 125.6 (2C), 125.2, 119.1, 110.0, 15.7 ppm.
4-(4-Methylthiophen-2-yl)benzonitrile (3d). The above compound was prepared from general procedure (C) on a 0.20 mmol (39.85 mg) scale. 1H NMR (300 MHz, CDCl3) δ 7.64 (d, J = 1.8 Hz, 4H), 7.23 (d, J = 1.1 Hz, 1H), 6.99 – 6.96 (m, 1H), 2.30 (d, J = 0.8 Hz, 3H) ppm. 13C NMR (126 MHz, CDCl3) δ 141.8, 139.3, 138.9, 132.8 (2C), 127.5, 125.9 (2C), 122.7, 119.0, 110.5, 15.9 ppm.
4-(3-Methylthiophen-2-yl)benzonitrile (3e). The above compound was prepared from general procedure (C) on a 0.20 mmol (39.85 mg) scale. 1H NMR (500 MHz, CDCl3) δ 7.70 – 7.67 (m, 2H), 7.59 – 7.55 (m, 2H), 7.30 (d, J = 5.1 Hz, 1H), 6.96 (d, J = 5.1 Hz, 1H), 2.36 (s, 3H) ppm. 13C NMR (126 MHz, CDCl3) δ 139.6, 135.8, 135.1, 132.4 (2C), 131.8, 129.3 (2C), 125.3, 119.0, 110.6, 15.3 ppm.
4-(Furan-2-yl)benzonitrile (3f). The above compound was prepared from general procedure (C) on a 0.20 mmol (33.64 mg) scale. 1H NMR (300 MHz, CDCl3) δ 7.74 (d, J = 8.7 Hz, 2H), 7.65 (d, J = 8.8 Hz, 2H), 7.54 (dd, J = 1.8, 0.6 Hz, 1H), 6.81 (dd, J = 3.4, 0.6 Hz, 1H), 6.53 (dd, J = 3.5, 1.8 Hz, 1H) ppm. 13C NMR (126 MHz, CDCl3) δ 152.1, 143.8, 134.78, 132.72 (2C), 124.0 (2C), 119.1, 112.4, 110.4, 108.3 ppm.
4-(Benzo[b]furan-2-yl)benzonitrile (3g). The above compound was prepared from general procedure (C) on a 0.20 mmol (43.85 mg) scale. 1H NMR (500 MHz, CDCl3) δ 7.95 – 7.92 (m, 2H), 7.73 – 7.70 (m, 2H), 7.63 (ddd, J = 7.7, 1.2, 0.6 Hz, 1H), 7.54 (dd, J = 8.3, 0.8 Hz, 1H), 7.36 (ddd, J = 8.4, 7.3, 1.3 Hz, 1H), 7.30 – 7.26 (m, 1H) ppm. 13C NMR (126 MHz, CDCl3) δ 155.4, 153.7, 134.6, 132.7 (2C), 128.8, 125.7, 125.2 (2C), 123.6, 121.6, 118.9, 111.65, 111.56, 104.5 ppm.
4-(Pyridin-2-yl)benzonitrile (3h). The above compound was synthesized following general procedure (C) on a 0.20 mmol (45 mg) scale. 1H NMR (500 MHz, CDCl3) δ 7.57 – 7.54 (m, 4H), 6.93 – 6.89 (m, 4H) ppm. 13C NMR (126 MHz, CDCl3) δ 159.7, 152.0, 134.3 (2C), 122.6, 119.2, 117.1, 116.3 (2C), 112.8, 103.7, 29.7 ppm.
2-(4-Methoxyphenyl)thiophene (3i). The above compound was synthesized following general procedure (C) on a 0.20 mmol (46 mg) scale ale. 1H NMR (500 MHz, CDCl3) δ 7.55 – 7.54 (m, 1H), 7.54 – 7.52 (m, 1H), 7.20 (ddd, J = 4.7, 4.3, 1.2 Hz, 2H), 7.05 (dd, J = 5.1, 3.6 Hz, 1H), 6.95 – 6.89 (m, 2H), 3.84 (s, J = 2.8 Hz, 3H) ppm. 13C NMR (126 MHz, CDCl3) δ 159.2, 143.2, 128.1, 127.4, 127.4, 124.0, 122.49, 122.21, 115.2, 114.4, 55.9 ppm.
