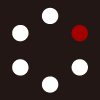
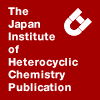
HETEROCYCLES
An International Journal for Reviews and Communications in Heterocyclic ChemistryWeb Edition ISSN: 1881-0942
Published online by The Japan Institute of Heterocyclic Chemistry
e-Journal
Full Text HTML
Received, 18th June, 2014, Accepted, 10th July, 2014, Published online, 24th July, 2014.
DOI: 10.3987/REV-14-800
■ Pyroglutamic Acid Derivatives: Building Blocks for Drug Discovery
Azzurra Stefanucci, Ettore Novellino, Roberto Costante, and Adriano Mollica*
Dipartimento di Farmacia, Università di Chieti-Pescara “G. d’Annunzio”, Via dei Vestini 31, 66100, Chieti, Italy
Abstract
Substituted prolines namely amino acid proline chimeras, have received a great synthetic efforts with the aim to develop a full library of compounds to be used in bioactive peptides drug discovery. Conversely pyroglutamic acid derivatives, strictly related to proline chimeras have been used only marginally in peptide modifications, probably due the lack of handled asymmetric syntheses. In this work we reviewed the “state of the art” on the synthetic approaches to pyroglutamic acid derivatives bearing a side chain related to that of natural amino acids.INTRODUCTION
Proline (1) is the only natural amino acid with a cyclic structure and a secondary amine. Its peculiar structure gave rise to a series of studies with the aim to understand its importance in the protein folding and structural ligand-receptor recognition. In the past few decades a series of substituted prolines, namely proline-chimeras, have been synthesized as useful tools in drug discovery (Figure 1).
Proline chimeras (2-6) are usually mono-substituted prolines bearing on the pyrrolidine ring a substituent, deeply related to the side chain of the natural occurring amino acids (Figure 1). Once inserted, in place of the native amino acid, into a bio-active peptide, a proline chimera can modify the folding of the peptide, still maintaining the natural side chain important for recognition. It has been demonstrated that a proline residue can induce a β-turn folding in the peptide backbone which is an important secondary structure often presents in bioactive peptides.1 Another important features of proline-chimeras is that the substituent on the pyrrolidine group is a mimetic of the side chain of other amino acids, but the rotation on the χ1 and χ2 angles results heavily restricted.1 This restriction in the conformational freedom could deeply change the bio-activity of the peptide, ranging from super-potent agonists to antagonists or other relevant changes in bio-activity. Pyroglutamic acid (8) is structurally linked to proline and substituted pyroglutamic acids can be easily inserted into a bio-active peptides backbone, similarly to proline-chimeras, in order to modify their secondary structure and bias the interaction with the biological target (Figure 2).1
The use of substituted pyroglutamic acids as surrogate of natural occurring amino acids is less explored. In a recent paper Tsai et al. 1f reported the use of substituted pyroglutamate derivatives in the design and SAR studies of Fibloblast activation protein (FAP) inhibitors. FAP is a serine protease that cleaves bioactive peptides preferentially after proline residues being expressed in near the 90% of epithelial cancers1g it has been suggested that FAP promotes tumor genesis, and its inhibition may attenuate the tumor growth. The design of novel petidomimetics, introduces a constraint in the P2 portion of lead IG-409, the conversion of the P2 side secondary amine to amide and a series of modification at the pyrrolidine C terminal, as show in Figure 3. Modifications on the amine substituents in position 4 and at the carbonylmethyl group lead to the discover of potent pyroglutamic acid-based FAP inhibitors with high selectivity for FAP over DPP-IV, DPP-II, DPP8 and DPP9.
Pyroglutamic acid (8) has been also widely used as chiral synthon for the preparation of a series of chiral bio-active compounds, as chiral auxiliary in asymmetric synthesis,2 and as precursor for other amino acids,3 such as α-amino acids and conformationally constrained glutamic acid, alanine, lysine and ornithine derivatives.4,5 A recent review by Panday et al.4 reported a series of synthesis where pyroglutamic (8) acid was used as chiral synthon. Some derivatives have shown biological activity, as inhibitors of the vascular cell adhesion molecule-1 (VCAM-1) and integrin interactions, which makes them particularly useful for treating rheumatoid arthritis and asthma.6
Both S and R-pyroglutamic acids are commercially available in optically pure form: S-pyroglutamic derivatives have been used to resolve racemic mixtures by forming diastereomeric species,7 or as substrate of enantioselective enzymatic reactions.8
Parrish et al.9 reported a series of pyroglutamic acid derivatives, easily prepared from the pyroglutamic diketopiperazines. Pyroglutamic containing DKPs undergo to a ring opening by reaction with diamines, in such way substituted pyroglutamic acids have also been synthesized.10
Numerous approaches to symmetric and asymmetric functionalized pyroglutamic acid derivatives have been reported, although each presents some limitations.11,12 One common method to obtain these functionalized pyroglutamates is the alkylation of the lithium enolate derived from N-protected pyroglutamic esters to give C4 substituted derivatives.13-17 This method works well for reactive electrophiles such as allylic halides and aryl aldehydes, with moderate yields and stereospecificity. Ohta et al.18 reported a hydroxylation of the lithium enolate derived from benzyl N-Boc-(L)-pyroglutamate with 3-phenyl-2-toluenesulfonyl-1,2-oxaziridine to give trans-4-hydroxypyroglutamate.19 Recently, Merino et al. reported an asymmetric synthesis of 4-hydroxypyroglutamic acid, involving a dipolar-cycloaddition of a nitrone with acrylamide derived from Oppolzer’s sultam.20
Functionalized pyroglutamic acid derivatives have been also prepared using the Michael reaction of amide-tethered di-acids with alkynones.21 One practical synthetic pathway is the homologation of nucleophilic glycine-equivalents for the synthesis of sterically constrained 3-substituted pyroglutamic acids, thus increasing the synthetic efficiency of the Michael addition reactions.22
In this review, various methods both asymmetric and symmetric, for the synthesis of pyroglutamic amino acid-chimeras are described (Figure 4).
Each paragraph has been named on the basis of the position on the pyrrolidine ring reporting side chain fragments of proteinogenic amino acids when present. Taking into account the presence of a review on pyroglutamic acid derivatives,23 we have focused our attention on the chemical strategies contributions of the literature from 2000 to 2014.
2. SYNTHESES OF PYROGLUTAMIC ACID DERIVATIVES
2.1 α-SUBSTITUTED PYROGLUTAMIC ACID DERIVATIVES
2.1.1 ANODIC AMIDE OXIDATION REACTION
Simpson et al.24 realized the synthesis of a series of TRH analogues. Initially, the strategy utilized allowed to reach the synthesis in six steps from pyroglutamic acid. A revised strategy for synthesizing the spirocyclic lactam ring skeletons was then proposed (Scheme 1).
The key step was the conversion of the 5-allylpyroglutamate derivative 11 into an advanced intermediate that could be used to build the desired analogue 12. This would be accomplished by using the olefin of the allyl group to set up either an intramolecular reductive amination reaction or an intramolecular Mitsunobu reaction. Thus, both the intermolecular reductive amination and the diketopiperazide formation would be avoided. The allyl-substituted pyroglutamate intermediate 11 was made by first functionalizing menthyl pyroglutamate 9 with the use of an anodic amide oxidation reaction,25,26 and then treating the resulting product 10 with allylsilane and TiCl4 to afford a mixture of stereoisomers allylpyroglutamate derivatives. Crystallization led to 11 in a 54% isolated yield.
2.1.2 UGI REACTION
For the synthesis of quaternary (α-substituted) pyroglutamic acid, the Ugi multicomponent reaction (U4C-3CR),27,28 was conducted on β-keto acid to give an amide derivative. The hydrolysis of the amide derivative is a limiting step.
Recently Isaacson et al.27 modified the procedure using a convertible isonitrile, 1-isocyano-2-(2,2-dimethoxyethyl)benzene (14) in the Ugi reaction, which consents a mild selective cleavage of the resulting C-terminal amide. The unprotected α-methylpyroglutamic acid 17, was thus obtained starting from levulinic acid (13) (Scheme 2).28-30
2.2 3-SUBSTITUTED PYROGLUTAMIC ACID DERIVATIVES SYNTHESES
2.2.1 ASYMMETRIC MICHAEL ADDITION REACTION OF GLYCINE NI(II) COMPLEXES
Ellis et al.31,32 recently reported the first practical synthesis of symmetrically (R,R)-dialkyl-substituted amino acids, including 2-aminoindane-2-carboxylic acid, using complex 20 as a stable, but still reactive glycine equivalent. Belokon’s group described the catalytic asymmetric alkylation of the glycine equivalent 20 under phase-transfer conditions.33 The Ni(II)-complex, was readily prepared on a multigrams scale under inexpensive conditions (Scheme 3).34
First was studied the addition between the complex 21 and oxazolidin-2-one carried out under standard reaction conditions.
The reaction occurred at a substantially high rate and the sole diastereomeric product obtained was decomposed without any purification, to afford the corresponding enantiomerically pure pyroglutamic acid (2S,3R)-25 (Scheme 4).35
Transformation of 25 to ethyl ester by thionyl chloride in ethanol, followed by protection with Boc groups produced the N-Boc-3-arylpyroglutamate 26 in good yields (75-96%).
2.2.2. MICHAEL ADDITION TO UNSATURATED ORTHOPYROGLUTAMATE DERIVATIVES
Oba et al.36 described a chiral synthesis of 3-substitued pyroglutamate derivatives via Michael addition using ABO (2,7,8-trioxabicyclo[3.2.1]octane) as C-terminal protecting group. Treatment of Michael acceptor 27 with Gilman reagent, (prepared from MeLi and CuI), gave derivative 28a in 90% yield (Scheme 5).