2-(4-Methoxyphenyl)benzo[b]thiophene (3j). The above compound was synthesized following general procedure (C) on a 0.20 mmol (61 mg) scale. 1H NMR (500 MHz, CDCl3) δ 7.82 – 7.79 (m, 1H), 7.74 (dd, J = 7.7, 1.1 Hz, 1H), 7.66 – 7.63 (m, 2H), 7.43 (d, J = 0.5 Hz, 1H), 7.35 – 7.32 (m, 2H), 7.30 – 7.28 (m, 1H), 6.98 – 6.94 (m, 2H), 3.86 (s, 3H) ppm. 13C NMR (126 MHz, CDCl3) δ 127.8 (2C), 124.93, 124.80, 124.44, 123.94, 123.73, 123.25, 122.2, 121.4, 118.2, 114.4 (2C), 55.4 ppm.
2-(3-Methoxyphenyl)thiophene (3k). The above compound was synthesized following general procedure (C) on a 0.20 mmol (46 mg) scale. 1H NMR (500 MHz, CDCl3) δ 7.31 (dd, J = 3.6, 1.2 Hz, 1H), 7.29 (s, 1H), 7.28 – 7.27 (m, 1H), 7.21 (ddd, J = 7.7, 1.7, 1.0 Hz, 1H), 7.15 – 7.14 (m, 1H), 7.08 (dd, J = 5.1, 3.6 Hz, 1H), 6.84 (ddd, J = 8.2, 2.5, 0.9 Hz, 1H), 3.86 (s, 3H) ppm. 13C NMR (126 MHz, CDCl3) δ 160.1, 144.4, 135.9, 130.0, 128.1, 125.0, 123.4, 118.7, 113.1, 111.8, 55.4 ppm.
2-(3-Methoxyphenyl)benzo[b]thiophene (3l). The above compound was synthesized following general procedure (C) on a 0.20 mmol (61 mg) scale. 1H NMR (500 MHz, CDCl3) δ 7.84 – 7.81 (m, 1H), 7.79 – 7.76 (m, 1H), 7.54 (d, J = 0.6 Hz, 1H), 7.37 – 7.29 (m, 4H), 7.26 – 7.24 (m, 1H), 3.89 (d, J = 1.9 Hz, 3H) ppm. 13C NMR (126 MHz, CDCl3) δ 160.01 144.2, 140.7, 139.6, 135.8, 130.10 124.6, 124.5, 123.7, 122.4, 119.8, 119.2, 113.9, 112.3, 55.5 ppm.
ACKNOWLEDGEMENTS
This work was funded by the Natural Sciences and Engineering Research Council of Canada (NSERC) and is dedicated to Professor Isao Kuwajima for his contributions to organic chemistry. Special thanks to Dr. Simon Woo for his help and guidance.
References
1. (a) E. Bey, S. Marchais-Oberwinkler, M. Negri, P. Kruchten, A. Oster, T. Klein, A. Spadaro, R. Werth, M. Frotscher, B. Birk, and R. W. Hartmann, J. Med. Chem., 2009, 52, 6724; CrossRef (b) N. W. Y. Wong, and P. Forgione, Org. Lett., 2012, 14, 2738. CrossRef
2. S. Nakamura, K. Mitsuyoshi, K. Goto, M. Nakamura, Y. Tsuda, and K. Shishido, Hetereocycles, 1996, 43, 2747. CrossRef
3. (a) J. Ohshita, S. Kangai, Y. Tada, H. Yoshida, K. Sakamaki, A. Kunai, and Y. Kunugi, J. Organomet. Chem., 2007, 692, 1020; CrossRef (b) J. Ohshita, S. Kangai, H. Yoshida, A. Kunai, S. Kajiwara, Y. Ooyama, and Y. Harima, J. Organomet. Chem., 2007, 692, 801; CrossRef (c) T. Beryozkina, V. Senkovskyy, E. Kaul, and A. Kiriy, Macromolecules, 2008, 41, 7817; CrossRef (d) D. J. Schipper and K. Fagnou, Chem. Mater., 2011, 23, 1594; CrossRef (e) J. H. Seo, E. B. Namdas, A. Gutacker, A. J. Heeger, and Bazan, Adv. Funct. Mater., 2011, 21, 3667; CrossRef (f) F. Zhang, D. Wu, Y. Xua, and X. Feng, J. Mater. Chem., 2011, 21, 17950; (g) K. Beydoun, J. Boixel, V. Guerchais, and H. Doucet, Catal. Sci. Technol., 2012, 2, 1242; CrossRef (h) R. J. Ono, S. Kang, and C. W. Bielawski, Macromolecules, 2012, 45, 2321. CrossRef
4. M. E. Matheron and M. Porchas, Plant. Dis., 2004, 88, 665. CrossRef
5. (a) L. J. Gooßen, G. Deng, and L. M. Levy, Science, 2006, 313, 662; CrossRef (b) J.-M. Becht and C. L. Drian, Org. Lett., 2008, 10, 3161; CrossRef (c) F. Bilodeau, M.-C. Brochu, N. Guimond, K. H. Thesen, and P. Forgione, J. Org. Chem., 2009, 75, 1550. CrossRef
6. (a) D. Alberico, M. E. Scott, and M. Lautens, Chem. Rev., 2007, 107, 174; CrossRef (b) Y. Li, J. Wang, M. Huang, Z. Wang, Y. Wu, and Y. Wu, J. Org. Chem., 2014, 79, 2890. CrossRef
7. S. J. Smith, J. V. Aardenne, Z. Klimont, R. J. Andres, A. Volke, and S. D. Arias, Atmos. Chem. Phys., 2011, 11, 1101. CrossRef
8. (a) K. Garves, J. Org. Chem., 1970, 35, 3273; CrossRef (b) G. Vitzthum and E. Lindnerl, Angew. Chem., Int. Ed. Engl., 1971, 10, 315; CrossRef (c) V. R. Selke and W. Thiele, J. Prakt. Chem., 1971, 313, 875; CrossRef (d) K. Sato and T. Okoshi, Patent US5159082, 1992.