Similar reactions with Grignard-cuprates readily produced the derivatives 28b and 28c (71% and 68% respectively). The addition of sodium diethyl malonate afforded the Michael adduct 28d (quantitative). The ABO ester was converted to methyl ester by acid methanolysis followed by reprotection of NH group by Boc group furnishing substituted pyroglutamate derivatives 29a-d in good yields (Scheme 6).
Felluga et al.37 synthesized the nucleus of kainoid amino acids by a chemoenzymatic process, exploiting the diastereomeric cis/trans methyl pyroglutamate derivatives as key intermediates. Compounds 31a-c are accessible by cyclization of intermediates 32a-c obtained from conjugate addition of methyl nitroacetates to the appropriate glutaconic diesters and are ready to enzymatic kinetic resolution (Scheme 7).
The trans pyroglutamic acid derivatives 31a-c were prepared in their racemic form by DBU mediated addition of methyl nitroacetate 34 to the appropriate glutaconic diesters 33a-c. The reaction furnished the corresponding nitrotriesters 35a-c. The nitro group of 35a-c was reduced with Raney nickel following by a spontaneous cyclization to form the lactams 31a-c and 36a-c. Unfortunately, this step is not completely diastereoselective and led to cis/trans mixtures of 31a-c and 36a-c in 9:1 and 4:1 ratio respectively (Scheme 8).
The trans and cis diastereoisomers were unseparable and each mixture was subjected to enzymatic resolution with alpha-chymotrypsin (α-CT). When the hydrolysis reaction was allowed to proceed to 80% conversion, the unreacted diesters (+)-31a-c were isolated with 99% ee in the case of (+)-31a,b and 95% for (+)-31c.
Protection of the amidic nitrogen with Boc group gave compound (+)-39, which after selective and exhaustive lactam carbonyl reduction, afforded the proline derivative (+)-(2S,3R)-40, having a positive optical rotation, in accordance with the data reported for the natural NMDA agonist (Scheme 9).
2.2.3 DIANIONIC ALLYLATION REACTION
A useful synthesis of 3-substituted pyrrolidines and pyrrolidinones, was achieved by the modification of the γ-unsaturated side-chain in allylated derivatives.38,39
Dihydroxylation and oxidative cleavage of the ester 41 gave the pyrrolidinone 43 (60% overall yield) (Scheme 10).
Cyclization of 44 in the presence of Hg(OAc)2 followed by demercuration led to the 5,5-dimethyl- pyrrolidine diester 45 in 70% overall yield.41 An alternative route presents the ozonolytic cleavage of the allyl group in 44, followed by hydrogenation, leading to the des-Cbz analogue of 45.42,43
This synthetic pathway was also used by Artale et al.44 for the synthesis of 4-allylpyroglutamic acid 46 promptly achieveable from glutamic acid (Scheme 11).
Methyl ester 46 was converted into tert-butyl ester 47,45 ozonolysis of 47 and treatment with NaBH4 gave the corresponding alcohol, which was protected as a tert-butyldimethylsilyl ether 48 in 78% yield. The resulting lactam was N-protected affording 49 in 80% yield suitable for peptide synthesis.46
2.3 4-SUBSTITUTED PYROGLUTAMIC ACID DERIVATIVES SYNTHESES
2.3.1 RuO2/NaIO4 OXIDATION
The 4-hydroxypyroglutamic acid is strictly related to 4-OH-proline and it has been widely used as chiral starting material or chiral auxiliary for asymmetric syntheses of γ-substituted glutamic acid (Figure 5).16
Zhang et al.47 reported an efficient route to chiral pure methyl N-Boc-4-hydroxypyroglutamates and their derivatives, starting from the commercially available (4R)-hydroxyproline 37 (Scheme 12).
The key step is a RuO2/NaIO4 oxidation of the opportunely protected 4-hydroxyproline.48,49 This oxidation has been previously reported by Yoshifuji et al.50,51 for the conversion of cyclic α−aminoacids to α-aminodicarboxylic acids. Application of this protocol to 54 and 57, respectively N-Boc-4-silyloxy and 4-acetoxyprotected proline derivatives led to the two diasteromers 55 and 60. At the beginning, (4R)-Hydroxyproline (52) was esterificated and N-Boc protected to give 53 in quantitative yield.52
At this point, in order to obtain the two diasteromeric pyroglutamic acid derivatives, two different pathways have been used. For 4-(R)-trans-N-Boc-4-hydroxylpyroglutamate, OH group of 53 was protected by TBDMS (54). Compound 54 was oxidized by RuO2/NaIO4 in EtOAc/H2O at rt to gave 55. The TBDMS protecting group was removed with TBAF to give methyl (4R)-trans-N-Boc-4-hydroxyl- pyroglutamate (56) in 80% yield. For (4S)-cis-N-Boc-4-hydroxylpyroglutamate 61, a two-step Mitsunobu inversion on C4 was conducted. The protection of 58 as silyl ether 59, oxidation of 59 with RuO2/NaIO4 and deprotection of 60 with TBAF, gave rise to methyl (4S)-cis-N-Boc-4-hydroxyl- pyroglutamate (61) in very good yield.
2.3.2 [1,3]-DIPOLAR CYCLOADDITION
Particularly appealing is the approach based on nitrones reactivity.53
In situ prepared nitrone 65,55 (Scheme 13) was reacted with methyl acrylate 66a in a sealed tube overnight. Two intermediates 67a and 68a (d.r. 2:1), were obtained. A reaction carried out with Oppolzer’s sultam derived acrylamide 66b,56 gave the 67b:67b ratio increased to 20:1 with trans adducts observed preferentially.
The Conversion of intermediates 67a,b, 68a,b to 4-hydroxy-D-pyroglutamic acid derivatives consisted in four sequential step: (i) The sugar was eliminated by acid treatment; (ii) N–O cleavage by hydrogenolysis gave unprotected ethyl 4-hydroxy-D-pyroglutamates 69 and 71; (iii) in situ protection of OH group with TBDMS; (iv) NH protection with Boc group to give the desired compounds 70 (D-pyroglutamate derivative) and 72 (in 1:20 ratio). (Scheme 14)
On the basis of these results, Merino et al.57 reported the first enantioselective synthesis of all the isomers of 4-hydroxypyroglutamic acid, using Oppolzer’s sultam as a chiral auxiliary (Scheme 15).
Nitrone 73 and acrylate 74 were used as starting materials. Reduction and acetylation of 75 gave the intermediate 76. Periodic acid and sodium ipochloride converted the dioxolane ring into the carboxylic functionality (77).57 All of the possible isomers of 4-hydroxypyroglutamic acid can be prepared by this method. Thus, this also served to confirm previous configurational assignments. The orthogonal protection of compound 77 allows its use as building blocks in peptide chemistry.
2.3.3 VIA 4-PROTECTED AMINO-GLUTAMIC ACID DERIVATIVES CYCLIZATION
Recently, Krasnov et al.58 explored the reactivity of γ-protected amino-glutamic acid dimethyl esters (78a,b) (Scheme 16). This study revealed an efficient synthetic approach for producing various substituted pyroglutamate derivatives and, after reduction, of proline derivatives. Derivatives of 4-amino-5-oxophenylproline, such as 4-tosylamino- (82a,b), 4-phthalimido- (79a,b) and 4-Boc-amino-(2S,4S)-5oxophenylprolines (79a,b) were prepared starting from dimethyl (2S,4S)-4-phenylamino- (78a) and (2S,4S)-4-(4-methoxyphenyl)amino-N-phthaloylglutamates 59 (78b), by hydrolysis in different conditions. Treatment with 10N HCl for 1 h at 70 °C resulted in the selective hydrolysis of ester group with the formation of compounds 79a and 79b respectively. Acids 79a and 79b were isolated and crystallized. The hydrolysis of compounds 78a, b in prolonged (12-14 h) refluxing 6N HCl leads to compounds 81a and 81b which posses the free 4-amino group and the protected Nα amino group. Removal of the phtaloyl group of compounds 79a and 79b by hydrazinolysis, under mild condition resulted in compounds 81a and 81b. Synthesis of 4-substituted pyroglutamic acids 84a and 84b was achieved by hydrazinolysis of compounds 78a and 78b and heating in vacuo to have the full conversion into the 4-aminosubstituted pyroglutamates 83a, 83b. Hydrolysis of the ester group by 1N NaOH in acetone yielded pyroglutamic acids 84a and 84b.
2.3.4 THIO-MEDIATED FREE RADICAL CYCLIZATION
Lamberto et al.60 reported the synthesis of three novel alkenyl isocyanides in solid phase and their use in thiol-mediated radical cyclizations. To develop a general synthetic route to isocyanide resins with variation of the alkenyl side chain, the O’Donnell-Solid Phase methods was performed (Scheme 17).61 Glycine coupled Wang resin 86 was reacted with benzophenone imine to activate the resin bound glycine for the next step.62 Deprotonation and alkylation of the benzophenone imine resin 87 with allyl bromide or crotyl bromide was accomplished using iminophosphorane Schweisinger base, BEMP.63
The residues on the resins 88a,b were then hydrolyzed to afford the free amino group and formylated 89a,b.64 The desired alkenyl formyl-amino acids 91a,b were obtained in excellent yields and purity (>90%). Dehydration of the resins 89a,b afforded the desired isocyanides 90a,b. The isocyanides 90a,b were treated with mercaptoethanol and the cyclized products 92a,b cleaved from the solid support to give 93a,b (Scheme 18).