9. (a) J. Colomb and T. Billard, Tetrahedron Lett., 2013, 54, 1471; CrossRef (b) C. Zhou, Y. Li, Y. Lu, R. Zhang, K. Jin, X. Fu, and C. Duan, Chin. J. Chem., 2013, 31, 1269; CrossRef (c) A. Barthelme, S. Aly, B. Desharnais, S. Rioux, and P. Forgione, Synthesis, 2013, 45, 694. CrossRef
10. (a) S. Sévigny and P. Forgione, New J. Chem., 2013, 37, 589; CrossRef (b) S. Sévigny and P. Forgione, Chem. Eur. J., 2013, 19, 2256. CrossRef
11. C. Zhou, Q. Liu, Y. Li, R. Zhang, X. Fu, and C. Duan, J. Org. Chem., 2012, 77, 10468. CrossRef
12. (a) G. W. Kabalka, L.-L. Zhou, and A. Naravane, Tetrahedron Lett., 2006, 47, 6887; CrossRef (b) J. Roger and H. Doucet, Org. Biomol. Chem., 2007, 6, 169. CrossRef
13. (a) C. G. Blettner, W. A. König, W. Stenzel, and T. Schotten, J. Org. Chem., 1999, 64, 3885; CrossRef (b) C. Rabeyrin and D. Sinou, J. Mol. Catal. A: Chem., 2004, 215, 89. CrossRef
14. D. A. Devi, B. Smitha, S. Sridhar, and T. M. Aminabhavi, Sep. Purif. Technol., 2006, 51, 104. CrossRef
15. C. Capello, U. Fischer, and K. Hungerbühler, Green Chem., 2007, 9, 927. CrossRef
16. (a) T. Chmielniak and M. Sciazko, Applied Energy, 2003, 74, 393; CrossRef (b) D. Pimentel and T. W. Patzek, Nat. Resour. Res., 2005, 14, 65. CrossRef
17. (a) D. S. Bose, L. Fatima, and H. B. Mereyala, J. Org. Chem., 2003, 68, 587; CrossRef (b) A. Laitinen, Y. Takebayashi, I. Kylänlahti, J. Yli-Kauhaluoma, T. Sugetab, and K. Otakeb, Green Chem., 2004, 6, 49. CrossRef
18. (a) G. W. Kabalka, E. Dadusha, and M. Al-Masum, Tetrahedron Lett., 2006, 47, 7459; CrossRef (b) J.-W. Wang, F.-H. Meng, and L.-F. Zhang, Organometallics, 2009, 28, 2334; CrossRef (c) W. Ren, J. Li, D. Zou, Y. Wu, and Y. Wu, Tetrahedron, 2012, 68, 1351; CrossRef (d) X. Le, Z. Dong, Z. Jin, Q. Wang, and J. Ma, Catal. Commun., 2014, 53, 47. CrossRef
19. J. R. Vyvyan, J. A. Dell Nee Meyer, T. J. Ligon, K. K. Motanic, and H. S. Wall, Synthesis, 2010, 3637. CrossRef
20. K. J. Hoffmann and P. H. J. Carlsen, Synth. Commun., 1999, 29, 1607. CrossRef
21. D. L. Comins and A. Dehghani, Tetrahedron Lett., 1992, 33, 6299. CrossRef
22. C. Martin, F. Sandrinelli, C. Perrio, S. Perrio, and M.-C. Lasne, J. Org. Chem., 2006, 71, 210. CrossRef