2.3.5 1,4-ADDITION OF ENOLATE TO UNSATURATED ESTERS
Wehbe et al.65 studied the preparation of several substituted glutamate analogues by Diels–Alder, 1,4-ionic and radical reactions starting from (2S)-4-methyleneglutamic acid. The synthesis of the analogues of (S)-Glu applying an intermolecular Heck reaction for soluble polymer (PEG) supported synthesis, was previously described. More recently, was demonstrated that (2S,3S)-3-methyl-glutamic acids act as blocker of the glutamate transport by EAAT2 (Scheme 19).66
The Schiff base 94 was reacted with DBU to give two diastereomers 100 and 96 (75% yield, d.r. 56:44). After separation of the two diasteromers, the cleavage of the chiral auxiliary using citric acid at rt afforded the two amino esters 97 and 101 (82% and 73% yields respectively) which, after hydrolysis provided the corresponding hydrochloride salts (80% yield).
The hydrochloride salts were then treated with propylene oxide in MeOH to give the amino acids 98 and 101 in 90% yield. The cyclic tert-butyl-3-methylpyroglutamates 99 and 103 were obtained quantitatively at rt for 3 h. NMR analysis revealed that the stereochemistry of 99 was cis and for 103 was trans. These results permitted the assignment of (2S,3R) configuration to the major compound 98 and (2S,3S) configuration to the minor compound 102.
Subsequently, Vandenberg et al.67 studied the influence of the chemical group on the C4. The 4-substituted glutamic acid analogues with acid or basic function was chosen.68 These were prepared using an asymmetric 1,4-addition of the enolate obtained from Schiff base, to unsaturated esters (Scheme 20).
For the 4-carboxymethyl derivatives 106 and 107 in situ preparation of using DBU was chosen because it did not give polycondensation products as other chelating bases. At 20 °C in THF, 106 was obtained in 64% yield as a mixture of two inseparable diastereomers.69,70 Acid treatment of 106 with citric acid followed by neutralization with carbonate yielded a diastereomeric mixture of the cyclic product 108 (74% yield) from which 1,3-cis-diastereomer 109 was obtained(35%) after recrystallization. The cyclization could produce a five membered ring 110. The 1,4-addition reaction afforded 107 in 75% yield as a mixture of two diastereomers, which were separated by silica gel chromatography: 107a (35%) and 107b (30%). As shown in Scheme 19, cleavage of the chiral auxiliary from 107a and 107b by citric acid yielded the aminoesters 111a and 111b (85% yield), which were transformed slowly and quantitatively into cyclized products 112a and 112b; after removal of tBu and Boc protecting groups by hydrolysis with 2M HCl, 113a and 113b were obtained (95% yield) (Scheme 21).
3. CONCLUSIONS
In this work we have reviewed the most representative synthetic approaches to the development of pyroglutamate derivatives. Pyroglutamic acid and its derivatives are strictly related to prolines but conversely to the proline-chimeras that have been extensively studied and widely accepted as modification of peptide sequences, pyroglutamate derivatives have been only a marginal role in peptide drug discovery.71
Their asymmetric syntheses have not been properly addressed yet, thus, there is a strong lack of pyroglutamic derivatives related to natural amino acids. A full pyroglutamic acid library derivatives, would expand the possibility to use these structures for drug discovery and certainly their insertion in place of native amino acids into bioactive peptides will achieve novel three dimensional arrangements and consequently peptides with different pharmacological and pharmacokinetic profiles.
References
1. (a) D. K. Chalmers and G. R. Marshall, J. Am. Chem. Soc., 1995, 117, 5927; CrossRef (b) A. Mollica, F. Pinnen, A. Stefanucci, L. Mannina, A. P. Sobolev, G. Lucente, P. Davis, J. Lai, S.-W. Ma, F. Porreca, and V. J. Hruby, J. Med. Chem., 2012, 55, 8477; CrossRef c) M. P. Paradisi, A. Mollica, I. Cacciatore, A. Di Stefano, F. Pinnen, A. M. Caccuri, G. Ricci, S. Duprè, A. Spirito, and G. Lucente, Bioorg. Med. Chem., 2003, 11, 1677; CrossRef (d) A. Mollica, M. P. Paradisi, K. Varani, S. Spisani, and G. Lucente, Bioorg. Med. Chem., 2006, 14, 2253; CrossRef (e) D. Torino, A. Mollica, F. Pinnen, F. Feliciani, S. Spisani, and G. Lucente, Bioorg. Med. Chem., 2009, 17, 251; CrossRef (f) T.-Y. Tsai, T.-K. Yeh, X. Chen, T. Hsu, Y.-C. Jao, C.-H. Huang, J.-S. Song, Y.-C. Huang, C.-H. Chien, J.-H. Chiu, S.-C. Yen, H.-K. Tang, Y.-S. Chao, and W.-T. Jiaang, J. Med. Chem., 2010, 53, 6572; CrossRef (g) P. Garin-Chesa, L. J. Old, and W. J. Rettig, Proc. Natl. Acad. Sci. USA, 1990, 87, 7235. CrossRef
2. T. Itoh, M. Miyazaki, S. Ikeda, K. Nagata, M. Yokoya, Y. Matsuya, Y. Enomoto, and A. Ohsawa, Tetrahedron, 2003, 59, 3527. CrossRef
3. F. Effenberger, W. Muller, R. Keller, W. Wild, and T. Ziegler, J. Org. Chem., 1990, 55, 3064. CrossRef
4. S. K. Panday, J. Prasad, and D. K. Dikshit, Tetrahedron: Asymmetry, 2009, 20, 1581. CrossRef
5. J. Dyer, S. Keeling, and M. G. Moloney, Chem. Commun., 1998, 4, 461. CrossRef
6. L. Chen, J. W. Tilley, R. W. Guthrie, F. Mennona, T. Huang, G. Kaplan, R. Trilles, D. Miklowski, N. Huby, V. Schwinge, B. Wolitzky, and K. Rowan, Bioorg. Med. Chem. Lett., 2000, 10, 729. CrossRef
7. D. W. Konas and J. K. Coward, J. Org. Chem., 2001, 66, 8831. CrossRef
8. S. Conde, P. L. Serrano, and A. Martínez, J. Mol. Cat. B, 1999, 7, 29. CrossRef
9. D. A. Parrish and L. J. Mathias, J. Org. Chem., 2002, 67, 1820. CrossRef
10. D. A. Parrish, L. J. Mathias, and K. M. Moore, Macromolecules, 2003, 36, 4250. CrossRef
11. S. El Ghammarti and B. Rigo, J. Heterocycl. Chem., 1998, 35, 555. CrossRef
12. W. Huang and M. J. Miller, Tetrahedron: Asymmetry, 2008, 19, 2835. CrossRef
13. J. Ezquerra, C. Pedregal, A. Rubio, B. Yruretagoyena, A. Escribano, and S. S. Ferrando, Tetrahedron, 1993, 49, 8665. CrossRef
14. J. Ezquerra, C. Pedregal, A. Rubio, J. J. Vaquero, M. P. Matia, J. Martin, A. Diaz, J. L. G. Navio, and J. B. Deeter, J. Org. Chem., 1994, 59, 4327. CrossRef
15. A. N. Bowler, P. M. Doyle, P. B. Hitchcock, and D. W. Young, Tetrahedron Lett., 1991, 32, 2679. CrossRef
16. J. E. Baldwin, T. Miranda, M. Moloney, and T. Hokelek, Tetrahedron, 1989, 45, 7459. CrossRef
17. D. K. Dikshit and S. K. Panday J. Org. Chem., 1992, 57, 1920. CrossRef
18. T. Ohta, A. Hosoi, and S. Nozoe, Tetrahedron Lett., 1988, 29, 329. CrossRef
19. A. G. Avent, A. N. Bowler, P. M. Doyle, C. M. Marchand, and D. W. Young, Tetrahedron Lett., 1992, 33, 1509. CrossRef
20. P. Merino, S. Anoro, S. Franco, F. L. Merchan, T. Tejero, and V. Tunon, J. Org. Chem., 2000, 65, 1590. CrossRef
21. M. Scansetti, X. Hu, B. P. McDermott, and H. W. Lam, Org. Lett., 2007, 9, 2159. CrossRef
22. T. K. Ellis, H. Ueki, T. Yamada, Y. Ohfune, and V. A. Soloshonok, J. Org. Chem., 2006, 71, 8572. CrossRef
23. C. Nájera and M. Yus, Tetrahedron: Asymmetry, 1999, 10, 2245. CrossRef
24. J. C. Simpson, C. Ho, E.F. B. Shands, M. C. Gershengorn, G. R. Marshall, and K. D. Moeller, Bioorg. Med. Chem., 2002, 10, 291. CrossRef
25. W. Li and K. D. Moeller, J. Am. Chem. Soc., 1996, 118, 10106. CrossRef
26. T. Shono, Y. Matsumura, and K. Tsubata, In Organic Synthesis ed. by G. Saucy, Organic Synthesis, 1984.
27. J. Isaacson, C. Gilley, and Y. Kobayashi, J. Org. Chem., 2007, 72, 3913. CrossRef
28. (a) K. M. Short and A. M. M. Mjalli, Tetrahedron Lett., 1997, 38, 359; CrossRef (b) G. C. B. Harriman, Tetrahedron Lett., 1997, 38, 5591; CrossRef (c) C. Hanusch-Kompa and I. Ugi, Tetrahedron Lett., 1998, 39, 2725; CrossRef (d) H. Tye and M. Whittaker, Org. Biomol. Chem., 2004, 2, 813.
29. C. B. Gilley, M. J. Buller, and Y. Kobayashi, Org. Lett., 2007, 9, 3631. CrossRef
30. (a) T. Takenishi and O. Simamura, Bull. Chem. Soc. Jpn., 1954, 27, 207; CrossRef (b) K. Pfister, W. J. Leanza, J. O. Conbere, H. J. Becker, A. R. Matzuk, and E. F. Rogers, J. Am. Chem. Soc., 1955, 77, 697. CrossRef
31. (a) T. K. Ellis, V. M. Hochla, and V. A. Soloshonok, J. Org. Chem., 2003, 68, 4973; CrossRef (b) V. A. Soloshonok, C. Cai, and V. J. Hruby, Tetrahedron Lett., 2000, 41, 135. CrossRef
32. T. K. Ellis, C. H. Martin, H. Ueki, and V. A. Soloshonok, Tetrahedron Lett., 2003, 44, 1063. CrossRef
33. Y. N. Belokon, N. B. Bespalova, T. D. Churkina, I. Cisarova, M. G. Ezernitskaya, S. R. Harutyunyan, R. Hrdina, H. B. Kagan, P. Kocovsky, K. A. Kochetkov, O. V. Larionov, K. A. Lyssenko, M. North, M. Polasek, A. S. Peregudov, V. V. Prisyazhnyuk, and S. Vyskocil, J. Am. Chem. Soc., 2003, 125, 12860. CrossRef
34. T. K. Ellis, C. H. Martin, H. Ueki, and V. A. Soloshonok, Eur. J. Org. Chem., 2003, 10, 1954.
35. V. A. Soloshonok, H. Ueki, R. Tiwari, C. Cai, and V. J. Hruby, J. Org. Chem., 2004, 69, 4984. CrossRef
36. M. Oba, T. Saegusa, N. Nishiyama, and K. Nishiyama, Tetrahedron, 2009, 65, 128. CrossRef
37. F. Felluga, C. Forzato, P. Nitti, G. Pitacco, F. Ghelfi and E. Valentin, Chirality, 2012, 24, 112. CrossRef
38. S. Hanessian, and R. Margarita, Tetrahedron Lett., 1998, 39, 5887. CrossRef
39. (a) S. J. Wittenberger, S. A. Boyd, and W. R. Baker, Synlett, 1993, 795; CrossRef (b) K. Narasaka and Y. Ukaji, Bull. Chem. Soc. Jpn., 1988, 61, 571. CrossRef
40. (a) S. Hanessian and R. Schaum, Tetrahedron Lett., 1997, 38, 163; CrossRef (b) A. Mollica, A. Stefanucci, F. Feliciani, D. Torino, I. Cacciatore, F. Pinnen, and G. Lucente Tetrahedron Lett., 2010, 51, 1333. CrossRef
41. G. Cardillo and M. Orena, Tetrahedron, 1990, 46, 3321. CrossRef
42. J. A. Marshall, A. W. Garofalo, and R. C. Sedreni, Synlett, 1992, 643.
43. (a) I. B. Parr, S. K. Boehlein, A. B. Dribben, S. M. Schuster, and N. G. J. Richards, J. Med. Chem., 1996, 39, 2367; CrossRef (b) J. M. Humphrey, R. J. Bridges, J. A. Hart, and A. R. Chamberlin, J. Org. Chem., 1994, 59, 2467. CrossRef
44. E. Artale, G. Banfi, L. Belvisi, L. Colombo, M. Colombo, L. Manzoni, and C. Scolastico, Tetrahedron, 2003, 59, 6241. CrossRef
45. (a) C. Alvarez-Ibarra, A. G. Csaky, M. Maroto and M. L. Quiroga, J. Org. Chem., 1995, 60, 6700; CrossRef (b) N. A. Sasaki, R. Pauly, C. Fontaine, A. Chiaroni, C. Riche, and P. Potier, Tetrahedron Lett., 1994, 35, 241. CrossRef
46. (a) S. Hanessian, G. McNaughton-Smith, H. G. Lombart, and D. W. Lubell, Tetrahedron, 1997, 53, 12789; CrossRef (b) J. Gante, Angew. Chem., Int. Ed. Engl., 1994, 33, 1699. CrossRef
47. X. Zhang, A. C. Schmitt, and W. Jiang, Tetrahedron Lett., 2001, 42, 5335. CrossRef
48. M. Kaname and S. Yoshifuji, Tetrahedron Lett., 1992, 33, 8103. CrossRef
49. K. Tanaka and H. Sawanishi, Tetrahedron: Asymmetry, 1998, 9, 71. CrossRef
50. S. Yoshifuji, K. Tanaka, T. Kawai, and Y. Nitta, Chem. Pharm. Bull., 1985, 33, 5515. CrossRef
51. S. Yoshifuji and M. Kaname, Chem. Pham. Bull., 1995, 43, 1617. CrossRef
52. T. Rosen, D. T. W. Chu, I. M. Lico, P. B. Fernades, K. Marsh, L. Shen, V. G. Cepa, and A. G. Pernet, J. Med. Chem., 1988, 31, 1598. CrossRef
53. P. Merino, J. Revuelta, T. Tejero, U. Chiacchio, A. Rescifina A. Piperno, and G. Romeo, Tetrahedron: Asymmetry, 2002, 13, 167. CrossRef
54. (a) M. Shimazaki, F. Okazaki, F. Nakashima, T. Ishikawa, and A. Ohta, Heterocycles, 1993, 36, 1823; CrossRef (b) J. D. White, R. A. Badger, H. J. Kezar, A. J. Pallenberg, and G. A. Schieser, Tetrahedron, 1989, 45, 6631; CrossRef (c) M. Burdisso, A. Gamba, R. Gandolfi, and R. Oberti, Tetrahedron, 1988, 44, 3735; CrossRef (d) P. Herczegh, I. Kovacs, L. Szilagyi, T. Varga, Z. Dinya, and F. Sztaricskai, Tetrahedron Lett., 1993, 34, 1211; CrossRef (e) E. Coutouli-Argyropoulou, E. Malamidou-Xenikaki, X. N. Stampelos, and I. N. Alexopoulou, Tetrahedron, 1997, 53, 707. CrossRef
55. P. Merino, J. Revuelta, T. Tejero, U. Chiacchio, A. Rescifina, A. Piperno, and G. Romeo, Tetrahedron: Asymmetry, 2002, 13, 173. CrossRef
56. P. Merino, E. Castillo, S. Franco, F. L. Merchan, and T. Tejero, Tetrahedron, 1998, 54, 12301. CrossRef
57. P. Merino, E. Castillo, S. Franco, F. L. Merchan, and T. Tejero, J. Org. Chem., 1998, 63, 2371. CrossRef
58. V. P. Krasnov, I. A. Nizova, A. Y. Vigorov, T. V. Matveeva, G. L. Levit, P. A. Slepukhin, M. A. Ezhikova, and M. I. Kodess, Eur. J. Org. Chem. 2008, 1802-1810. CrossRef
59. I. A. Nizova, V. P. Krasnov, O. V. Korotovskikh, and L. V. Alekseeva, Izv. Akad. Nauk SSSR, Ser. Khim. 1989, 2781–2785.
60. M. Lamberto, D. F. Corbett, and J. D. Kilburn Tetrahedron Lett., 2004, 45, 8541. CrossRef
61. M. J. O’Donnell, C. Zhou, and W. L. Scott, J. Am. Chem. Soc., 1996, 118, 6070. CrossRef
62. M. J. O’Donnell and R. L. Polt, J. Org. Chem., 1982, 47, 2663. CrossRef
63. (a) R. Schweisinger, J. Willaredt, H. Schlemper, M. Keller, D. L. Schmidt, and H. Fritz, Chem. Ber., 1994, 127, 2435; CrossRef (b) D. L. Griffith, M. J. O’Donnell, R. S. Pottorf, W. L. Scott, and J. A. Porco, Tetrahedron Lett., 1997, 38, 8821. CrossRef
64. (a) J. C. Sheehan and D. -D. H. Yang, J. Am. Chem. Soc., 1958, 80, 1154; CrossRef (b) L. I. Krimen, Org. Synth., 1988, 50, 8.
65. J. Wehbe, V. Rolland, M. -L. Roumestant, and J. Martinez, Tetrahedron: Asymmetry, 2003, 14, 1123. CrossRef
66. J. Wehbe, V. Rolland, A. Fruchier, M. -L. Roumestant, and J. Martinez, Tetrahedron: Asymmetry, 2004, 15, 851. CrossRef
67. R. J. Vandenberg, A. D. Mitrovic, M. Chebib, V. J. Balcar, and G. A. R. Johnston, Mol. Pharmacol., 1997, 51, 809.
68. Z.-Q. Gu, D. P. Hesson, J. C. Pelletier, M.-L. Maccecchini, L.-M. Zhou, P. Skolnick, J. Med. Chem., 1995, 38, 2158. CrossRef
69. A. El Achqar, M. Boumzebra, M. L. Roumestant, and P. Viallefont, Tetrahedron, 1988, 44, 5319. CrossRef
70. T. Oguri, N. Kawai, T. Shioiri, and S. Yamada, Chem. Pharm. Bull., 1978, 26, 803.
71. (a) S. K. Panday, M. Dikshit, and D. K. Dikshit, Med. chem. Res., 2009, 18, 566-578; CrossRef (b) R. J. Nachman, K. Kaczmarek, H. J. Williams, G. M. Coast, and J. Zabrocki, Biopol., 2004, 75, 412-419; CrossRef (c) A. Y. Vigorov, I. A. Nizova, K. E. Shalunova, A. N. Grishakov, L. S. Sadretdinova, I. N. Ganebnykh, M. A. Ezhikova, M. I. Kodess, and V. P. Krasnov, Russ. Chem. Bull., Int. Ed., 2011, 60, 873.